Heat input determines the response and rapid recovery of post-fire soil microbial biomass
Rong She A , Jing-Chao Li

A
B
C
Abstract
The post-fire recovery of soil microbes is critical for ecological conservation, yet the mechanisms behind it are not well understood.
In this study, we examined the recovery patterns of culturable soil microbes following a fire.
A field experiment was conducted in which a forest soil was subjected to surface fire, and the culturable microbial biomass and soil physicochemical characteristics were evaluated 1 day after the fire, and subsequently every 10 days for 90 days.
Microbial biomass significantly reduced post-fire, with varying effects across microbial taxa and soil layers. The recovery patterns of microbial biomass at topsoil (0–10 cm) and subsoil (10–20 cm), and among different microbial taxa were also different and were determined by the residual microbiomes. Heat released during a fire (the combination of heat duration and temperature reached during treatment) was significantly related to the decrease and recovery of microbial biomass, whereas there was no relationship between soil physicochemical properties and microbial biomass recovery.
Soil microbial biomass recovered quickly post-fire, which can be mainly due to the rapid attenuation of heat along the soil profile. Heat released during fire was the key factor determining the residual biomass, and the residual microbiomes determined the recovery patterns of the various taxa that comprise the culturable microbial biomass.
Due to the complexity of natural fire, simulated fire experiment and systematic sampling based on space (soil profile) and time are crucial to investigate the dynamics of soil microbes post-fire.
Keywords: dormant species, fire ecology, fire disturbance, peak temperature, pure culture, restoration ecology, soil depth, soil microorganisms.
Introduction
Fire has a long history that can be traced back 450 million years (Belcher et al. 2013), and is a major environmental factor that affects the formation and maintenance of biodiversity (Certini 2014; Maurin et al. 2014; Pausas and Ribeiro 2017; He et al. 2019). While high-intensity fires can cause a dramatic reduction in biodiversity that is often irreversible, low-intensity burns can boost biodiversity (He et al. 2019). Early inhabitants in China used to burn wild areas in the spring, to increase supplies of large edible fungi and fodder grass (Hart et al. 2005). Departments that are responsible for the management and conservation of forests frequently use prescribed burning as a fire prevention strategy. However, the optimal intensity of fire for supporting forest biodiversity maintenance and preservation remains to be determined (Lazarina et al. 2019; Leverkus et al. 2019; Jones and Tingley 2021). Fire intensity represents the energy released during a fire, which can be described by metrics such as flame height, reaction intensity, duration, radiant energy, and temperature ranges during the fire (Keeley 2009). Different fire intensities have different effects on the ecosystem, and the pattern of post-fire recovery in the forest ecosystem will vary accordingly.
The role of microbial communities in the material and energy cycles of belowground ecosystems is crucial for maintaining the structural and functional stability of aboveground ecosystems (van der Heijden et al. 2008; Coban et al. 2022). These communities are key biological factors in the overall restoration of post-fire forest ecosystems (Fittipaldi et al. 2012; van der Bij et al. 2018). Understanding microbial responses to various fire durations and their recovery dynamics can provide a theoretical basis for determining best practices for post-fire restoration (Dangi et al. 2010; van der Bij et al. 2018; Averill et al. 2022; Coban et al. 2022).
Depending on the fire intensity, surface soil peak temperatures during a forest fire range from 300 to 350°C; In extreme fire events, temperatures more than 1500°C have been recorded (Mataix-Solera et al. 2009; Certini et al. 2021), resulting in soil microbial death (Certini et al. 2021). Since soil is a poor thermal conductor, the immediate lethal effect of heat rapidly diminishes with increasing soil depth (Enninful and Torvi 2008). Most research on the effects of natural fires on soil microbes has been conducted post-fire in the field (Mataix-Solera et al. 2009; Mataix-Solera et al. 2011; Agbeshie et al. 2022), preventing real time monitoring of soil ecosystems during and after a fire. For instance, monitoring the temperature dynamics in different soil layers throughout a fire’s duration or tracking post-fire microbial dynamics in various soil layers was not feasible. Experiments that simulate fires can be used to study the dynamics of soil ecosystems (Lucas-Borja et al. 2019; She et al. 2022).
Investigations of microbiomes have mainly been carried out using high-throughput sequencing, which cannot distinguish between dead and living cells and thus, cannot accurately reflect the activity of soil microbes (Fittipaldi et al. 2012). Soil microbial biomass relates not only to biochemical processes and the soil nutrient composition and transformation but also represents the biological activity in the soil, which is a main factor in maintaining and restoring forest productivity (Devi and Yadava 2006; Rawat et al. 2021). A reduction in microbial biomass, activity, or diversity may lead to the decline of multiple ecosystem functions (Wagg et al. 2014). Furthermore, as different soil microbial taxa have distinct functions and respond variably to fire, the recovery patterns of different microbial taxa may significantly differ (Dunn et al. 1985; Owen et al. 2019). Therefore, controlled burning experiments that continuously monitor changes in microbial biomass in different soil layers after a fire can offer better insights into the recovery patterns of the soil microbiome.
In view of the importance of heat on soil microbes during a fire, we used the plate count method (Holden and Treseder 2013; Ben-David and Davidson 2014; Sultana et al. 2021; Naimoddin Mirza and Sudhakarrao Patil 2022) in our field experiments to monitor the effects of surface fire duration on the culturable microbial biomass in different soil layers. We also examined the recovery patterns of soil microbial biomass following the fire, as well as the reaction of soil microbial biomass by monitoring changes in temperature and soil physicochemical properties in different soil layers.
Materials and methods
Experimental plot construction and burning treatments
We collected strongly acidic, yellow-red soil from the 0–20 cm depth of the soil layer in the Pinus yunnanensis dominated forest within Cangshan National Nature Reserve, Dali, Yunnan, China (25°40′N, 100°09′E; elevation, 2233 m a.s.l.). After sieving through a 5-mm mesh to remove large branches, decaying leaves, and stones, the soil was thoroughly mixed with a garden spade until a homogeneous mixture was obtained. A rectangular plot (1.3 m × 1.7 m; 50 cm deep) was excavated at the field practice base of Dali University (25°41′N, 100°10′E; elevation, 2009 m a.s.l.), which is located in a rural area. To reduce introduction of microbes from the field soil, the previously collected and mixed forest soil was filled into the excavated plot to a height of 30 cm. The plot was then divided into four treatments using red bricks, with the partition walls being 10 cm higher than the surrounding soil level to prevent rainwater infiltration. Finally, each treatment was further divided into nine areas measuring 10 cm × 10 cm, separated by stainless-steel plates (Fig. 1, see Supplementary Fig. S1).
Schematic of the sample plot. Sampling units are identified in green. The bricks were used to divide the plot into four sub-plots. (a) Vertical view and (b) front view where the light-coloured rectangle indicate previously collected mixed forest soil used to reduce introduction of microbes from the field soil. C, control (no fire/heat treatment); T1, treated with blow torch for 20 min; T2, treated with blow torch for 60 min; T3, treated with charcoal for 4 h.
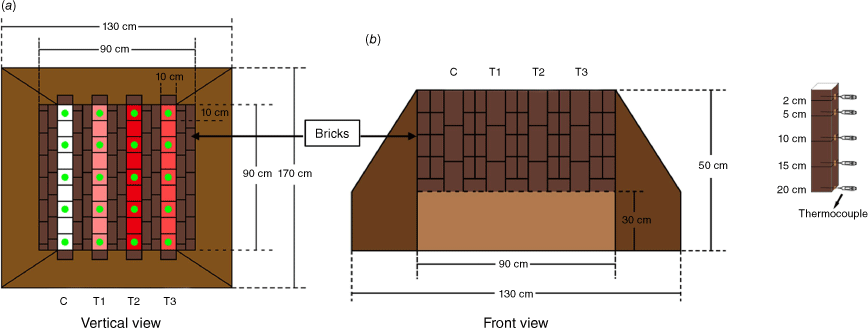
This experimental approach simulated only the heat effect of a forest fire, while excluding the deposition of aboveground burned biomass on the soil surface. The impact of a forest fire on soil microbes was simulated using a blow torch (Fig. S2) or charcoal. The four experimental treatments were: (1) T1, a short-term heat treatment applied with a blow torch for 20 min (2) T2, a medium-term heat treatment applied with a blow torch for 60 min; (3) T3, a long-term heat treatment applied with charcoal wrapped in aluminium foil for 4 h; and (4) C, a control treatment with no fire or heat application. Details are in Table 1.
Group | Detailed treatment methods | |
---|---|---|
C | No fire/heat treatment. | |
T1 | The blow torch was turned to the mid-range, and the flame was sprayed on the units for 20 min. | |
T2 | The blow torch was turned to the mid-range, and the flame was sprayed on the units for 60 min (1 h). | |
T3 | 200 g charcoal was put into tin foil paper and then placed on the units to bake the soil for 4 h. |
Soil temperature
Soil temperature during the experiment was monitored. To prevent disturbance to the soil profile during temperature testing, particularly when removing the thermocouple, we conducted a separate plot for this purpose. Five thermocouples were used per unit, placed at soil depths of 2, 5, 10, 15, and 20 cm. Each thermocouple was horizontally inserted at the centre of a square, corresponding to its specific soil depth. Temperatures were manually recorded at 5-min intervals (Fig. 1).
Soil sample collection
Soil samples were collected immediately after the soil had cooled (Day 1), followed by collections every 10 days (Day 10, 20, 30, 40, 50, 60, 70, 80, and 90). At each sampling time, five units out of nine were chosen from each treatment (Fig. 1). The soil was collected using an ad hoc sampler (Fig. S3) at depths of 0–10 cm (topsoil) and 10–20 cm (subsoil). Soil samples from the five units within the same treatment were then combined and labelled according to their treatment group (C, T1, T2, T3) for both topsoil and subsoil samples. For each treatment, a total of 20 composite samples (10 topsoil and 10 subsoil) were collected at each of the 10 timepoints, resulting in a total of 80 samples evaluated for the overall study.
Measurement of microbial biomass
The dilution plate count method was used to determine the culturable viable microbial biomass (Holden and Treseder 2013; Ben-David and Davidson 2014; Sultana et al. 2021; Naimoddin Mirza and Sudhakarrao Patil 2022). The media used were:
for bacteria, beef extract peptone medium (containing 3 g beef extract, 10 g peptone, 5 g NaCl, 19 g agar and 1000 mL deionised water, pH 7.4–7.6);
for fungi, Bengal red medium (containing 5 g peptone, 10 g glucose, 1 g KH2PO4, 0.5 g MgSO4·7H2O, 0.22 mL 30 g/L penicillin, 0.156 mL 50 g/L streptomycin, 100 mL 0.33 g/L Rose Bengal Na salt, 19 g agar and 1000 mL deionised water);
for actinomycetes, Gao’s No. 1 culture medium (containing 20 g of soluble starch, 1 g KNO3, 0.5 g K2HPO4, 0.5 g MgSO4·7H2O, 0.5 g NaCl, 0.01 g FeSO4·7H2O, 10 mL 0.1% potassium dichromate, 19 g agar and 1000 mL deionised water).
Bacterial biomass was determined by adding 10 g of each soil sample to 90 mL sterile water, shaken for 5 min, and then allowing the mixture to stand for 5 min. A volume of 1 mL of the supernatant was diluted to 10−2, 10−3, 10−4, 10−5, and 10−6 with sterile water. Three appropriately diluted levels were selected and 150 μL of each dilution was spread onto beef extract peptone medium plates. This procedure was repeated three times for each dilution level, resulting in N = 3 analytical replicates per dilution level, per treatment, and per timepoint. All the plates were incubated at 30°C for 2 days, after which the colonies on each plate were counted to quantify microbial biomass, and recorded as colony forming units (CFU).
The same serial dilution procedure was used to quantify fungal biomass on Bengal red medium, with dilution ratios adjusted to 10−1, 10−2, 10−3 and 10−4 because fungal biomass is in general lower than bacterial biomass. Plates were incubated at 28°C for 4 days before counting the microbial colonies, and recorded as colony forming units (CFU).
For actinomycetes, quantification was made on Gao’s No. 1 medium, and the serial dilution ratios were modified to 100, 10−1, 10−2 and 10−3 because the biomass of actinomycetes was lower than bacteria. Plates were incubated at 28°C for 7 days, after which the microbial colony numbers were counted, and recorded as colony forming units (CFU).
Measurement of soil physicochemical properties
Soil physicochemical properties were monitored on each sampling occasion. The soil gravimetric moisture content (% GMC) was determined after oven drying a subsample at 105°C until the weight was stable (24–48 h). Measurements of soil pH, organic matter (OM), total nitrogen (TN), and total phosphorus (TP) were conducted on air-dried samples. The soil was ground and passed through a 20-mesh sieve for pH analysis and a 100-mesh sieve for the others. Soil pH was measured using a glass electrode in a 1:2.5 w/v soil-to-water mixture. Organic matter was quantified via the K2Cr2O7 oxidation method. TN was determined through the Kjeldahl method. TP was determined with NaHCO3 extraction and H2SO4–HClO4 digestion, and analysed using the molybdenum blue method.
Data analysis
For colony counts of bacteria, fungi, actinomycetes, we referred to the book ‘Brock Biology of Microorganisms’ (Madigan et al. 2011). If there were two dilutions containing the appropriate range of colonies, we employed the following equation:
where N is the number of colonies in 1 g sample; ΣC is the sum of colonies on the plates (on plates containing appropriate number of colonies); n1 is the number of plates of the first dilution (low dilution); n2 is the number of plates of the second dilution (high dilution); and d is the dilution factor (first dilution).
Total microbial biomass was calculated as the sum of bacterial, fungal and actinomycetes biomass. The microbial biomass of the entire soil profile (0–20 cm) was determined by averaging the microbial biomass values of the topsoil (0–10 cm) and subsoil (10–20 cm). Similarly, the physiochemical properties of the entire soil profile (0–20 cm) were obtained by averaging the values from the topsoil and subsoil. A graph showing the relationship between temperature and microbial biomass was created using GraphPad Prism ver. 9.5.0. Based on the area under the temperature curve, the amount of heat transmitted to each soil layer was calculated using Origin 8.0 software. SPSS was used for statistical analysis. A test for homogeneity of variance was conducted to first determine whether the variance was homogeneous. If the variance was homogeneous, a one-way ANOVA was used, and the least significant difference was used for the inter-treatment pairwise test. In cases of non-homogeneous variance, a Welch ANOVA and Games Howell test were used. The relationships between heat released during treatment, or peak temperature, and soil microbial biomass on Day 1 were analysed through linear regression using GraphPad Prism ver. 7.0. A Generalised Linear Model (GLM) was applied to assess the effects of soil physicochemical properties on microbial biomass during the whole experimental period.
Results
Temperature dynamics and heat released during treatment
The maximum temperatures at soil depths of 2 cm, 5 cm, and 10 cm following the cessation of heat were 385.0°C, 46.7°C, and 18.9°C, respectively. After the heating cessation, the temperature at a depth of 2 cm dropped quickly, whereas it gradually increased at a depth of 5 cm, reaching 75.6°C after 30 min. The peak temperature at a depth of 10 cm was observed to be 29.6°C at 40 min post-heating. Temperature below a depth of 10 cm remained constant during the whole experiment. The area under the temperature curve, representing the total heat released during the heating phase, was calculated to be 15 667.50 at a soil depth of 2 cm (Figs 2 and 5) (the area under temperature curves are shown in Fig. 5b as ‘heat’).
Dynamic of soil temperature at different soil depth during heating treatment. Each temperature curve is from a single thermocouple. (a): treated with blow torch for 20 min. (b): treated with blow torch for 60 min. (c): treated with charcoal for 4 hours. numbers in legends represent soil depth (cm). Numbers in legends represent soil depth.

The temperatures at soil depths of 2 cm, 5 cm, and 10 cm immediately after heating cessation were 639°C, 84.4°C, and 26.2°C, respectively. The temperature at a depth of 2 cm declined then linearly, while at a depth of 5 cm it progressively rose, reaching 149.3°C before it began to decrease. At 90 min following the cessation of heating, the temperatures at depths of 2 cm, 5 cm, 10 cm, 15 cm, and 20 cm were 96°C, 147.8°C, 56.7°C, 26.5°C, and 14.9°C, respectively. Notably, the temperature at a depth of 5 cm, the temperature remained above 80°C for a duration of 110 min. The area under the temperature curve at a depth of 2 cm was significantly higher, measuring 53231.75 (Figs 2 and 5) (the area under temperature curves are shown in Fig. 5b as ‘heat’).
The soil temperatures at soil depths of 2 cm, 5 cm, and 10 cm immediately after heating cessation were 258°C, 110.5°C, and 45.7°C, respectively. However, the peak temperatures were 358°C, 112.3°C, and 46.1°C, respectively. The temperature at a depth of 5 cm sustained above 80°C for 200 min, and between 30°C and 40°C for 300 min at a depth of 10 cm. The temperature increased to 19°C and above at a depth of 15 cm and remained at this level for over 100 min, with no change observed at a depth of 20 cm. The area under the temperature curve at a depth of 2 cm was the largest among the treatments, recorded at 75,662.5 (Figs 2 and 5) (the area under temperature curves are shown in Fig. 5b as ‘heat’).
Dynamics of the total microbial biomass
Within 90 days, there was a highly significant difference (P < 0.001) among the four treatments at each assessment point. When compared to the control treatment (C), the biomass of the three experimental treatments exhibited an immediate decline post-fire (Day 1), with T2 and T3 experiencing particularly pronounced decreases. By Day 10, while the biomass of T2 and T3 exceeded that of T1, this difference was not statistically significant (P > 0.05); however, their biomass levels remained significantly lower than those of the control (P < 0.001). On Day 20, the biomass levels of all experimental treatments surpassed that of the control (P < 0.001) with T1 displaying significantly lower biomass compared to T2 and T3 (P < 0.001). Subsequently, the biomass levels of the experimental treatments were all higher than the control treatment except on Day 40 and Day 90 when the biomass of T3 was lower than the control, and on Day 60 and 90 when the biomass of T2 was lower than the control (Fig. 3, Supplementary Table S1).
Total microbial biomass from (a) topsoil layer (0–10 cm), (b) subsoil layer (10–20 cm), and (c) entire soil (0–20 cm). There was significant difference among treatments at each timepoint. C, control without fire/heat treatment; T1, treated with blow torch for 20 min; T2, treated with blow torch for 60 min; T3, treated with charcoal for 4 h.
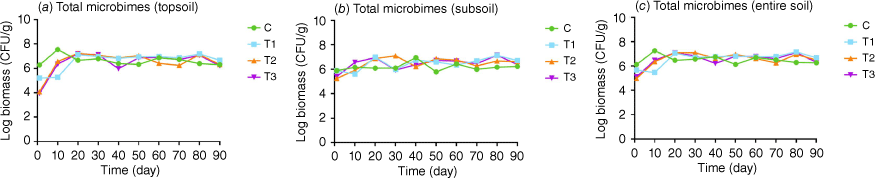
Within 90 days, a highly significant difference in microbial biomass (P < 0.001) was noted among the four treatments at each assessment point. When compared to the control treatment (C), on Day 1 the biomass of T2 and T3 was significantly reduced, whereas T1 showed no significant disparity from the control (P > 0.05). By Day 10, the biomass of T3 was significantly higher than that of the control while that of T1 was significantly lower than that of the control (P < 0.001), and T2 was also significantly lower than that of the control, albeit to a lesser extent (P < 0.05). On Day 20, the biomass of all experimental treatments was significantly greater than that of the control (P < 0.001). Beyond Day 20, the biomass levels of all experimental treatments remained above those of the control at each assessment point, with an exception noted on Day 40 (Fig. 3, Table S1).
The total microbial biomass of the three treatments decreased significantly following the simulated fire treatment, with an average reduction of 1.11 × 106 CFU/g compared to the control on Day 1, equating to a decrease of 82.76%. Within the 90 days, a highly significant difference in microbial biomass (P < 0.001) was detected among the four treatments at each timepoint, with the biomass dynamics of all treatments mirroring those observed in the topsoil layer (Fig. 3, Table S1).
Dynamics of bacterial biomass
Within 90 days, a highly significant variation (P < 0.001) was observed among the four treatments at each assessment point, with the exceptions being on Day 30 and Day 70, when the differences were significant (P < 0.05) (Fig. 3). When compared to the control treatment (C), the biomass of the three experimental treatments decreased immediately following the fire, especially for T2 and T3. The biomass of T1 initially decreased by Day 10, subsequently increasing to surpass that of the control by Day 20, a trend that persisted until the conclusion of the experiment. The biomass of T2 and T3 began to increase by Day 10, exceeding that of the control by Day 20 and maintaining this advantage for the majority of the assessment period. However, on Day 40, the biomass of T3 was lower than that of the control, though not significant (P > 0.05). Notable declines below the reference levels of the control were observed for T2 on Days 60 and 70 (Fig. 4, Table S2).
Log microbial biomass of (a) bacteria, (b) fungi, and (c) actinomyces in the topsoil (0–10 cm), subsoil (10–20 cm), and the entire soil (0–20 cm) following fire or heat treatment. Means and s.e. are from three replicates. C, control without fire/heat treatment; T1, treated with blow torch for 20 min; T2, treated with blow torch for 60 min; T3, treated with charcoal for 4 h. n.s., no significant difference between treatments.
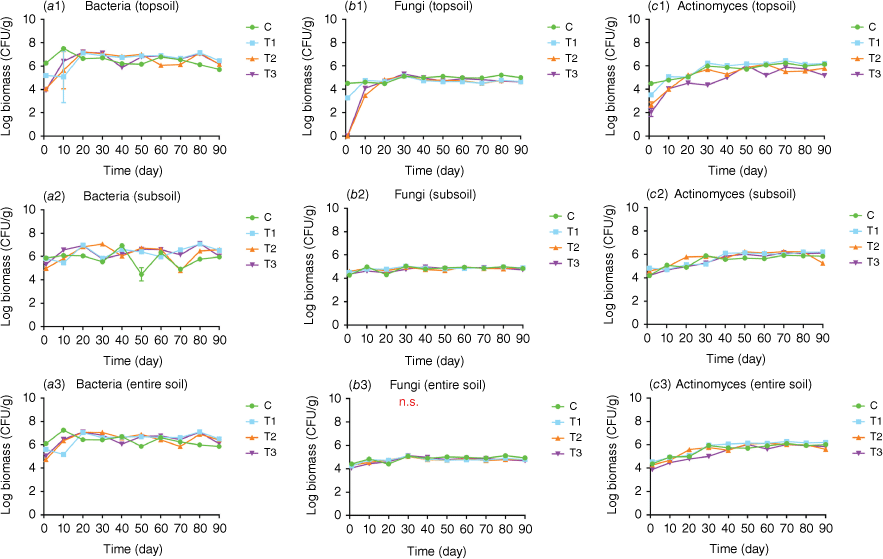
At each assessment point, there was a consistent highly significant difference (P < 0.001) among the four treatments. The trends in bacterial biomass changes in the three experimental treatments during the initial 20 days following the fire, mirrored those in the topsoil. Immediately after the fire, the bacterial biomass of T2 and T3 treatments decreased immediately, while T1 exhibited no noticeable change. The subsequent bacterial biomass changes in three experimental treatments closely paralleled those of the topsoil bacteria on Days 20 and 30 and were higher than those of the control treatment (Fig. 4, Table S2).
Over the whole experimental period, bacteria biomasses of the three treatments decreased by 85.00% compared to the control treatment, with the greatest decrease observed in T2 (95.71%) and the smallest in T1 (67.92%). The patterns of change in bacterial biomass in the entire soil layer were akin to those in the topsoil, except on Day 40 when the biomass of T3 was highly significantly lower than that of the control (P < 0.001) (Fig. 4, Table S2).
Dynamics of fungal biomass
Throughout the entire experiment, there was a very significant difference among the four treatments (P < 0.001); However, the changes in fungal biomass were generally consistent among the three experimental treatments. The biomass of T1, T2 and T3 all exhibited an immediate decrease following the fire, with treatments T2 and T3 experiencing more pronounced declines. By Day 10, the biomass in T1 exceeded that of the control treatment. By Day 20, the biomass of all experimental treatments surpassed that of the control treatment, subsequently declining and remaining highly significantly lower than the control treatment until the end (Fig. 4, Table S3).
There was a highly significant difference among the four treatments over the whole experiment (P < 0.001), with the exception on Day 70, when the difference was significant (P < 0.05). On Day 1, the biomass of T1 (P < 0.05) and T2 (P < 0.001) was significantly higher than the control. The trends in biomass change during the initial 30 days in T1, T2 and T3 followed a similar pattern to that of the control treatment. Throughout the first 30 days, the biomass of T3 was lower than that of T1 and T2 but peaked on Day 40, becoming higher than that of the other treatments (P < 0.001) (Fig. 4, Table S3).
Over the whole experimental period, bacteria biomasses of the three treatments decreased by 35.74% compared to the control group, with the largest decrease observed in T3 (54.08%) and the smallest in T2 (21.03%). There was a highly significant difference among the four treatments at each timepoint throughout the experiment (P < 0.001) except on Day 30, when there was no significant difference among treatments. The changes in biomass of each treatment were analogous to those observed in the subsoil (Fig. 4, Table S3).
Dynamics of actinomycetes biomass
Among the four treatments, there was a highly significant difference Throughout the entire experiment (P < 0.001), except for Day 20 when the difference was merely significant (P < 0.05). On Day 1, the biomass of actinomycetes in all three experimental treatments was highly significantly lower than that of the control treatment (P < 0.001), with T3 exhibiting the pronounced decline. However, on Day 10, the biomass began to increase in all treatments. T1 maintained a higher biomass than the control from Day 10 onwards. The s biomass of T2 exceeded that of the control treatment on Days 20, 50, and 60. The biomass of T3 remained lower than that of the control throughout the experiment, except on Day 50 (Fig. 4, Table S4).
There was a highly significant difference (P < 0.001) among the four treatments. On Day 1, T1 and T2 exhibited greater biomass than the control, while the biomass of T3 was comparable to that of the control. On Days 10 and 30, the biomass in the experimental treatments was lower than that of the control. On Day 20, the biomass in the experimental treatments was higher than that of control. Except for Day 10, the biomass changes in T3 and T1 were essentially identical. After 40 days, the biomass of the three experimental treatments was higher than that of the control, yet it sharply decreased in T2 on Day 90 (Fig. 4, Table S4).
Throughout the whole experiment, the biomasses of bacteria in the three treatments decreased by 11.45% compared to the control group, with the largest decrease observed in T3 (66.70%) and the smallest in T1 (−50.57%). There was a highly significant difference (P < 0.001) among the four treatments at each timepoint, and the dynamics of biomass changes in each treatment were similar to those in the subsoil (Fig. 4, Table S4).
Change in soil physicochemical properties
There was a highly significant difference (P < 0.001) among the four treatments in terms of pH value at each timepoint, except on Day 20 where the difference was not significant (P > 0.05). For GMC, there was a highly significant difference (P < 0.001) among the four treatments at each timepoint except on Days 10 and 60 (P > 0.05); Regarding TN, The differences were significant(P < 0.05) on Days 50 and 80, and highly significant (P < 0.001) on all other timepoints. For TP, only on Day 10 there was significant difference (P < 0.05) among the four treatments. For OM, there was a highly significant difference (P < 0.001) among the four treatments at each timepoint except Days 10 and 50 when the difference was significant (P < 0.05) and there was no significant difference on Day 60 (P > 0.05) (Fig. 5, Table S5).
Soil physicochemical properties for (a) pH, (b) GMC, (c) TN, (d) TP, and (e) OM in the topsoil layer (0–10 cm), subsoil layers (10–20 cm), and entire soil (0–20 cm) following fire or heat treatment. Means and s.e. are from three replicates. C, control without fire/heat treatment; T1, treated with blow torch for 20 min; T2, treated with blow torch for 60 min; T3, treated with charcoal for 4 h.
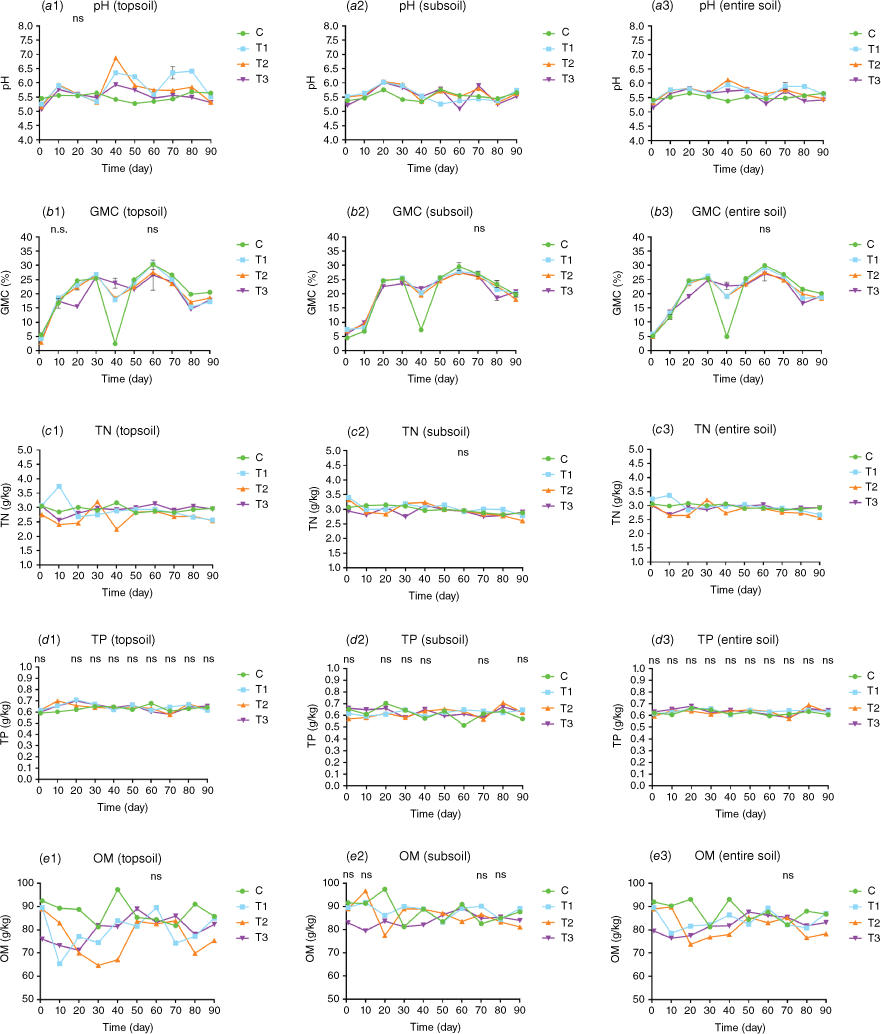
There was a highly significant difference (P < 0.001) among the four treatments in terms of pH value at each timepoint. For GMC, there was a highly significant difference (P < 0.001) among the four treatments at each timepoint except on Day 70 (P > 0.05) and the difference was significant on Day 50, 60, 80. For TN, there was a highly significant difference (P < 0.001) at each timepoint except on Days 20, 50, 70 when the difference was significant (P < 0.05) and Day 60 there was no significant difference (P > 0.05). For TP, there was a highly significant difference (P < 0.001) on Day 60 and 80, significant difference (P < 0.05) on Days 1 and 50. For OM, there was a highly significant difference (P < 0.001) among the four treatments at each timepoint except on Day 90 when the difference was significant and there was no significant difference on Days 1, 10, 70, 80 (Fig. 5, Table S5).
There was a highly significant difference (P < 0.001) among the four treatments in pH value at each timepoint except Days 30 and Day 70 when the difference was just significant (P < 0.05). For GMC, there was a highly significant difference (P < 0.001) among the four treatments at each timepoint except on Day 60 (P > 0.05) and the difference was significant on Days 10, 70. For TN, There was a highly significant difference (P < 0.001) at each timepoint except on Days 50, 70, 80 when the difference was significant (P < 0.05). For TP, there was no significant difference (P < 0.05) among the four treatments at each timepoint. For OM, there was a highly significant difference (P < 0.001) among the four treatments at each timepoint except on Days 1, 10, 50, 60, 80 when the difference was significant and there was no significant difference on Day 70 (Fig. 5, Table S5).
Relationship between microbial biomass on Day 1 and peak temperature or heat released during the fire
Regression analysis indicated no significant correlation between the log microbial biomass of any of the three microbial taxa (bacteria, fungi, actinomycetes) on Day 1 and the peak soil temperature at a depth of 2 cm (P > 0.05). However, the log microbial biomass of these three taxa on Day 1 exhibited a significant linear correlation with the amount of heat released during the treatment at a soil depth of 2 cm (P < 0.05) (Fig. 6).
Correlation between soil microbial biomass and physicochemical properties
Throughout the whole experimental period, the log bacterial biomass of treatment T1 in the topsoil exhibited a significant positive association with pH, OM, and GMC and a negative association with TN (P < 0.05). The log actinomycetes biomass in treatment T1 in the topsoil showed a significant negative association with TN and TP and a positive association with GMC (P < 0.05). The log fungal biomass of treatment T2 and T3 in the topsoil demonstrated a significant positive correlation with GMC (P < 0.05). No significant correlation was observed between log microbial biomass and physicochemical properties in the other treatments (Table 2).
Soil layer | Taxon | Treatment | pH | MOT | TN | TP | GMC | |
---|---|---|---|---|---|---|---|---|
Topsoil (0−10 cm) | Actinomyces | C | 0.1745 | (0.4215) | (0.2660) | 15.2021 | (0.0409) | |
T1 | 0.7331 | 7.8215* | (1.6390)5* | (16.4565)* | (0.0618) | |||
T2 | 0.1388 | 11.4857 | 0.0568 | (5.2259) | (0.0241) | |||
T3 | 1.4384 | 3.5698 | 0.4362 | 5.2292 | 0.1042 | |||
Bacteria | C | 0.2740 | (1.0638) | (3.1807) | 0.2249 | 0.0226 | ||
T1 | 0.6428* | 4.8931* | (2.1136)* | 8.9813 | 0.0544* | |||
T2 | 0.2707 | 7.6571 | (0.2570) | 9.2880 | (0.0399) | |||
T3 | (0.4026) | 10.4174 | (0.0242) | 13.2884 | (0.0179) | |||
Fungi | C | 0.2773 | (1.5144) | (1.5196) | 8.1173 | (0.0051) | ||
T1 | 0.1455 | 3.9544 | (0.5079) | (2.7475) | (0.0386) | |||
T2 | 0.1633 | 17.4777* | (0.8646) | 0.1930 | (0.0564) | |||
T3 | 0.5943 | 16.1511 | (0.3986) | 15.6812 | 0.0130 | |||
Subsoil (10−20 cm) | Actinomyces | C | 0.1112 | 2.3730 | (0.6911) | (2.9883) | (0.0551) | |
T1 | (1.2433) | 3.3305 | (1.3655) | 0.9246 | (0.0365) | |||
T2 | (1.9767) | 6.6640 | (0.6379) | (1.4736) | (0.0162) | |||
T3 | (0.5632) | 10.3908 | (1.0478) | 4.3466 | (0.0132) | |||
Bacteria | C | (2.4241) | 0.6023 | (0.3120) | (4.1663) | 0.1062 | ||
T1 | 0.3474 | 1.9124 | (0.9733) | (5.2740) | (0.1183) | |||
T2 | 2.7117 | (0.5105) | 0.4316 | 18.4360 | 0.0253 | |||
T3 | 0.5749 | 5.7577 | (1.5816) | 16.6195 | 0.0558 | |||
Fungi | C | 0.0548 | 0.3926 | 0.4865 | (2.7864) | (0.0373) | ||
T1 | 0.0488 | 1.1187 | (0.2748) | (0.2946) | 0.0058 | |||
T2 | (0.3203) | 1.4940 | (0.3026) | (1.6297) | 0.0087 | |||
T3 | (0.4077) | 3.3896 | (0.1036) | (0.6664) | (0.0402) |
C, control without fire/heat treatment; T1, treated with blow torch for 20 min; T2, treated with blow torch for 60 min; T3, treated with charcoal for 4 h; TN, total nitrogen; TP, total phosphorus; OM, organic matter; GMC, gravimetric moisture content.
*: 0.001 < P < 0.05. Numbers in parentheses represent negative numbers.
Discussion
Fires significantly reduce microbial biomass in soil (Dooley and Treseder 2012; Pressler et al. 2018; Barreiro and Díaz-Raviña 2021), as was also demonstrated in this study. However, the extent of biomass reduction varied among microbial taxa and the effects of different heat treatments on microbial biomass were significantly distinct (Nelson et al. 2022). Notably, the most substantial decrease in total microbial biomass was observed in treatment T2. In terms of individual microbial taxa, bacteria exhibited changes consistent with the total microbial biomass, whereas fungi and actinomycetes experienced the most significant decreases in biomass in T3. Given that treatment T2 reached the highest peak temperature and treatment T3 involved the longest duration of heat exposure, it can be inferred that bacteria are susceptible to high temperatures, whereas fungi and actinomycetes are more sensitive to prolonged heat exposure.
This study has demonstrated that fire intensity had significantly different effects on the microorganisms in different soil layers. Overall, the total microbial biomass in the topsoil layer was reduced by 1.84 × 106 (96.80%) while it decreased by 3.76 × 105 in the subsoil layer (48.39%), approximately half the decrease observed in the topsoil layer. These findings align with prior research indicating that fires predominantly impact the surface soil ecosystem negatively, with this adverse effect diminishing with increased soil depth. The difference in fire disturbances impact between soil layers also corresponds with temperature gradient along the soil profile (DeBano 2000). For example, the maximum temperature reached in treatment T1 was 385°C at 2 cm depth and only 29.6°C at 10 cm depth. In the T2 treatment, the peak temperature was 639°C at 2 cm and just 56.7 at 10 cm. In the T3 treatment, the highest temperature was 348°C at 2 cm and 45.5°C at 10 cm. Furthermore, the impact of different fire conditions varied across soil layers. In the topsoil layer, the most significant reduction in the total microbial biomass occurred in the T3 treatment, with the least reduction in the T1 treatment. In the subsoil layer, the greatest decrease was observed in the T2 treatment, and the smallest in the T1 treatment. This indicates that the duration of fire exposure is a crucial factor affecting microorganisms in the topsoil, whereas microorganisms in the subsoil are primarily impacted by high temperatures.
The impact of fire on microorganisms across different soil layers varied among the various types of microorganisms. The change trends in the biomass of bacteria, actinomyces and fungi in topsoil were universal, but in the subsoil layer, only bacterial biomass exhibited a significant decrease in all three experimental treatments. Conversely, fungal biomass increased in all three experimental treatments, and actinomyces biomass increased in the T1 and T2 treatments. Historically, it was widely assumed that fungi were highly susceptible to fire and struggle with post-fire recovery (Neary et al. 1999; Pingree and Kobziar 2019). However, stratification analysis in our study shows an increase in fungal biomass in the subsoil post-fire, likely associated with the sharp drop in temperature along the soil profile. Consequently, future studies on the effects of fire on microorganisms should include: (1) exploring variations among different soil profiles; (2) acknowledging that temperature gradients may facilitate microorganism recovery in specific soil layers; and (3) considering actinomycetes, which have been largely overlooked in fire research despite their critical ecological roles (such as the diversity of their metabolites) (Demain 2014; Chandra et al. 2020; Sayed et al. 2020).
Correlation analysis showed that heat (the cumulative effect of heating) was significantly negatively correlated with the biomass of microorganisms in the three treatments post-fire, whereas there was no significant correlation with peak temperature. This phenomenon has also been reported by other studies (Lombao et al. 2020, 2021). Peak temperature and the duration of heat are important factors of a fire (Barreiro et al. 2020), with peak temperature influencing the temperature gradient across the soil profile, and the lethality of different temperatures dependent on the duration of warming (Pingree and Kobziar 2019). Heat, as a parameter that encapsulates both peak temperature and heat duration of fire, emerges as an important descriptor of fire and should be emphasised in future fire studies (Lombao et al. 2015, 2020).
Although the post-fire microbial biomass varied greatly among the experimental treatments, all these treatments recovered to control levels for the first time by Day 20 post-fire, a recovery timeline that is quicker than previously reported (Villar et al. 2004; Dangi et al. 2010; Xiang et al. 2014; Olejniczak et al. 2019). There were also differences in post-fire recovery patterns between microbial taxa and soil layers. Bacterial counts post-fire remained at 5.88 × 104 in the topsoil, and 3.28 × 105 in the subsoil, with both layers achieving control levels by Day 20 post-fire. Despite the complete removal of fungi in the topsoil layer post-fire, they also reached control levels by Day 20 post-fire. This may potentially be due to the upward migration of microorganisms from the subsoil layer (Gongalsky et al. 2012; Maurin et al. 2014; Olejniczak et al. 2019; Certini et al. 2021). In this context, She et al. (2020) suggested that nematode-trapping fungi (NTF) in the subsoil layer (10–20 cm) might rapidly migrate upward post-fire, thus facilitating the swift recovery of the NTF community in the topsoil layer (0–10 cm). The observed increase in microbial biomass in the subsoil layer post-fire is also likely to contribute to the quick restoration of microbial biomass in the topsoil layer (Bollen 1969). The recovery pattern of actinomycetes in treatments T1 and T2 mirrored that of fungi, but differed in treatment T3. While actinomycetes were not entirely eradicated from the topsoil layer, and their biomass in the subsoil layer increased post-fire, it did not reach control levels until Day 50. Considering the diverse metabolites of actinomycetes and their slower recovery post-fire compared to bacteria and fungi, this taxon warrants increased focus in the restoration of soil ecosystems.
In this study, we also continuously monitored changes in the physicochemical properties of the soil post-fire and analysed their correlation with microbial biomass. The findings revealed that physicochemical properties were correlated with the biomass of specific microbial taxa in certain treatments only. This indicates that the influence of physicochemical factors on the short-term recovery process post-fire is not yet evident.
Conclusions
This study draws three main conclusions. (1) The impact of fire on microbial biomass in the soil was profound, with the most significant reduction observed in bacteria, followed by fungi, and the least affected were actinomycetes (85.00, 29.66, and 11.45%, respectively). (2) The impact of fire disturbance varied between soil layers; notably, microbial biomass diminished by 96.80% in the topsoil layer (0–10 cm), a decrease approximately twice as large as that in the subsoil layer (10–20 cm). (3) Heat input was correlated with post-fire changes in microbial biomass and its recovery, as demonstrated by correlation analysis (P < 0.05). These findings suggest that the rapid recovery of soil microbial biomass post-fire surpasses prior expectations, facilitated by the swift decline in heat and peak temperature generated by the fire along the soil profile. Consequently, the negative effects on soil microbial biomass were limited to the soil surface (0–10 cm), while the effects on subsurface microbial biomass were positive. Subsoil (10–20 cm) microorganisms are the key factors driving the rapid recovery of soil microorganisms post-fire, necessitating future studies to focus on their distribution and function, particularly actinomycetes and fungal groups.
Data availability
The data that support this study will be shared on reasonable request to the corresponding author.
Declaration of funding
This research was funded by National Natural Science Foundation of China, Grant No. 32360002, the Second Tibetan Plateau Scientific Expedition and Research Program (STEP), Grant No. 2019QZKK0402, and Yunnan Intelligence Union Program, Grant No. 202203AM140009.
References
Agbeshie AA, Abugre S, Atta-Darkwa T, Awuah R (2022) A review of the effects of forest fire on soil properties. Journal of Forestry Research 33, 1419-1441.
| Crossref | Google Scholar |
Averill C, Anthony MA, Baldrian P, Finkbeiner F, van den Hoogen J, Kiers T, Kohout P, Hirt E, Smith GR, Crowther TW (2022) Defending Earth’s terrestrial microbiome. Nature Microbiology 7, 1717-1725.
| Crossref | Google Scholar | PubMed |
Barreiro A, Díaz-Raviña M (2021) Fire impacts on soil microorganisms: mass, activity, and diversity. Current Opinion in Environmental Science & Health 22, 100264.
| Crossref | Google Scholar |
Barreiro A, Lombao A, Martín A, Cancelo-González J, Carballas T, Díaz-Raviña M (2020) Soil heating at high temperatures and different water content: effects on the soil microorganisms. Geosciences 10(9), 355.
| Crossref | Google Scholar |
Ben-David A, Davidson CE (2014) Estimation method for serial dilution experiments. Journal of Microbiological Methods 107, 214-221.
| Crossref | Google Scholar | PubMed |
Bollen GJ (1969) The selective effect of heat treatment on the microflora of a greenhouse soil. Netherlands Journal of Plant Pathology 75, 157-163.
| Crossref | Google Scholar |
Certini G (2014) Fire as a soil-forming factor. Ambio 43, 191-195.
| Crossref | Google Scholar | PubMed |
Certini G, Moya D, Lucas-Borja ME, Mastrolonardo G (2021) The impact of fire on soil-dwelling biota: a review. Forest Ecology and Management 488, 118989.
| Crossref | Google Scholar |
Chandra P, Sharma RK, Arora DS (2020) Antioxidant compounds from microbial sources: a review. Food Research International 129, 108849.
| Crossref | Google Scholar | PubMed |
Coban O, De Deyn GB, van der Ploeg M (2022) Soil microbiota as game-changers in restoration of degraded lands. Science 375(6584), abe0725.
| Crossref | Google Scholar | PubMed |
Dangi SR, Stahl PD, Pendall E, Cleary MB, Buyer JS (2010) Recovery of soil microbial community structure in a wildfire impacted forest soil. Land Degradation & Development 21, 423-432.
| Crossref | Google Scholar |
DeBano LF (2000) The role of fire and soil heating on water repellency in wildland environments: a review. Journal of Hydrology 231-232, 195-206.
| Crossref | Google Scholar |
Demain AL (2014) Importance of microbial natural products and the need to revitalize their discovery. Journal of Industrial Microbiology & Biotechnology 41, 185-201.
| Crossref | Google Scholar | PubMed |
Devi NB, Yadava PS (2006) Seasonal dynamics in soil microbial biomass C, N and P in a mixed-oak forest ecosystem of Manipur, North-east India. Applied Soil Ecology 31, 220-227.
| Crossref | Google Scholar |
Dooley SR, Treseder KK (2012) The effect of fire on microbial biomass: a meta-analysis. Biogeochemistry 109, 49-61.
| Crossref | Google Scholar |
Dunn PH, Barro SC, Poth M (1985) Soil moisture affects survival of microorganisms in heated chaparral soil. Soil Biology and Biochemistry 17, 143-148.
| Crossref | Google Scholar |
Enninful EK, Torvi DA (2008) A variable property heat transfer model for predicting soil temperature profiles during simulated wildland fire conditions. International Journal of Wildland Fire 17, 205-213.
| Crossref | Google Scholar |
Fittipaldi M, Nocker A, Codony F (2012) Progress in understanding preferential detection of live cells using viability dyes in combination with DNA amplification. Journal of Microbiological Methods 91, 276-289.
| Crossref | Google Scholar | PubMed |
Gongalsky KB, Malmström A, Zaitsev AS, Shakhab SV, Bengtsson J, Persson T (2012) Do burned areas recover from inside? An experiment with soil fauna in a heterogeneous landscape. Applied Soil Ecology 59, 73-86.
| Crossref | Google Scholar |
Hart SC, DeLuca TH, Newman GS, MacKenzie MD, Boyle SI (2005) Post-fire vegetative dynamics as drivers of microbial community structure and function in forest soils. Forest Ecology and Management 220, 166-184.
| Crossref | Google Scholar |
He T, Lamont BB, Pausas JG (2019) Fire as a key driver of Earth’s biodiversity. Biological Reviews 94, 1983-2010.
| Crossref | Google Scholar | PubMed |
Holden SR, Treseder KK (2013) A meta-analysis of soil microbial biomass responses to forest disturbances. Frontiers in Microbiology 4, 163.
| Crossref | Google Scholar | PubMed |
Jones GM, Tingley MW (2021) Pyrodiversity and biodiversity: a history, synthesis, and outlook. Diversity and Distributions 28, 386-403.
| Crossref | Google Scholar |
Keeley JE (2009) Fire intensity, fire severity and burn severity: a brief review and suggested usage. International Journal of Wildland Fire 18, 116-126.
| Crossref | Google Scholar |
Lazarina M, Devalez J, Neokosmidis L, Sgardelis SP, Kallimanis AS, Tscheulin T, Tsalkatis P, Kourtidou M, Mizerakis V, Nakas G, Palaiologou P, Kalabokidis K, Vujic A, Petanidou T (2019) Moderate fire severity is best for the diversity of most of the pollinator guilds in Mediterranean pine forests. Ecology 100, e02615.
| Crossref | Google Scholar | PubMed |
Leverkus AB, Murillo PG, Doña VJ, Pausas JG (2019) Wildfires: opportunity for restoration? Science 363, 134-135.
| Crossref | Google Scholar | PubMed |
Lombao A, Barreiro A, Cancelo-González J, Martín Á, Díaz-Raviña M (2015) Impact of thermal shock on forest soils affected by fires of different severity and recurrence. Spanish Journal of Soil Science 5, 165-179.
| Crossref | Google Scholar |
Lombao A, Barreiro A, Fontúrbel MT, Martín A, Carballas T, Díaz-Raviña M (2020) Key factors controlling microbial community responses after a fire: importance of severity and recurrence. Science of the Total Environment 741, 140363.
| Crossref | Google Scholar | PubMed |
Lombao A, Barreiro A, Fontúrbel MT, Martín A, Carballas T, Díaz-Raviña M (2021) Effect of repeated soil heating at different temperatures on microbial activity in two burned soils. Science of The Total Environment 799, 149440.
| Crossref | Google Scholar | PubMed |
Lucas-Borja ME, Miralles I, Ortega R, Plaza-Álvarez PA, Gonzalez-Romero J, Sagra J, Soriano-Rodríguez M, Certini G, Moya D, Heras J (2019) Immediate fire-induced changes in soil microbial community composition in an outdoor experimental controlled system. Science of the Total Environment 696, 134033.
| Crossref | Google Scholar | PubMed |
Mataix-Solera J, Cerdà A, Arcenegui V, Jordán A, Zavala LM (2011) Fire effects on soil aggregation: a review. Earth-Science Reviews 109, 44-60.
| Crossref | Google Scholar |
Maurin O, Davies TJ, Burrows JE, Daru BH, Yessoufou K, Muasya AM, van der Bank M, Bond WJ (2014) Savanna fire and the origins of the ‘underground forests’ of Africa. New Phytologist 204, 201-214.
| Crossref | Google Scholar | PubMed |
Naimoddin Mirza A, Sudhakarrao Patil S (2022) Mapping of microbial diversity of Gautala Reserve Forest in Aurangabad (District) (M.S.), India. Applied Ecology and Environmental Sciences 10, 303-310.
| Crossref | Google Scholar |
Neary DG, Klopatek CC, DeBano LF, Ffolliott PF (1999) Fire effects on belowground sustainability: a review and synthesis. Forest Ecology and Management 122, 51-71.
| Crossref | Google Scholar |
Nelson AR, Narrowe AB, Rhoades CC, Fegel TS, Daly RA, Roth HK, Chu RK, Amundson KK, Young RB, Steindorff AS, Mondo SJ, Grigoriev IV, Salamov A, Borch T, Wilkins MJ (2022) Wildfire-dependent changes in soil microbiome diversity and function. Nature Microbiology 7, 1419-1430.
| Crossref | Google Scholar | PubMed |
Olejniczak I, Górska EB, Prędecka A, Hewelke E, Gozdowski D, Korc M, Panek E, Tyburski Ł, Skawińska M, Oktaba I, Boniecki P, Kondras M,Oktaba L (2019) Selected biological properties of the soil in a burnt-out area under old pine trees three years after an fire. Rocznik Ochrona Srodowiska 21, 1279-1293.
| Google Scholar |
Owen SM, Patterson AM, Gehring CA, Sieg CH, Baggett LS, Fulé PZ (2019) Large, high-severity burn patches limit fungal recovery 13 years after wildfire in a ponderosa pine forest. Soil Biology and Biochemistry 139, 107616.
| Crossref | Google Scholar |
Pausas JG, Ribeiro E (2017) Fire and plant diversity at the global scale. Global Ecology and Biogeography 26, 889-897.
| Crossref | Google Scholar |
Pingree MRA, Kobziar LN (2019) The myth of the biological threshold: a review of biological responses to soil heating associated with wildland fire. Forest Ecology and Management 432, 1022-1029.
| Crossref | Google Scholar |
Pressler Y, Moore JC, Cotrufo MF (2018) Belowground community responses to fire: meta-analysis reveals contrasting responses of soil microorganisms and mesofauna. Oikos 128, 309-327.
| Crossref | Google Scholar |
Rawat M, Arunachalam K, Arunachalam A (2021) Seasonal dynamics in soil microbial biomass C, N and P in a temperate forest ecosystem of Uttarakhand, India. Tropical Ecology 62, 377-385.
| Crossref | Google Scholar |
Sayed AM, Hassan MHA, Alhadrami HA, Hassan HM, Goodfellow M, Rateb ME (2020) Extreme environments: microbiology leading to specialized metabolites. Journal of Applied Microbiology 128, 630-657.
| Crossref | Google Scholar | PubMed |
She R, Zhou XJ, Wang HQ, Zhang F, Yang XY, Xiao W (2020) Succession of soil nematode-trapping fungi following fire disturbance in forest. Journal of Forest Research 25, 433-438.
| Crossref | Google Scholar |
She R, Zhou XJ, Wang HQ, Zhang F, Yang XY, Xiao W (2022) Natural recovery from fire disturbance is more favorable than assisted recovery for the restoration of soil nematode-trapping fungi. Canadian Journal of Microbiology 68, 329-339.
| Crossref | Google Scholar | PubMed |
Sultana S, Chowdhury A, Sultana T, Alam K, Khan RA (2021) Physicochemical and microbiological evaluation of surface water quality of aquaculture ponds located in Savar, Dhaka, Bangladesh. GSC Advanced Research and Reviews 9, 138-146.
| Crossref | Google Scholar |
van der Bij AU, Weijters MJ, Bobbink R, Harris JA, Pawlett M, Ritz K, Benetková P, Moradi J, Frouz J, van Diggelen R (2018) Facilitating ecosystem assembly: plant-soil interactions as a restoration tool. Biological Conservation 220, 272-279.
| Crossref | Google Scholar |
van der Heijden MGA, Bardgett RD, Van Straalen NM (2008) The unseen majority: soil microbes as drivers of plant diversity and productivity in terrestrial ecosystems. Ecology Letters 11, 296-310.
| Crossref | Google Scholar | PubMed |
Villar MC, Petrikova V, Dı’az-Raviña M, Carballas T (2004) Changes in soil microbial biomass and aggregate stability following burning and soil rehabilitation. Geoderma 122, 73-82.
| Crossref | Google Scholar |
Wagg C, Bender SF, Widmer F, Van Der Heijden MGA (2014) Soil biodiversity and soil community composition determine ecosystem multifunctionality. Proceedings of the National Academy of Sciences 111, 5266-5270.
| Crossref | Google Scholar | PubMed |
Xiang X, Shi Y, Yang J, Kong J, Lin X, Zhang H, Zeng J, Chu H (2014) Rapid recovery of soil bacterial communities after wildfire in a Chinese boreal forest. Scientific Reports 4, 3829.
| Crossref | Google Scholar | PubMed |