Effect of live/dead condition, moisture content and particle size on flammability of gorse (Ulex europaeus) measured with a cone calorimeter
Katharine O. Melnik






A
B
C
D
E
F
Abstract
Live fuel comprises a significant portion of the fuel consumed in forest and scrub crown fires. However, its flammability remains poorly understood. Although live fuel differs from dead fuel in moisture content, chemical composition, cellular structure and physiological characteristics, its higher moisture content masks the effect of other characteristics on its flammability.
The aim of the study was to delineate and assess the effects of live/dead condition, moisture content and particle size on flammability of gorse (Ulex europaeus L.).
Live and dead gorse material of three size classes (0–3, 3–6, and 6–10 mm in diameter) at six moisture contents (0, 10, 25, 50, 75 and 100%) was tested in a cone calorimeter to evaluate its flammability using new sample preparation and moisture conditioning techniques.
On average, live fuel ignited 21% slower, reached 11% higher peak heat release rate, and had a 12% shorter burn duration than dead fuel of the same moisture content. These differences were most pronounced in coarser material.
For gorse, fine dead fuels increase the likelihood of ignition, fine live fuels contribute to high burning intensities, and coarser live and dead fuels prolong combustion.
These findings highlight the need to account for flammability differences between live and dead fuels in fire behaviour models beyond those driven by variations in moisture content.
Keywords: burning behaviour, calorimetry, dead fuel, foliage, heat release rate, HRR, live fuel, New Zealand, oxygen consumption, scrub, shrub, surface area, time to ignition, twigs, water content.
Introduction
In recent years, wildfires around the world have increased in frequency, intensity and duration (Jolly et al. 2015), leading to the increased loss of lives and property, and the destruction or disturbance of ecologically important areas; e.g. 2016 Tasmanian wilderness wildfires (Bowman et al. 2021). Alongside changes to the wildfire regime, a growing number of people are living in the wildland–urban interface (Andrew and Dymond 2013) – the interface between the urban setting and the natural landscape including residential areas adjacent to grasslands, shrublands and forests. This trend raises concerns about increased risk of potential damage from wildfires due to the proximity of people and property to large vegetated areas. More wildfires are entering the urban environment from the natural surroundings, such as the 2018 Carr Fire in northern California (Lareau et al. 2018) and the 2017 Port Hills Fire in Christchurch, New Zealand (Pearce 2018). Wildfire mitigation and control rely on the accuracy of fire behaviour predictions, which require an in-depth understanding of vegetative fuels in the wildland-urban interface and their effect on fire behaviour. This is particularly important for highly flammable species such as gorse (Ulex europaeus L.), which is invasive in several parts of the world (Kariyawasam and Ratnayake 2019) and poses a serious problem for wildfire management (Anderson and Anderson 2009, 2010; Wyse et al. 2016). In order to protect the growing wildland–urban interface communities and make them more resilient to future wildfire events, it is vital to enhance our current understanding of vegetation flammability.
Vegetation flammability, the fuel’s capacity to initiate and propagate combustion, consists of four components: (1) ignitability, the ease with which the fuel ignites; (2) combustibility, the rate at which combustion occurs; (3) sustainability, the ability of the fuel to maintain combustion and produce energy (Anderson 1970); and (4) consumability, the proportion of biomass that gets consumed (Martin 1994). There is no procedure defined in a formal standard for testing the flammability of wildland fuels (White and Zipperer 2010; Kauf et al. 2014; Morandini et al. 2019), and previous studies used various instruments and measured a host of different attributes to quantify the four flammability components, making comparisons between studies challenging. Some methods have been repeated across multiple studies with the goal of standardising such experiments to allow broader comparisons. For instance, a procedure for testing fuel beds of forest floor litter developed by Fonda (2001) was used in several studies, facilitating comparisons of litter across numerous species and experimental conditions (Engber and Varner 2012; Kreye et al. 2020; Kane et al. 2021; Varner et al. 2021, 2022). Bench-scale apparatuses for evaluating the flammability of a wide range of wildland fuel types include the fire propagation apparatus (e.g. Bartoli et al. 2011), the mass loss calorimeter (e.g. Madrigal et al. 2012), the cone calorimeter (e.g. Barboni et al. 2017), and the modified cone calorimeter for in-fire testing (Melnik et al. 2022). The cone calorimeter (Huggett 1980; Janssens 1991) is widely used in fire engineering, and is an effective instrument for assessing vegetation flammability because it reliably measures the heat release rate, the main attribute describing the combustibility of fuel (Babrauskas and Peacock 1992; Madrigal et al. 2012), in addition to the other three components of flammability. While there is a standard testing procedure suitable for testing building materials in the cone calorimeter (ASTM E1354, ISO 5660), some adaptations need to be made for testing wildland fuels due to their highly variable shape, size, and moisture content. Different studies have used the cone calorimeter to evaluate the flammability of ground surface fuels (e.g. Blank et al. 2006), live or dead foliage (e.g. Weise et al. 2005), live twigs with attached foliage (e.g. Dibble et al. 2007), as well as branches separated into foliage and twigs of various sizes (e.g. Barboni et al. 2017), with the fresh weight, dry weight, or visually estimated projected surface area kept consistent across the experimental samples.
Live fuel comprises a significant portion of the fuel consumed in forest and scrub crown fires during the passage of the fire front (e.g. Melnik 2016). Despite its major role in dictating wildfire behaviour, the flammability of live fuel is not yet well understood (McAllister et al. 2012), and live fuel is often treated as wet dead fuel in fire behaviour prediction models (Fons 1946; Fosberg and Schroeder 1971; Rothermel 1972; Grishin and Perminov 1998; Porterie et al. 2003). While live vegetation sampled at field conditions indeed contains more moisture than dead vegetation, and early studies attribute the flammability differences between live and dead material to differences in moisture content alone (Pickard and Wraight 1961), recent works have shown that moisture content cannot explain the difference in flammability between the live and dead fuel types on its own, and that other factors such as chemical compositions and cellular structures of live fuels also play an important role (Jolly et al. 2012; McAllister et al. 2012; Jervis and Rein 2016; Ramadhan et al. 2021).
In order to decouple the effect of live and dead condition from the influence of moisture content, flammability differences between live and dead fuels need to be evaluated at similar moisture contents. No study has attempted this yet, although several studies have manipulated the moisture content of live and/or dead fuels and tested their flammability. Pickett et al. (2010) humidified excelsior (wood shavings) to make its moisture content closer to that of live fuel, although its moisture content was still considerably lower than that of live fuels, and its physical and chemical characteristics differed. Another study dried live and dead leaves of Eucalyptus saligna to a wide range of moisture contents (Ramadhan et al. 2021) and demonstrated that moisture content affects time to ignition differently in live and dead fuels. No direct comparison between live and dead fuels was carried out due to drastically different moisture content ranges of live (4–120%) and dead (3–14%) fuels during the experiments. Reszka et al. (2020) rehydrated dead pine needles using a climate chamber to a moisture content of 18%, and compared their ignitibility to that of live needles with 60–131% moisture content. McAllister et al. (2012) point out the lack of data within the moisture content range of 0–65% for field-collected live fuel specimens, which hinders the evaluation of the relationship between time to ignition and moisture content in its lower range. This information can be critically important for crown fire behaviour modelling in the extreme situations of severe physiological drought and the dying live plants. These limitations underscore the need for a method that can directly manipulate the moisture content of fuel samples, allowing for evaluation of flammability at a larger range of moisture contents and for separation of the effect of moisture content from vegetation characteristics such as live/dead condition and particle size.
The present study aims to experimentally examine the relative effects of live/dead condition, moisture content and particle size on the flammability of gorse (Ulex europaeus) by isolating the effect of moisture content from other vegetation characteristics. To do so, this work introduces novel sample preparation and moisture conditioning techniques aiming to increase testing consistency between samples.
Materials and methods
The flammability of live and dead gorse (Ulex europaeus L.) needles, twigs and stems was investigated at six moisture contents using a cone calorimeter. Gorse was collected from the banks of the Waimakariri river north of West Melton, New Zealand (43.461°S, 172.353°E) during the southern hemisphere summer between January and March 2023. The predominant vegetation at the sampling location was gorse, with plant heights ranging from 0.4 to 2 m. Gorse material up to 10 mm in size (diameter) was separated into needles, twigs and stems using a go/no-go gauge (Brown 1974; McRae et al. 1979), with material up to 3 mm in size classified as needles, material 3.1–6 mm classified as twigs, and material 6.1–10 mm classified as stems. The groups were further separated into live and dead condition based on the colour and general appearance of the material at the time of collection. The moisture content at the time of collection was on average 140% for live needles, 100% for live twigs and stems, and 12% for dead needles, twigs and stems. The collected plant material was trimmed to 9.5 cm lengths to fit into the 10 cm × 10 cm sample holder of the cone calorimeter.
The experimental design consisted of three factors of two conditions (live and dead), three particle sizes (0–3, 3.1–6 and 6.1–10 mm), and six moisture contents (0, 10, 25, 50, 75 and 100%), with N = 3 replicates for each combination (Fig. 1a). This was a full factorial design, meaning that all possible combinations of categorically sampled fuel condition, moisture content and particle size levels were tested, and the effect of all three factors and their interactions was evaluated. In the case of the 0% moisture content samples, the 0% moisture content represents the moisture content of the samples that have been completely dried according to the oven-drying methodology used (i.e. after 48 h at 60°C), with a small amount of moisture likely still retained within the plant cell structures.'
Experimental design and moisture content conditioning. (a) Five samples of live and dead needles, twigs and stems of equal projected surface area were created for each of the six prescribed moisture content combinations, with three samples per treatment combination tested in the cone calorimeter. (b) A diagram of the procedure used to condition samples to the prescribed moisture content level, where M0 is the moisture content of sample 'MC1' (g/g), assumed to be equal to the initial moisture content of the remaining four samples, wwet1 is the target wet weight of a sample, wwet0 is the initial wet weight of a sample (g), M1 is the target moisture content assigned to the sample, and M1(actual) is the moisture content measured for sample 'MC2', used for checking that the samples tested in the cone calorimeter had moisture content close to what was prescribed.
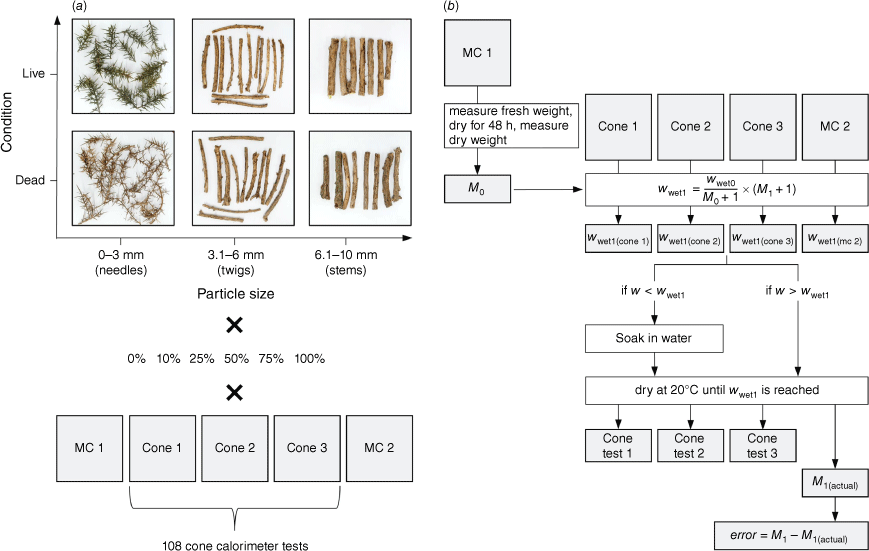
Moisture content conditioning
A batch of five samples was prepared for each combination of particle size, condition and moisture content. One sample (sample ‘MC1’) was used to determine the initial moisture content of all five samples. After this, the four remaining samples were conditioned to one of the six prescribed moisture contents, and three of these samples were subsequently tested in the cone calorimeter, while the last sample of each batch (sample ‘MC2’) was used for measuring the moisture content at testing for verification purposes (Fig. 1a, b). The wet weight (wwet) of the ‘MC1’ sample was recorded, then the sample was dried in the oven at 60°C for 48 h to obtain its dry weight (wdry). Wet weight of a sample is defined as the combined weight of the dry matter and water contained within the sample, while dry weight is defined as the weight of the dry matter only. The decision to use a lower oven-drying temperature of 60°C instead of the higher temperatures (100–105°C) often recommended for fuel moisture samples (Countryman and Dean 1979; Matthews 2010) was made to minimise the loss of volatile organic compounds which can occur at higher temperatures (Englund and Nussbaum 2000), especially by live fuels, altering the mass of the sample. This was offset by using a longer drying time of 48 h (compared with the more common 24 h). Many other studies have also used lower temperatures (60–80°C) and longer drying times (Viegas et al. 1992; Kreye et al. 2020; Varner et al. 2022). The moisture content of the ‘MC1’ sample was assumed to be equal to the initial moisture content of all the samples in the batch, and was calculated using the following equation:
where M0 is the initial moisture content of the batch (g/g), wwet0 is the initial wet weight of the sample (g), and wdry is the dry weight (g) of the sample. Eqn 2 was rearranged to calculate the dry weight of the remaining samples:
The initial moisture content M0 and dry weight wdry of the samples were used to calculate the weight that each sample needed to be conditioned to (dried or wetted) in order to reach the desired moisture content (0, 10, 25, 50, 75 or 100%):
where M1 is the target moisture content (g/g), and wwet1 is the target wet weight (g). After the initial moisture content of the batch was obtained and the target wet weight of each sample was calculated, the samples with the initial wet weight lower than the target were rehydrated by soaking them in water at 3°C. Live samples either did not require rehydration or needed to be rehydrated for less than an hour due to their high initial moisture content, while dead samples were rehydrated for several hours up to 5 days to reach the required moisture content levels. No decomposition or any visual changes to the samples was observed during this process. Finally, the target wet weight for each sample was achieved by air-drying the samples at 20°C and 30–50% relative humidity with their weights checked frequently. Air-drying lasted for several hours for finer fuels and high target moisture contents. For coarse fuels and lower target moisture content the fuels dried for up to 7 days. A slight colour change from vibrant green to medium green occurred in live needles for low target moisture contents, with no visible changes observed in other sample types. As soon as the desired target weight was reached, each sample was placed into a resealable plastic bag inside an airtight plastic container and stored at 3°C until it was tested in the cone calorimeter. The pre-test moisture content of each treatment combination was confirmed by measuring the moisture content of the last remaining sample of each batch (sample ‘MC2’) immediately after testing the three samples of the batch in the cone calorimeter. The difference between the moisture content prescribed to each treatment combination and the actual moisture content measured for sample 'MC2'immediately after cone testing was on average 3%.
Volume measurements
The volume of the samples was measured by immersing them in water and by using Eqn 4 following Melnik (2016):
where Vs is the volume of the sample (mL), wcw is the weight of the container fully filled with water and containing no air (g), wcws is the weight of the container fully filled with water and sample and containing no air (g), ws is the initial weight of the sample (g), and ρw is the density of water (1 g/mL). A glass bottle with a convex lid was used as the measuring container, as the convex lid pushed out all air and the excess water. The volume of the samples is provided in Table 1.
Size/condition | Initial wet weight (g) | Initial dry weight, estimated (g) | Volume (mL) | Density based on wet weight (g/mL) | Density based on dry weight (g/mL) | |
---|---|---|---|---|---|---|
Live needles | 5.55 ± 1.76 | 3.80 ± 0.41 | 9.40 ± 1.13 | 0.58 ± 0.15 | 0.41 ± 0.05 | |
Dead needles | 5.84 ± 1.21 | 4.14 ± 0.50 | 8.64 ± 1.21 | 0.69 ± 0.18 | 0.48 ± 0.04 | |
Live twigs | 21.38 ± 6.53 | 14.80 ± 1.20 | 25.66 ± 1.68 | 0.83 ± 0.22 | 0.58 ± 0.02 | |
Dead twigs | 20.11 ± 5.65 | 13.98 ± 0.90 | 24.26 ± 1.24 | 0.85 ± 0.22 | 0.58 ± 0.02 | |
Live stems | 36.46 ± 10.85 | 25.28 ± 2.34 | 42.31 ± 2.72 | 0.86 ± 0.23 | 0.60 ± 0.03 | |
Dead stems | 35.27 ± 7.91 | 24.81 ± 1.89 | 41.23 ± 2.24 | 0.86 ± 0.19 | 0.60 ± 0.06 |
The values provided are the mean and the standard deviation for each size/condition combination, summarised across all moisture content levels. Initial dry weight was estimated from the initial wet weight of the sample tested in the cone calorimeter and the moisture content of the ‘MC2’ sample.
Projected surface area and sample characterisation
The projected surface area of a sample was defined as the 2D area of the 3D sample projected onto the horizontal plane. This area was kept consistent between samples to ensure that they were exposed to the same amount of radiative heat flux from the conical heater during tests in the cone calorimeter. A sample was placed inside a lightbox onto a white background with four pink markers of known size and spacing printed on the background, and a Logitech C920 camera with 1080p image resolution was mounted 25 cm above the sample (Fig. 2a). The lightbox ensured consistent lighting, the overhead position of the camera minimised distortion due to perspective, the pink markers allowed straightening and scaling of the image, and the white colour of the background created contrast between the background and the sample.
Setup for measuring the projected surface area of the samples. (a) A webcam was mounted on top of a lightbox, and plant material was placed inside the lightbox over the white background with four pink markers used for straightening the image. (b) A screenshot of a sample analysed using the custom python script that measures the projected area in real time, with the detected sample particles outlined in blue. (c) Diagram of the image processing workflow.
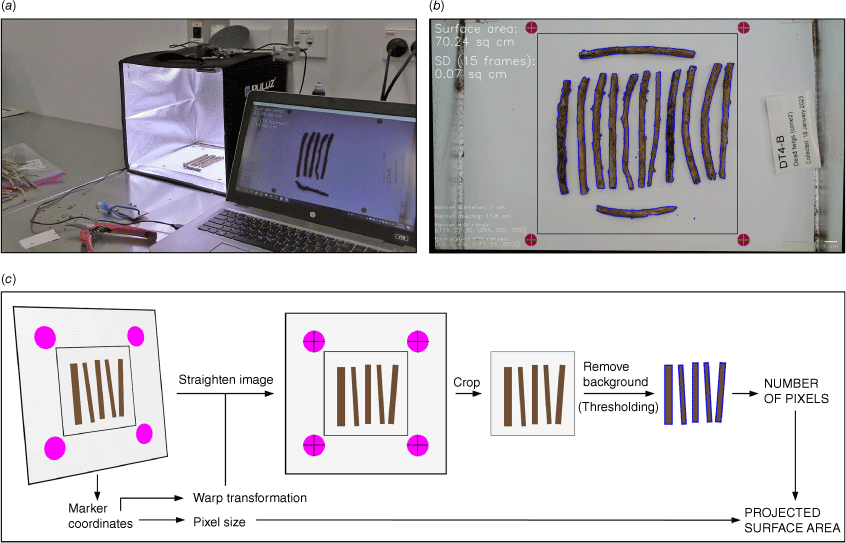
Projected surface area was measured by analysing each frame of the incoming video feed in real time (Fig. 2b, c). First, a colour threshold based on the HSV (hue, saturation, value) colour space was applied to the video feed using the opencv library (Bradski 2000) to determine the coordinates of the centre-points of the four pink markers. The marker coordinates were used to warp the image in order to remove perspective and straighten the image. Additionally, the known distance between the printed markers allowed calculation of the height and width of the pixels in the straightened image. Second, the image was cropped to only retain the area outlined by the markers to facilitate background removal. The hue, saturation and value combination that represented most of the background pixels and excluded most of the sample pixels was manually obtained by using trackbars, and the number of pixels not occupied by the background (i.e. occupied by the sample) was counted. Finally, the number of pixels occupied by the sample was multiplied by the area of each pixel to obtain the projected surface area. The projected surface area of the current frame and the standard deviation of the last 15 frames was displayed on the screen (Fig. 2b). Material was added to the sample until the projected surface area reading was between 69 and 71 cm2, and the standard deviation from the last 15 frames was less than 1 cm2. Because the variable kept consistent between all samples was the projected surface area, the initial wet weight, initial estimated dry weight, and volume differed between samples and are presented in Table 1.
Cone calorimetry
A total of 36 treatment combinations were evaluated, with 108 samples tested in the cone calorimeter. The samples were tested in accordance with the ISO 5660-1 standard (ISO 5660-1 2002), with the exception that porous 10 cm × 10 cm stainless-steel wire mesh sample holders (similar to Barboni et al. 2017) were used. Three different sample holders were used to keep equal distance from the cone heater to the exposed surface of needles, twigs and stems. The sample holders were elevated above an insulation plate and placed onto a heavy-duty stainless steel sample holder used for standard cone colorimeter tests (Fig. 3). The height of the insulation varied to ensure that the tops of the wire baskets were at the same height, even though the baskets themselves were of different heights: 20 mm for needles; 6 mm for twigs; and 10 mm for stems. The heat flux of 50 kW/m2 used for radiant heating of the samples in the cone calorimeter tests was similar to the radiative heat flux at the vegetation surface during fire front arrival in a shrub fire (Morandini and Silvani 2010). The surface area of the sample exposed to the cone heater element was kept at 70 cm2 for samples of all treatment combinations to ensure consistent heating and ignition conditions. Dry mass was found to be relatively consistent across live and dead samples of the same size class. For more details, see Tables 1 and Appendix 1.
Ignitability was quantified as time to ignition (s), combustibility was quantified as peak heat release rate (kW/m2) and mean heat release rate (average heat release rate during flaming combustion, kW/m2), and sustainability was quantified as burn duration (duration of flaming combustion, s) and flaming heat release (heat released during flaming combustion, kJ). Consumability was not investigated due to technical limitations.
Analysis
Data preparation and analyses were performed in R software (R Core Team 2023). The effects of condition, size and moisture content on flammability attributes were evaluated using ANOVA and Pearson correlation tests. A three-way ANOVA (N = 3) was performed for each flammability attribute to examine if it was significantly affected by live/dead condition, particle size and moisture content, and also to measure the level of interaction between these three factors. A relationship was considered significant if the P-value was below 0.05. The correlation between moisture content and each flammability attribute was examined by performing a Pearson correlation test (N = 18) for each size/condition combination, resulting in six correlation tests for each flammability attribute. In addition, the correlation between size and each flammability attribute was examined by performing a Pearson correlation test (N = 18) for each moisture content, resulting in the additional six correlation tests for each flammability attribute. A correlation was considered significant if the P-value was below 0.05. A correlation was considered to be weak when r (Pearson correlation coefficient) was below 0.5, moderate with r between 0.5 and 0.7, and strong if r was above 0.7. Finally, the relative differences between live and dead fuel (live-dead deltas) for all the flammability attributes were compared between needles, twigs and stems across six moisture contents by subtracting flammability values of dead fuel from those of live fuel. The live-dead deltas were compared between needles, twigs and stems using a one-way ANOVA and Tukey’s test for pair-wise comparisons (N = 6). A one-sample t-test was performed to determine whether the live-dead delta for each particle size was significantly different from zero.
Results
The heat release rate (HRR) curves for live and dead needles, twigs and stems at six moisture contents measured with the cone calorimeter suggest that the size, condition and moisture content of gorse material all influence its flammability (Fig. 4). Visual inspection of the curves revealed that plant material of smaller size ignited faster and with greater intensity compared to the larger size classes, while large particle sizes were associated with longer burning and decay stages of combustion, such as smouldering and residual burning. Additionally, live and dead needles largely produced unimodal (i.e. single peak) HRR curves, while the HRR curves of twigs and stems transitioned from unimodal to bimodal (i.e. dual peak) as moisture content increased. In order to further investigate and analyse the influence of moisture content, particle size and live/dead condition on gorse flammability in detail, the HRR data were summarised and expressed in terms of ignitability (time to ignition), combustibility (peak HRR and mean HRR during flaming combustion) and sustainability (burn duration and flaming heat release). The results of the statistical analyses are presented below, with an in-depth synthesis of the results and a discussion about their significance, contribution and implications provided in the next section. The sample characteristics and flammability attributes for each treatment combination are shown in Table A1.
Heat release rate for live and dead gorse needles (a and b), twigs (c and d) and stems (e and f) as a function of time since the start of exposure to the radiative heat flux of 50 kW/m2, coloured by moisture content. The difference in time ranges on the x-axes are due to the longer burn durations of the larger particle sizes.
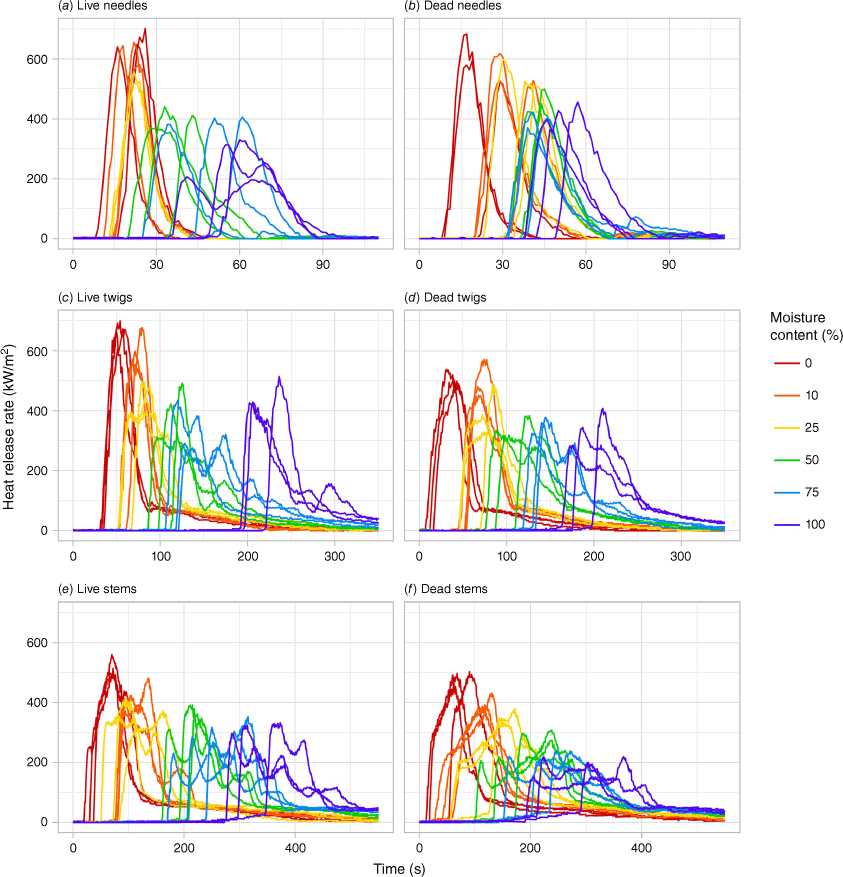
Ignitability
Ignitability was quantified as time to ignition, and ranged from 8 s to 347 s across all tests. Time to ignition was strongly influenced by all three examined variables – condition, size and moisture content (Table 2), with larger particle size exhibiting stronger effects of live/dead condition and moisture content. Fig. 5a shows time to ignition as a function of moisture content, with line colour indicating particle size, the solid line representing live fuel, and dashed line representing dead fuel. The differences between live and dead fuels (live-dead deltas) were obtained by subtracting times to ignition of dead fuels from times to ignition of live fuels, and are shown in Fig. 5b for needles, twigs and stems. The estimated population mean (µ) of the live-dead deltas was determined to either be significantly different from zero (µ ≠ 0) or comparable to zero (µ ≈ 0). The live-dead deltas were also compared between needles, twigs and stems, with deltas that significantly differed between groups marked with an asterisk. Time to ignition did not significantly differ for live and dead needles, while live twigs took on average 9% longer to ignite than dead twigs, and live stems took on average 24% longer to ignite than dead stems.
Variable | Size | Condition | MC | Size * condition | Size * MC | Condition * MC | Size * condition * MC | |
---|---|---|---|---|---|---|---|---|
Time to ignition (s) | <0.001 | 0.01 | <0.001 | 0.002 | <0.001 | 0.129 | 0.236 | |
Peak HRR (kW/m2) | <0.001 | <0.001 | <0.001 | 0.023 | 0.115 | 0.085 | 0.002 | |
Mean HRR (kW/m2) | 0.045 | <0.001 | <0.001 | 0.333 | 0.022 | 0.254 | 0.078 | |
Burn duration (s) | <0.001 | 0.014 | <0.001 | 0.183 | <0.001 | 0.711 | 0.297 | |
Flaming heat release (kJ) | <0.001 | 0.898 | 0.267 | 0.617 | 0.023 | 0.011 | 0.206 |
Effect of size, condition and moisture content on ignitability quantified as time to ignition. (a) Time to ignition as a function of moisture content coloured by particle size, with solid lines representing mean values for live fuel and dashed lines representing mean values for dead fuel. (b) Box plots of live-dead delta for time to ignition – the difference between live and dead fuel obtained by subtracting time to ignition of dead fuels from time to ignition of live fuels. The live-dead deltas that significantly differed from zero are marked as ‘µ ≠ 0’, and those comparable to zero are marked with ‘µ ≈ 0’. The live-dead deltas that significantly differed between needles, twigs, and/or stems are marked with an asterisk (*).
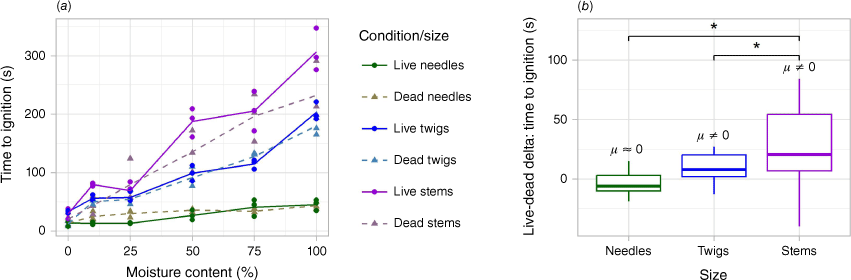
Time to ignition increased with increase in moisture content and particle size. There was a strong positive correlation between time to ignition and moisture content for all size and condition combinations (r = 0.80 to 0.97, P < 0.001). On average, time to ignition was seven times higher for samples with 100% moisture content as opposed to oven-dry (0% moisture content) samples. There was also a strong positive correlation between time to ignition and particle size for all moisture contents above 0% (r = 0.76 to 0.96, P < 0.001), with no correlation detected at 0% moisture content.
Combustibility
Combustibility was quantified as peak heat release rate (peak HRR) and mean heat release rate (mean HRR), and ranged from 199 to 701 kW/m2, and from 108 to 479 kW/m2, respectively. Combustibility was significantly affected by condition, moisture content and particle size (Table 2). Combustibility generally decreased with an increase in moisture content and particle size (Fig. 6a, b), and was on average higher for live than for dead material (Fig. 6c, d). The only exception to this general trend was that the peak heat release rate did not significantly differ between live and dead needles, whereas it was 21% higher for live than dead twigs and 19% higher for live than dead stems. Correspondingly, mean heat release rate was 23% higher for live than dead needles, 13% higher for live than dead twigs, and 13% higher for live than dead stems.
Effect of size, condition and moisture content on combustibility quantified as peak HRR and mean HRR. Peak HRR (a) and mean HRR (b) as a function of moisture content, coloured by particle size, with solid lines representing mean values for live fuel and dashed lines representing mean values for dead fuel. Live-dead delta (differences between live fuel and dead fuel) for peak HRR (c) and mean HRR (d), with ‘µ ≠ 0’ marking live-dead deltas that are significantly different from zero, and asterisks (*) denoting significant difference in live-dead deltas between needles, twigs and stems.
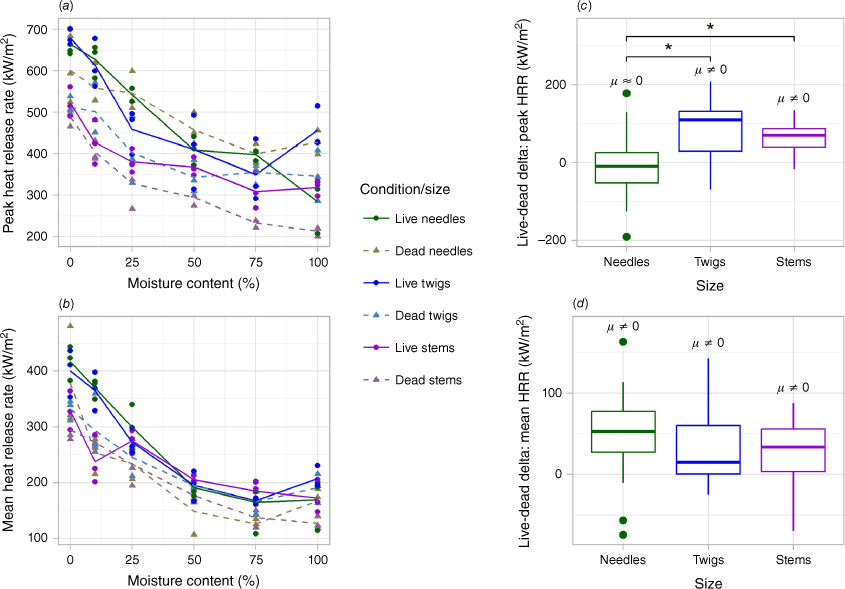
There was a moderate to strong negative correlation between moisture content and peak HRR for all size/condition combinations (r = –0.70 to –0.95, P ≤ 0.001), and a strong negative correlation between moisture content and mean HRR for all size/condition combinations (r = –0.75 to –0.94, P ≤ 0.001). On average, peak HRR was 40% lower and mean HRR was 52% lower for samples with 100% moisture content when compared to oven-dry samples. Peak HRR was also moderately to strongly negatively correlated with particle size for moisture contents from 0 to 75% (r = –0.64 to –0.83, P from <0.001 to 0.004), but not significantly affected by size for moisture content of 100%. Particle size moderately negatively correlated with mean heat release rate for 0% moisture content (r = –0.61, P = 0.007), but there was no correlation detected for the other moisture contents.
Sustainability
Sustainability was quantified as burn duration and flaming heat release, and ranged from 11 to 241 s and 46 to 378 kJ, respectively. Both sustainability metrics were affected by size, with burn duration also affected by condition and moisture content (Table 2). Burn duration and flaming heat release increased with size (Fig. 7a, b). Moisture content caused an increase in burn duration, but there was no clear trend for its effect on flaming heat release. Condition had an effect on burn duration, with burn duration 24% shorter for live than dead needles, 14% shorter for live than dead stems, and similar for live and dead twigs, while flaming heat release was similar for live and dead fuel (Fig. 7c, d).
Effect of size, condition and moisture content on sustainability quantified as burn duration and flaming heat release. Burn duration (a) and flaming heat release (b) as a function of moisture content, coloured by particle size, with solid lines representing mean values for live fuel and dashed lines representing mean values for dead fuel. Live-dead delta (differences between live fuel and dead fuel) for burn duration (c) and flaming heat released (d), with ‘µ ≠ 0’ marking live-dead deltas that are significantly different from zero, and the absence of asterisks (*) indicating no significant difference in live-dead deltas between needles, twigs and stems.
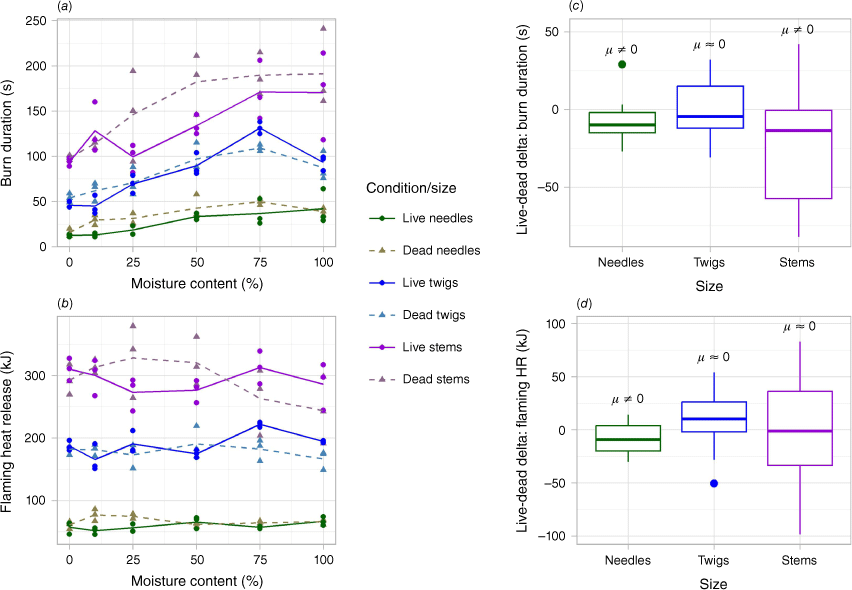
Burn duration was moderately to strongly positively correlated with moisture content for all size/condition combinations (r = 0.70 to 0.80, P < 0.001 to P = 0.002), and was on average two times higher for samples with 100% moisture content than for oven-dry samples. Flaming heat release was positively correlated with moisture content for live twigs (r = 0.48, P = 0.04), negatively correlated with moisture content for dead stems (r = –0.48, P = 0.04), and was not correlated with moisture content for the other size/condition combinations. Burn duration was also strongly positively correlated with particle size for all moisture content levels (r = 0.84 to 0.99, P < 0.001). Flaming heat release was positively correlated with particle size for all moisture content levels (r = 0.94 to 0.99, P < 0.001).
Discussion
This study investigated the flammability of gorse, a shrub species invasive in several parts of the world and notorious for its wildfire potential. Each of the three examined vegetation characteristics – the condition of the plant material (live or dead), its moisture content, and its size – along with the complex interactions between them, had a significant impact on gorse flammability and consequently on the likelihood of ignition and intensity of wildfires.
Effect of live and dead fuel
The presented research is the first to examine the effect of live and dead condition on vegetation flammability separately from the fuel’s moisture content. Other works have previously shown that moisture content alone cannot account for flammability differences between live and dead vegetation (Jolly et al. 2012; McAllister et al. 2012; Jervis and Rein 2016; Ramadhan et al. 2021), and the presented study quantifies the differences between live and dead fuel without the influence of moisture content. On average, live fuel took longer to ignite, burned faster and with greater intensity, and released a similar amount of energy during flaming combustion as dead fuel of the same moisture content.
The longer ignition time and greater peak heat release rate observed in live twigs and stems compared with dead twigs and stems may be attributed to the intact cellular structures in live woody tissues that delay the release of water and volatiles. Twigs and stems mostly consist of vascular tissue made up of long cells fortified with lignin and cellulose that transport water and nutrients (Koide et al. 2000), and previous research has shown that in the case of conifer needles, the cell wall of live cells needs to break down for the water and volatiles to be released (McAllister et al. 2012). It is possible that the rigid lignified cell walls in live twigs and stems might allow for cell pressurisation before the water can escape, delaying water release and increasing the rate at which flammable compounds are ejected after the collapse of cell walls. The delayed water release could explain the observed longer ignition times, while the faster ejection of flammable compounds after cell wall collapse may be linked to the greater peak heat release rates in live twigs and stems. In contrast, dead tissues, especially those partially decomposed, facilitate easier lateral water evaporation when heated, which could induce quicker ignition and slower release of flammable compounds, and lead to lower initial rates of pyrolysis and lower peak heat release rates.
Previous research has demonstrated that moisture release mechanisms differ between live and dead fuels (Fletcher et al. 2007). Dead fuels lose water diffusively as the sample is being heated, while live fuels retain a lot of water in their cells until the cell walls fail (Finney et al. 2012), suggesting higher intra-cellar pressures and correspondingly higher boiling point, and thus higher energy required for fuel preheating before evaporation occurs. Supporting this notion, Anand et al. (2017) reported that living cells release bound water around 200°C, double the temperature of the boiling point of water in normal conditions. During some tests with samples of higher moisture content, water was bubbling out of the dead twigs and stems (Fig. 8), but not live twigs and stems, showing the expedited water escape from dead woody material and suggesting a considerably higher water retention by live compared to dead material. The results reported in the current work partially align with results reported in other studies, which highlighted longer ignition times but lower intensities in live fuel (Jervis and Rein 2016; Reszka et al. 2020; Ramadhan et al. 2021), though their results cannot be compared directly due to the live fuels having higher moisture content in these studies. Our findings are also consistent with results reported by Zhao et al. (2014) where intact, undecomposed dead twigs dried out slower and took more time to ignite than partially decomposed dead twigs of the same moisture content.
Water escaping from the cut ends of dead stems prior to ignition in the form of bubbling foam. This effect was observed in dead twigs and stems but did not occur in live twigs and stems.
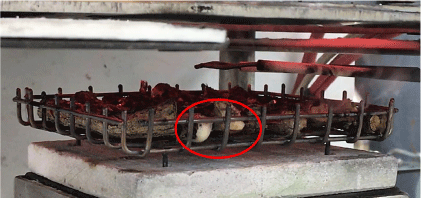
Contrarily to the trend observed in twigs and stems, the ignition times and the peak heat release rates for live and dead needles did not significantly differ, although mean heat release rate was higher for live tissue of all three sizes. The lack of difference in time to ignition and peak heat release rate in live and dead needles could be linked to the high surface-area-to-volume ratio of needles relative to the other size classes, and to needles being thermally thin, which considerably enhances the heating and pyrolysis rate when exposed to a high heat flux. This, combined with the low total energy available for combustion compared to twigs and stems (due to the low mass of the samples necessary to keep a constant projected surface area) likely results in equally rapid surface heating and water evaporation for both live and dead needles, yielding insignificant differences in time to ignition and peak heat release rate. Additionally, the lack of differences in these attributes between live and dead needles could also be related to the distinct cellular composition of needles, which mostly contain mesophyll cells that are not fortified with lignin and cellulose. When subjected to heat, the live mesophyll cells could rupture more easily than the more robust cells of twigs and stems, leading to ignition times comparable to those of dead needles. Leaves with high moisture content have been shown in past research to burst and rapidly release water through ejection (McAllister et al. 2012; Anand et al. 2017; Fazeli et al. 2022), resulting in less energy being used for water evaporation and more energy available for pyrolysis. The water might be released through sudden droplet ejection and subsequent burning, as observed in several experiments (Ahmad et al. 2021; Fazeli et al. 2022). It was proposed that droplet ejection in live fuels could result in shorter ignition times for live than dead fuels (Fazeli et al. 2022), and our findings with needles, albeit statistically insignificant, seem to align with this hypothesis.
It is important to note that live tissue studied here has been separated from the rest of the plant, disrupting the xylem structure and preventing water movement, while in an intact living shrub water can move to the heated region of the branch from other areas (Cohen and Omi 1991). Therefore, water transport throughout xylem structure during combustion in an intact living shrub may affect flammability of live tissue, further contributing to differences between live and dead fuel. Nevertheless, the ability to test live and dead fuels at equivalent moisture content in this study made it possible to evaluate the effect of condition without the confounding effect of moisture content, addressing the challenges faced by previous research (Pickett et al. 2010; Reszka et al. 2020) in matching moisture contents of live and dead fuels. This approach underscores the contribution of our research to the understanding of fuel flammability beyond the lens of moisture content alone, as highlighted in other investigations (Jolly et al. 2012; Ramadhan et al. 2021).
Effect of moisture content
The results of this study suggest that an increase in moisture content significantly decreased ignitability and burning intensity, and increased burning duration, but did not significantly affect the total energy release during flaming. In their work, Etlinger and Beall (2004) showed that moisture content heavily influences plant flammability, as water stored in the plant tissue increases the thermal capacity of the tissue, requiring more energy for ignition and diluting pyrolysis gases once released. The fuel only ignites when the concentration of pyrolysates reaches a critical value close to the ignition pilot (Scarff et al. 2012), and water in plant tissue delays ignition and reduces the pyrolysis rate by using energy for heating and evaporation of water instead of heating the fuel material (Drysdale 2011; Sullivan 2017). The observed strong correlation between time to ignition and moisture content aligns with results of previous experiments (Gill and Moore 1996; Bianchi and Defossé 2015; Reszka et al. 2020; Ramadhan et al. 2021; Fazeli et al. 2022) and with results from numerical simulations (Scarff et al. 2012). McAllister et al. (2012) observed a negative linear relationship between moisture content and flammability for moisture contents above 65%, and the results of the current study suggest that this relationship persists in moisture contents from 0 to 100% as well. Scarff et al. (2021) observed up to three-fold increase in time to ignition in fuel with moisture content ranging from 69 to 250%, compared with a seven-fold increase observed in the current study for moisture content ranging from 0 to 100%.
Moisture content affects fuel combustion beyond ignition, as there is still water present in the sample after ignition occurs (McAllister et al. 2012). Numerical modelling supports the observed negative effect of moisture content on peak and mean heat release rates, as the substantial amount of water remaining in the fuel after ignition results in reduced burning intensity (Yashwanth et al. 2016). Water vapour cools the flame by diluting the pyrolysates and increasing thermal heat capacity (Grant and Drysdale 1997), causing reduction in reaction rate and energy release rate. Interestingly, in our study, moisture content affected the duration and rate of the energy release process (slower heat release over a longer particle flaming time), but did not affect the total amount of energy released during flaming. However, the observed lack of effect on energy release during flaming may not be applicable to wildland fires where the fuel particles are exposed to external heat flux only during the fire front passage, and the longer time to ignition of wetter fuels can result in lower energy release during the passage of a fire front. For instance, a substantial negative effect of moisture content on energy release was observed by Melnik et al. (2022) for live fuel samples tested in an in-flame flammability testing apparatus simulating combustion of fuel in the conditions within the frontal flame for the assumed flame-front residence time of 60 s.
The results of this study indicate that the HRR curves for each size class generally transition from a unimodal shape at lower moisture contents to a bimodal shape at higher moisture contents. Babrauskas (2002) and Khan et al. (2008) both note that ignition times are longer for higher moisture contents, consistent with the findings of this study, but Khan et al. also refer to the delay in ignition time being as a result of increased thermal properties and the effects of moisture evaporation and moisture migration. It is possible that the bimodal shape observed in heat release rate curves at higher moisture contents occurs because the top portion of the sample located closer to the external heat source loses moisture faster and ignites first, while the ignition of the remaining fuel further from the external heat source is delayed due to a delay in moisture evaporation and moisture migration from the top portion of the sample. This phenomenon is more pronounced at higher moisture contents. The subsequent ignition of this lower portion of the fuel leads to the second peak in the HRR curve.
Effect of particle size
Vegetation flammability studies often focus on foliage due to the ease of study, the prevalent consumption of fine particles (Whight and Bradstock 1999; Pearce et al. 2010) and their importance in ignition and combustion (Van Wagner 1983). However, the flammability of larger particles is also relevant since material up to 10 mm in diameter can be consumed during the passage of a fire front (Stocks et al. 2004), and material up to 6 mm in diameter have been shown to dictate fire intensity and rate of spread (Morvan and Dupuy 2004). Particles of different sizes burn differently, with larger particles taking longer to ignite, burning less intensely, sustaining combustion for a longer period and producing more energy overall. Several factors contributed to the observed differences in flammability, with differences in volume and amount of dry material as the main drivers. Because the projected surface area was the same between samples, larger particles with greater weight and volume received less radiative energy per given mass of material and required more energy to heat up the inner portions (Scarff et al. 2012), which could lead to slower ignition times and lower peak heat release rates. The burning duration and the flaming heat release increased with the increase in particle size, which was expected due to the greater mass of material in larger particles.
A comprehensive study on the small shrub Cistus monspeliensis (Montpellier rock rose) demonstrated similar results, where larger particle sizes had longer ignition times and higher total heat release, but lower peak heat release rate (Barboni et al. 2017). A study that measured time to ignition for different species of Utah broadleaf and chaparral detected only a slight influence of leaf thickness on time to ignition (Fletcher et al. 2007). However, the weakness of the correlation could be associated with the narrow leaf thickness range examined and the confounding effects associated with other variables being different between the studied plant species. Notably, the shape of the heat release rate curves in the present study considerably varied with the particle size, with needles typically exhibiting a single peak (unimodal), while twigs and stems displayed two HRR peaks (bimodal), particularly pronounced in samples with high moisture content. Previous research has shown that dead plant material with a diameter over 4 mm develops a second HRR peak, indicating thermally thick charring materials (Barboni et al. 2017).
Limitations and future research
The present study offers valuable insights into the influence of live/dead condition, particle size, and moisture content, as well as their interactions, on gorse flammability. However, the number of replicates tested is a limitation of this study. Despite conducting 108 tests, intra-group variability in the heat release rate curves (Fig. 4) was observed among the three replicates for each of the 36 treatment combinations, particularly with increasing moisture content, appearing to be driven mostly by differences in time to ignition. As can be seen in the Results section, time to ignition varied between 30 s and 43 s for live needles at 50% moisture content in the three repetitions of the test, and from 286 s to 373 s for live stems at 100% moisture content. By summarising the heat release rate data and expressing them in terms of flammability attributes (i.e. time to ignition, peak heat release rate, mean heat release rate, burn duration and flaming heat release), noticeable effects of live/dead condition, particle size and moisture content on all flammability attributes can still be identified. However, it is possible that some interactions between the three factors were present but not detected by the three-way ANOVA due to only having three replicates in each treatment combination. In contrast, the correlation analysis was more robust due to the larger sample size of 18 samples per group for each analysis.
Future research should aim to further explore the mechanisms underlying the observed effects of live and dead fuel characteristics, particle size, and moisture content on flammability in different species. Investigating the chemical composition and cellular structure of different vegetation types can provide insights into the specific factors that contribute to variations in ignitability, combustibility, and sustainability. Additionally, studying the interactions between fuel characteristics and environmental conditions can enhance our understanding of how vegetation flammability is influenced by changing climatic conditions. It would also be important to investigate how the amount of live and dead fuel, particle size distribution and moisture content affect the flammability of whole intact plants, and to examine the relationship between flammability attributes of plant parts measured at the bench scale and those observed when an entire plant is burned in flammability experiments. Additionally, future works could assess the effectiveness of different management strategies in altering fuel properties and their corresponding flammability with the aim to reduce fire risk, informing more targeted and sustainable approaches to wildfire management. Continuing to explore the underlying mechanisms and conducting further investigations into fuel flammability would improve the ability to predict and manage wildfires in a changing environment, ultimately reducing the risk and impact of destructive wildfires.
Conclusions
The presented study provides valuable insights into the flammability of gorse. The study used a novel moisture conditioning technique to experimentally study the relative effects of live/dead condition, moisture content and particle size and their interactions on plant flammability, underscoring the importance of accounting for the differences between live and dead fuels in fire behaviour prediction models. The study was able to directly assess the impact of live and dead condition, moisture content and particle size independently of each other thanks to the developed fuel conditioning technique which allowed for assigning a pre-determined moisture content to each condition and size combination, thereby treating moisture content as one of the experimental factors in a full-factorial design. Furthermore, the methodology used for accurately measuring the projected surface area of samples ensured that the variability of this aspect was minimised, improving on visual estimation used by Barboni et al. (2017) and ensuring that a consistent sample surface area was exposed to the radiative heat from the cone heater. The findings suggest that the fine dead material that accumulates in the gorse canopy increases the likelihood of ignition, the fine live fuels contribute to the high burning intensities observed in this vegetation type, and the coarser live and dead particles prolong combustion. This research contributes to the current understanding of vegetation flammability, and highlights the need to account for flammability differences between live and dead fuels in fire behaviour prediction models resulting from their chemical, physiological and cell structure properties in addition to those driven by variations in moisture content.
Data availability
The data supporting this study will be shared upon reasonable request to the corresponding author.
Declaration of funding
This research was co-funded by Ministry of Business, Innovation and Employment (MBIE), New Zealand (grant number C04X2103 ‘Extreme wildfire, our new reality, are we ready?’), the Royal Society of New Zealand (grant number RDF-UOC1701), and the University of Canterbury College of Engineering.
Acknowledgements
The authors thank Grant Dunlop, Logan Cooper, Milap Dhakal, Jeihan Hapsari and Fearghal Gill for assistance with sample collection and testing, Environment Canterbury (Gill Walsh and Karen Elliott) for providing access to the research site, and two anonymous reviewers for their constructive feedback that helped to improve the quality of this manuscript.
References
Ahmad AD, Abubaker AM, Salaimeh A, Akafuah NK, Finney M, Forthofer JM, Saito K (2021) Ignition and burning mechanisms of live spruce needles. Fuel 304(July), 121371.
| Crossref | Google Scholar |
Anand C, Shotorban B, Mahalingam S, McAllister S, Weise DR (2017) Physics-based modeling of live wildland fuel ignition experiments in the forced ignition and flame spread test apparatus. Combustion Science and Technology 189(9), 1551-1570.
| Crossref | Google Scholar |
Anderson HE (1970) Forest fuel ignitibility. Fire Technology 6(4), 312-319.
| Crossref | Google Scholar |
Anderson SAJ, Anderson WR (2009) Predicting the elevated dead fine fuel moisture content in gorse (Ulex europaeus L.) shrub fuels. Canadian Journal of Forest Research 39(12), 2355-2368.
| Crossref | Google Scholar |
Anderson SAJ, Anderson WR (2010) Ignition and fire spread thresholds in gorse (Ulex europaeus). International Journal of Wildland Fire 19(5), 589-598.
| Crossref | Google Scholar |
Andrew R, Dymond JR (2013) Expansion of lifestyle blocks and urban areas onto high-class land: an update for planning and policy. Journal of the Royal Society of New Zealand 43(3), 128-140.
| Crossref | Google Scholar |
Babrauskas V (2002) Ignition of wood: a review of the state of the art. Journal of Fire Protection Engineering 12(3), 163-189.
| Crossref | Google Scholar |
Babrauskas V, Peacock RD (1992) Heat release rate: the single most important variable in fire hazard. Fire Safety Journal 18, 255-272.
| Crossref | Google Scholar |
Barboni T, Leonelli L, Santoni PA, Tihay-Felicelli V (2017) Influence of particle size on the heat release rate and smoke opacity during the burning of dead Cistus leaves and twigs. Journal of Fire Sciences 35(4), 259-283.
| Crossref | Google Scholar |
Bartoli P, Simeoni A, Biteau H, Torero JL, Santoni PA (2011) Determination of the main parameters influencing forest fuel combustion dynamics. Fire Safety Journal 46(1–2), 27-33.
| Crossref | Google Scholar |
Bianchi LO, Defossé GE (2015) Live fuel moisture content and leaf ignition of forest species in Andean Patagonia, Argentina. International Journal of Wildland Fire 24(3), 340-348.
| Crossref | Google Scholar |
Blank RR, White RH, Ziska LH (2006) Combustion properties of Bromus tectorum L.: influence of ecotype and growth under four CO2 concentrations. International Journal of Wildland Fire 15(2), 227-236.
| Crossref | Google Scholar |
Bowman DMJS, Rodriguez-Cubillo D, Prior LD (2021) The 2016 Tasmanian Wilderness Fires: Fire Regime Shifts and Climate Change in a Gondwanan Biogeographic Refugium. In ‘Ecosystem Collapse and Climate Change’. (Eds JG Canadell, RB Jackson) pp. 133–153. (Springer International Publishing) 10.1007/978-3-030-71330-0_6
Bradski G (2000) The OpenCV Library. Dr. Dobb’s Journal of Software Tools. https://www.drdobbs.com/open-source/the-opencv-library/184404319
Brown JK (1974) Handbook for inventorying downed woody material Issue General Technical Report INT-16. https://www.fs.usda.gov/rm/pubs_int/int_gtr016.pdf
Cohen WB, Omi PN (1991) Water-stress effects on heating-related water transport in woody plants. Canadian Journal of Forest Research 21, 199-206.
| Google Scholar |
Countryman CM, Dean WA (1979) Measuring moisture content in living chaparral: a field user’s manual. USDA For. Serv. Gen. Tech. Rep. PSW-36. p. 28. Available at http://www.fs.fed.us/psw/publications/documents/psw_gtr036/
Dibble AC, White RH, Lebow PK (2007) Combustion characteristics of north-eastern USA vegetation tested in the cone calorimeter: Invasive versus non-invasive plants. International Journal of Wildland Fire 16(4), 426-443.
| Crossref | Google Scholar |
Drysdale DD (2011) Chapter 6 - Ignition: The Initiation of Flaming Combustion. In ‘An Introduction to Fire Dynamics’. 3rd edn. pp. 225–275. (John Wiley & Sons, Ltd.) 10.1002/9781119975465
Engber EA, Varner JM (2012) Patterns of flammability of the California oaks: the role of leaf traits. Canadian Journal of Forest Research 42(11), 1965-1975.
| Crossref | Google Scholar |
Englund F, Nussbaum RM (2000) Monoterpenes in Scots pine and Norway spruce and their emission during kiln drying. Holzforschung 54(5), 449-456.
| Crossref | Google Scholar |
Etlinger MG, Beall FC (2004) Development of a laboratory protocol for fire performance of landscape plants. International Journal of Wildland Fire 13(4), 479-488.
| Crossref | Google Scholar |
Fazeli H, Jolly WM, Blunck DL (2022) Stages and time-scales of ignition and burning of live fuels for different convective heat fluxes. Fuel 324(PA), 124490.
| Crossref | Google Scholar |
Finney MA, Cohen JD, McAllister SS, Jolly WM (2012) On the need for a theory of fire spread. International Journal of Wildland Fire 22, 25-36.
| Crossref | Google Scholar |
Fletcher TH, Pickett BM, Smith SG, Spittle GS, Woodhouse MM, Haake E, Weise DR (2007) Effects of moisture on ignition behavior of moist California Chaparral and Utah leaves. Combustion Science and Technology 179(6), 1183-1203.
| Crossref | Google Scholar |
Fonda RW (2001) Burning characteristics of needles from eight pine species. Forest Science 47(3), 390-396.
| Crossref | Google Scholar |
Fons WL (1946) Analysis of fire spread in light forest fuels. Journal of Agricultural Research 72(13), 93-121.
| Google Scholar |
Gill AM, Moore PHR (1996) Ignitibility of leaves of Australian plants. A Contract Report to the Australiam Flora Foundation, October, 1–34. http://aff.antl.com.au/wp-content/uploads/Gill_ignitibility_final.pdf
Grishin AM, Perminov VA (1998) Mathematical modeling of the ignition of tree crowns. Combustion, Explosion and Shock Waves 34(4), 378-386.
| Crossref | Google Scholar |
Huggett C (1980) Estimation of rate of heat release by means of oxygen consumption measurements. Fire and Materials 4(2), 61-65.
| Crossref | Google Scholar |
ISO 5660-1 (2002) Reaction‐to‐fire tests‐Heat release, smoke production and mass loss rate‐Part 1: heat release rate, cone calorimeter method. https://www.standards.govt.nz/shop/iso-5660-12002
Janssens ML (1991) Measuring rate of heat release by oxygen consumption. Fire Technology 27(3), 234-249.
| Crossref | Google Scholar |
Jervis FX, Rein G (2016) Experimental study on the burning behaviour of Pinus halepensis needles using small-scale fire calorimetry of live, aged and dead samples. Fire and Materials 40, 385-395.
| Crossref | Google Scholar |
Jolly WM, Parsons RA, Hadlow AM, Cohn GM, McAllister SS, Popp JB, Hubbard RM, Negron JF (2012) Relationships between moisture, chemistry, and ignition of Pinus contorta needles during the early stages of mountain pine beetle attack. Forest Ecology and Management 269, 52-59.
| Crossref | Google Scholar |
Jolly WM, Cochrane MA, Freeborn PH, Holden ZA, Brown TJ, Williamson GJ, Bowman DMJS (2015) Climate-induced variations in global wildfire danger from 1979 to 2013. Nature Communications 6(May), 1-11.
| Crossref | Google Scholar |
Kane JM, Kreye JK, Barajas-Ramirez R, Varner JM (2021) Litter trait driven dampening of flammability following deciduous forest community shifts in eastern North America. Forest Ecology and Management 489, 119100.
| Crossref | Google Scholar |
Kariyawasam C, Ratnayake S (2019) Reproductive biology of gorse, Ulex europaeus (Fabaceae) in the Mount Lofty Ranges of South Australia and Sri Lanka. The International Journal of Plant Reproductive Biology 11(2), 145-152.
| Crossref | Google Scholar |
Kauf Z, Fangmeier A, Rosavec R, Španjol Ž (2014) Testing vegetation flammability: the problem of extremely low ignition frequency and overall flammability score. Journal of Combustion 2014, 970218.
| Crossref | Google Scholar |
Khan MM, De Ris JL, Ogden SD (2008) Effect of moisture on ignition time of cellulosic materials. Fire Safety Science 9, 167-178.
| Crossref | Google Scholar |
Koide RT, Robichaux RH, Morse SR, Smith C (2000) Plant water status, hydraulic resistance and capacitance. In ‘Plant physiological ecology: Field methods and instrumentation’. (Eds RW Pearcy, J Ehleringer, HA Mooney, PW Rundel) pp. 161–184. (Chapman and Hall) 10.1007/978-94-010-9013-1
Kreye JK, Kane JM, Varner JM, Hiers JK (2020) Radiant heating rapidly increases litter flammability through impacts on fuel moisture. Fire Ecology 16(1), 8.
| Crossref | Google Scholar |
Lareau NP, Nauslar NJ, Abatzoglou JT (2018) The Carr Fire Vortex: a case of pyrotornadogenesis? Geophysical Research Letters 45(23), 13,107-13,115.
| Crossref | Google Scholar |
Madrigal J, Marino E, Guijarro M, Hernando C, Díez C (2012) Evaluation of the flammability of gorse (Ulex europaeus L.) managed by prescribed burning. Annals of Forest Science 69(3), 387-397.
| Crossref | Google Scholar |
Martin R (1994) Assessing the flammability of domestic and wildland vegetation. In ‘12th Conference on Fire and Forest Meteorology’. Jekyll Island, GA, USA, 26–28 November. pp. 26-28. 10.13140/RG.2.1.3999.3680
Matthews S (2010) Effect of drying temperature on fuel moisture content measurements. International Journal of Wildland Fire 19(6), 800-802.
| Crossref | Google Scholar |
McAllister S, Grenfell I, Hadlow A, Jolly WM, Finney M, Cohen J (2012) Piloted ignition of live forest fuels. Fire Safety Journal 51, 133-142.
| Crossref | Google Scholar |
McRae DJ, Alexander ME, Stocks BJ (1979) Measurement and description of fuels and fire behavior on prescribed burns: A handbook. Issue Information Report O-X-287. http://parkscanadahistory.com/publications/o-x-287.pdf
Melnik O (2016) A proposed experimental methodology for assessing the effects of biophysical properties and energy content on live fuel flammability. University of Alberta, Canada. 10.7939/R3862BH01
Melnik OM, Paskaluk SA, Ackerman MY, Melnik KO, Thompson DK, McAllister SS, Flannigan MD (2022) New in-flame flammability testing method applied to monitor seasonal changes in live fuel. Fire 5(1), 1.
| Crossref | Google Scholar |
Morandini F, Silvani X (2010) Experimental investigation of the physical mechanisms governing the spread of wildfires. International Journal of Wildland Fire 19(5), 570-582.
| Crossref | Google Scholar |
Morandini F, Santoni PA, Tramoni JB, Mell WE (2019) Experimental investigation of flammability and numerical study of combustion of shrub of rockrose under severe drought conditions. Fire Safety Journal 108(May),.
| Crossref | Google Scholar |
Morvan D, Dupuy JL (2004) Modeling the propagation of a wildfire through a Mediterranean shrub using a multiphase formulation. Combustion and Flame 138(3), 199-210.
| Crossref | Google Scholar |
Pearce HG (2018) The 2017 Port Hills wildfires - a window into New Zealand’s fire future? Australasian. Journal of Disaster and Trauma Studies 22(Special Issue), 35-50.
| Google Scholar |
Pearce HG, Anderson WR, Fogarty LG, Todoroki CL, Anderson SAJ (2010) Linear mixed-effects models for estimating biomass and fuel loads in shrublands. Canadian Journal of Forest Research 40(10), 2015-2026.
| Crossref | Google Scholar |
Pickard RW, Wraight H (1961) The Effect of Moisture on the Ignition and Flame Propagation of Thin Cellulosic Materials. Fire Research Station. https://publications.iafss.org/publications/frn/450/-1
Pickett BM, Isackson C, Wunder R, Fletcher TH, Butler BW, Weise DR (2010) Experimental measurements during combustion of moist individual foliage samples. International Journal of Wildland Fire 19(2), 153-162.
| Crossref | Google Scholar |
Porterie B, Loraud JC, Bellemare LO, Consalvi JL (2003) A physically based model of the onset of crowning. Combustion Science and Technology 175(6), 1109-1141.
| Crossref | Google Scholar |
Ramadhan ML, Carrascal J, Osorio A, Hidalgo JP (2021) The effect of moisture content and thermal behaviour on the ignition of Eucalyptus saligna leaves. International Journal of Wildland Fire 30(9), 680-690.
| Crossref | Google Scholar |
Reszka P, Cruz JJ, Valdivia J, González F, Rivera J, Carvajal C, Fuentes A (2020) Ignition delay times of live and dead pinus radiata needles. Fire Safety Journal 112(September 2019),.
| Crossref | Google Scholar |
Scarff FR, Gray BF, Westoby M (2012) Exploring phosphate effects on leaf flammability using a physical chemistry model. International Journal of Wildland Fire 21(8), 1042-1051.
| Crossref | Google Scholar |
Scarff FR, Lenz T, Richards AE, Zanne AE, Wright IJ, Westoby M (2021) Effects of plant hydraulic traits on the flammability of live fine canopy fuels. Functional Ecology 35(4), 835-846.
| Crossref | Google Scholar |
Stocks BJ, Alexander ME, Wotton BM, Stefner CN, Flannigan MD, Taylor SW, Lavoie N, Mason JA, Hartley GR, Maffey ME, Dalrymple GN, Blake TW, Cruz MG, Lanoville RA (2004) Crown fire behaviour in a northern jack pine - Black spruce forest. Canadian Journal of Forest Research 34(8), 1548-1560.
| Crossref | Google Scholar |
Sullivan AL (2017) Inside the inferno: fundamental processes of wildland fire behaviour: Part 2: Heat transfer and interactions. Current Forestry Reports 3(2), 150-171.
| Crossref | Google Scholar |
Varner JM, Kane JM, Kreye JK, Shearman TM (2021) Litter flammability of 50 Southeastern North American tree species: evidence for mesophication gradients across multiple ecosystems. Frontiers in Forests and Global Change 4, 727042.
| Crossref | Google Scholar |
Varner JM, Shearman TM, Kane JM, Banwell EM, Jules ES, Stambaugh MC (2022) Understanding flammability and bark thickness in the genus Pinus using a phylogenetic approach. Scientific Reports 12(1), 7384.
| Crossref | Google Scholar | PubMed |
Viegas DX, Viegas TSP, Ferreira D (1992) Moisture content of fine forest fuels and fire occurrence in central portugal. International Journal of Wildland Fire 2(2), 69-86.
| Crossref | Google Scholar |
Weise DR, White RH, Beall FC, Etlinger MG (2005) Use of the cone calorimeter to detect seasonal differences in selected combustion characteristics of ornamental vegetation. International Journal of Wildland Fire 14(3), 321-338.
| Crossref | Google Scholar |
Whight S, Bradstock R (1999) Indices of fire characteristics in sandstone heath. International Journal of Wildland Fire 9(2), 145-153.
| Google Scholar |
White RH, Zipperer WC (2010) Testing and classification of individual plants for fire behaviour: plant selection for the wildland–urban interface. International Journal of Wildland Fire 19(2), 213-227.
| Crossref | Google Scholar |
Wyse SV, Perry GLW, O’Connell DM, Holland PS, Wright MJ, Hosted CL, Whitelock SL, Geary IJ, Maurin KJL, Curran TJ (2016) A quantitative assessment of shoot flammability for 60 tree and shrub species supports rankings based on expert opinion. International Journal of Wildland Fire 25(4), 466-477.
| Crossref | Google Scholar |
Yashwanth BL, Shotorban B, Mahalingam S, Lautenberger CW, Weise DR (2016) A numerical investigation of the influence of radiation and moisture content on pyrolysis and ignition of a leaf-like fuel element. Combustion and Flame 163, 301-316.
| Crossref | Google Scholar |
Zhao W, Blauw LG, van Logtestijn RSP, Cornwell WK, Cornelissen JHC (2014) Interactions between fine wood decomposition and flammability. Forests 5(4), 827-846.
| Crossref | Google Scholar |
Appendix 1
Size/condition | Moisture content (%) | Initial wet weight (g) | Initial dry weight, estimated (g) | Volume (mL) | Density based on wet weight (g/mL) | Density based on dry weight (g/mL) | Time to ignition (s) | Peak heat release rate (kW/m2) | Mean heat release rate (kW/m2) | Burn duration (s) | Flaming heat release (kJ) | |
---|---|---|---|---|---|---|---|---|---|---|---|---|
Live needles | 0 | 3.94 ± 0.50 | 3.94 ± 0.50 | 9.46 ± 0.20 | 0.42 ± 0.05 | 0.42 ± 0.05 | 13.67 ± 4.93 | 663.57 ± 33.51 | 416.38 ± 30.91 | 12.67 ± 1.53 | 57.15 ± 9.77 | |
Live needles | 10 | 3.73 ± 0.19 | 3.39 ± 0.17 | 7.54 ± 0.47 | 0.49 ± 0.01 | 0.45 ± 0.01 | 13.33 ± 2.08 | 627.01 ± 39.84 | 369.60 ± 17.31 | 13.00 ± 2.00 | 51.53 ± 5.25 | |
Live needles | 25 | 4.12 ± 0.16 | 3.30 ± 0.13 | 9.25 ± 0.35 | 0.45 ± 0.00 | 0.36 ± 0.00 | 13.50 ± 0.71 | 541.12 ± 22.25 | 299.26 ± 57.07 | 18.50 ± 6.36 | 56.54 ± 7.92 | |
Live needles | 50 | 6.29 ± 0.15 | 4.19 ± 0.10 | 9.48 ± 0.23 | 0.66 ± 0.01 | 0.44 ± 0.01 | 27.00 ± 8.54 | 407.90 ± 34.45 | 190.85 ± 19.51 | 33.33 ± 3.51 | 65.57 ± 9.59 | |
Live needles | 75 | 6.46 ± 0.55 | 3.69 ± 0.32 | 10.85 ± 1.08 | 0.60 ± 0.01 | 0.34 ± 0.01 | 41.00 ± 14.42 | 396.94 ± 13.07 | 164.73 ± 49.71 | 36.67 ± 14.36 | 57.29 ± 2.25 | |
Live needles | 100 | 8.26 ± 0.28 | 4.13 ± 0.14 | 9.75 ± 0.41 | 0.85 ± 0.01 | 0.42 ± 0.00 | 45.33 ± 9.29 | 283.64 ± 66.71 | 169.00 ± 47.81 | 42.00 ± 19.16 | 66.57 ± 7.01 | |
Dead needles | 0 | 3.89 ± 0.18 | 3.89 ± 0.18 | 8.05 ± 1.36 | 0.49 ± 0.06 | 0.49 ± 0.06 | 13.67 ± 8.14 | 600.29 ± 79.72 | 377.97 ± 89.14 | 15.67 ± 4.04 | 60.93 ± 6.10 | |
Dead needles | 10 | 5.47 ± 0.17 | 4.97 ± 0.16 | 10.09 ± 0.29 | 0.54 ± 0.01 | 0.49 ± 0.01 | 25.00 ± 7.81 | 557.94 ± 52.40 | 253.67 ± 33.64 | 29.67 ± 5.51 | 76.86 ± 9.54 | |
Dead needles | 25 | 5.63 ± 0.30 | 4.51 ± 0.24 | 9.76 ± 0.59 | 0.58 ± 0.01 | 0.46 ± 0.01 | 30.00 ± 6.08 | 544.91 ± 47.18 | 234.33 ± 39.84 | 31.50 ± 7.78 | 74.61 ± 5.28 | |
Dead needles | 50 | 5.76 ± 0.38 | 3.84 ± 0.25 | 7.63 ± 0.90 | 0.76 ± 0.06 | 0.51 ± 0.04 | 35.67 ± 3.21 | 457.87 ± 38.07 | 148.54 ± 36.47 | 42.67 ± 13.32 | 61.66 ± 2.96 | |
Dead needles | 75 | 6.76 ± 0.44 | 3.86 ± 0.25 | 8.58 ± 0.50 | 0.79 ± 0.07 | 0.45 ± 0.04 | 34.00 ± 3.46 | 399.02 ± 26.57 | 126.31 ± 7.99 | 49.67 ± 3.51 | 63.87 ± 3.41 | |
Dead needles | 100 | 7.55 ± 0.61 | 3.78 ± 0.30 | 7.72 ± 0.83 | 0.98 ± 0.05 | 0.49 ± 0.02 | 43.33 ± 6.11 | 426.98 ± 29.12 | 167.25 ± 25.04 | 39.00 ± 4.00 | 66.25 ± 4.28 | |
Live twigs | 0 | 15.24 ± 0.82 | 15.24 ± 0.82 | 26.10 ± 1.90 | 0.58 ± 0.02 | 0.58 ± 0.02 | 33.33 ± 2.08 | 678.64 ± 18.81 | 400.16 ± 42.67 | 46.00 ± 3.46 | 187.13 ± 8.38 | |
Live twigs | 10 | 15.17 ± 1.33 | 13.79 ± 1.21 | 24.05 ± 1.63 | 0.63 ± 0.02 | 0.57 ± 0.02 | 56.33 ± 4.93 | 612.80 ± 58.56 | 365.07 ± 34.62 | 45.00 ± 10.58 | 165.56 ± 21.83 | |
Live twigs | 25 | 18.35 ± 1.04 | 14.68 ± 0.83 | 25.33 ± 1.49 | 0.72 ± 0.01 | 0.58 ± 0.01 | 57.67 ± 8.96 | 458.24 ± 53.34 | 272.54 ± 23.04 | 69.33 ± 10.02 | 190.49 ± 18.27 | |
Live twigs | 50 | 19.96 ± 1.03 | 13.31 ± 0.69 | 24.19 ± 0.77 | 0.83 ± 0.04 | 0.55 ± 0.03 | 99.00 ± 13.00 | 409.24 ± 89.76 | 195.16 ± 27.00 | 89.67 ± 12.50 | 174.80 ± 5.96 | |
Live twigs | 75 | 27.65 ± 0.33 | 15.80 ± 0.19 | 27.03 ± 0.55 | 1.02 ± 0.01 | 0.58 ± 0.00 | 115.00 ± 7.94 | 348.87 ± 75.82 | 167.86 ± 6.28 | 131.33 ± 6.51 | 221.88 ± 4.24 | |
Live twigs | 100 | 31.90 ± 1.06 | 15.95 ± 0.53 | 27.23 ± 0.68 | 1.17 ± 0.03 | 0.59 ± 0.01 | 203.33 ± 15.50 | 456.27 ± 50.36 | 207.23 ± 20.15 | 93.33 ± 8.14 | 194.40 ± 1.73 | |
Dead twigs | 0 | 13.49 ± 0.87 | 13.49 ± 0.87 | 24.33 ± 1.74 | 0.55 ± 0.01 | 0.55 ± 0.01 | 12.33 ± 4.51 | 512.33 ± 23.11 | 331.96 ± 18.20 | 54.00 ± 5.00 | 182.00 ± 7.95 | |
Dead twigs | 10 | 16.13 ± 1.07 | 14.66 ± 0.97 | 24.82 ± 1.52 | 0.65 ± 0.01 | 0.59 ± 0.01 | 50.33 ± 4.73 | 500.89 ± 62.71 | 293.66 ± 57.10 | 62.00 ± 10.58 | 181.14 ± 9.20 | |
Dead twigs | 25 | 16.50 ± 0.43 | 13.20 ± 0.34 | 22.93 ± 1.15 | 0.71 ± 0.03 | 0.57 ± 0.03 | 54.33 ± 14.43 | 401.69 ± 81.50 | 245.16 ± 29.49 | 70.67 ± 15.53 | 172.95 ± 19.43 | |
Dead twigs | 50 | 21.25 ± 0.20 | 14.16 ± 0.13 | 24.28 ± 0.65 | 0.88 ± 0.02 | 0.58 ± 0.01 | 91.33 ± 16.92 | 342.38 ± 38.24 | 195.25 ± 8.52 | 97.00 ± 15.59 | 190.79 ± 25.43 | |
Dead twigs | 75 | 23.82 ± 2.30 | 13.61 ± 1.32 | 23.67 ± 1.33 | 1.01 ± 0.06 | 0.57 ± 0.03 | 127.67 ± 7.57 | 354.18 ± 27.14 | 165.68 ± 20.53 | 109.33 ± 3.51 | 182.32 ± 17.10 | |
Dead twigs | 100 | 29.46 ± 0.88 | 14.73 ± 0.44 | 25.09 ± 1.05 | 1.18 ± 0.07 | 0.59 ± 0.04 | 180.00 ± 17.35 | 345.54 ± 60.86 | 190.57 ± 26.07 | 87.67 ± 16.07 | 166.64 ± 15.36 | |
Live stems | 0 | 25.97 ± 2.07 | 25.97 ± 2.07 | 42.76 ± 3.30 | 0.61 ± 0.00 | 0.61 ± 0.00 | 29.67 ± 8.50 | 521.41 ± 36.13 | 328.72 ± 34.62 | 93.67 ± 4.51 | 310.14 ± 17.90 | |
Live stems | 10 | 28.37 ± 2.47 | 25.79 ± 2.24 | 42.74 ± 2.54 | 0.66 ± 0.02 | 0.60 ± 0.02 | 79.67 ± 2.52 | 426.39 ± 53.26 | 237.37 ± 43.68 | 128.33 ± 27.97 | 300.17 ± 29.22 | |
Live stems | 25 | 26.63 ± 3.29 | 21.31 ± 2.63 | 39.13 ± 4.31 | 0.68 ± 0.01 | 0.54 ± 0.01 | 69.33 ± 16.17 | 379.70 ± 28.67 | 274.51 ± 21.05 | 99.33 ± 15.53 | 273.44 ± 26.42 | |
Live stems | 50 | 38.66 ± 1.09 | 25.78 ± 0.72 | 42.12 ± 1.11 | 0.92 ± 0.01 | 0.61 ± 0.01 | 187.67 ± 24.44 | 367.11 ± 22.09 | 205.13 ± 7.52 | 134.00 ± 10.82 | 276.63 ± 18.29 | |
Live stems | 75 | 45.64 ± 0.68 | 26.08 ± 0.39 | 42.75 ± 0.76 | 1.07 ± 0.00 | 0.61 ± 0.00 | 205.33 ± 34.00 | 308.47 ± 42.37 | 184.30 ± 18.72 | 171.00 ± 32.42 | 312.96 ± 26.21 | |
Live stems | 100 | 53.48 ± 1.34 | 26.74 ± 0.67 | 44.38 ± 1.60 | 1.21 ± 0.02 | 0.60 ± 0.01 | 306.67 ± 36.47 | 318.23 ± 18.56 | 172.68 ± 29.77 | 170.33 ± 48.58 | 286.25 ± 37.44 | |
Dead stems | 0 | 24.41 ± 2.49 | 24.41 ± 2.49 | 42.26 ± 2.98 | 0.58 ± 0.08 | 0.58 ± 0.08 | 20.67 ± 10.26 | 488.44 ± 20.60 | 294.64 ± 23.14 | 98.33 ± 2.52 | 292.69 ± 24.20 | |
Dead stems | 10 | 27.89 ± 1.10 | 25.36 ± 1.00 | 40.26 ± 0.41 | 0.69 ± 0.02 | 0.63 ± 0.02 | 43.33 ± 15.50 | 403.46 ± 23.91 | 272.39 ± 11.19 | 114.33 ± 5.03 | 313.90 ± 10.90 | |
Dead stems | 25 | 32.98 ± 1.39 | 26.38 ± 1.11 | 40.69 ± 1.42 | 0.81 ± 0.01 | 0.65 ± 0.00 | 79.67 ± 38.40 | 327.72 ± 57.33 | 232.89 ± 42.24 | 146.00 ± 50.12 | 328.26 ± 58.56 | |
Dead stems | 50 | 39.37 ± 2.43 | 26.24 ± 1.62 | 39.96 ± 0.68 | 0.99 ± 0.07 | 0.66 ± 0.04 | 135.67 ± 35.53 | 293.70 ± 17.55 | 176.64 ± 15.91 | 182.33 ± 33.17 | 320.78 ± 38.40 | |
Dead stems | 75 | 41.53 ± 2.54 | 23.73 ± 1.45 | 41.86 ± 1.80 | 0.99 ± 0.04 | 0.57 ± 0.03 | 196.33 ± 40.80 | 232.31 ± 9.76 | 137.46 ± 15.69 | 189.67 ± 23.35 | 263.54 ± 53.82 | |
Dead stems | 100 | 45.42 ± 2.39 | 22.71 ± 1.20 | 42.33 ± 4.43 | 1.08 ± 0.06 | 0.54 ± 0.03 | 232.33 ± 51.78 | 212.44 ± 11.35 | 127.20 ± 11.51 | 191.33 ± 43.36 | 244.08 ± 53.46 |
The values provided are the mean and the standard deviation for each size/condition/moisture content combination, summarised across three replicates. Initial dry weight was estimated from the initial wet weight of the sample tested in the cone calorimeter and the moisture content of the ‘MC2’ sample.