Effects of arsenite and dimethylarsenic on the growth and health of hydroponically grown commercial Doongara rice
Hayden P. Martin A , William A. Maher

A
B
Abstract
Arsenic’s effect on rice plant health is a critical environmental issue. This study reveals that rice plants absorb inorganic arsenic and dimethylarsenic differently, with dimethylarsenic posing a greater threat to rice plant health. These findings contribute to our understanding of arsenic toxicity in plants, highlighting the need for further research into detoxification strategies for dimethylarsenic.
Arsenic toxicity in plants, particularly the effects of different arsenic species, is not well understood. This study investigated the response of juvenile rice plants, grown hydroponically, to prolonged exposure to inorganic and dimethyl arsenic species. The hydroponic system removed complexity by eliminating soil processes.
The accumulation of inorganic As (Asi) and dimethylarsenic (DMA) in hydroponically grown rice was monitored for plants exposed to different As concentrations (0–6.7 µmol L−1). Dose–response experiments were conducted to compare the effects of As species on plant health in terms of growth.
Plants absorb Asi and DMA linearly, with faster Asi uptake than DMA. Asi exposure leads to higher As concentrations in roots and shoots than DMA. Despite more Asi in roots, its translocation to shoots is lower. Asi and DMA accumulation in shoots remains relatively constant at lower As concentrations. At the highest As concentration, more Asi and DMA accumulate in shoots. Exceeding 1.6 µmol L−1, Asi and DMA reduce plant height and biomass. Asi-exposed plants show little health differences except at the highest concentrations. DMA-exposed plants show more unhealthy instances above 1.6 µmol L−1.
DMA’s lower uptake rate aligns with other rice species results, as do lower shoot and root translocation factors. Near constant As concentrations in shoots at low Asi concentrations suggest an Asi exposure threshold before plants lose their As sequestration ability, resulting in reduced growth. DMA exposure increases the number of unhealthy plants, suggesting a greater potential effect on plant health and fitness, differing from Asi-induced stress.
Keywords: arsenic species, dimethylarsinic acid, health effects, hydroponics, rice, rice grain, straight head disease, uptake.
Introduction
Rice is a stable food source for 3.5 billion people (https://www.cgiar.org/research/center/irri/). Arsenic (As) is accumulated in rice from natural sources, e.g. soils and rocks (Palmer et al. 2021) and the historical use of herbicides such as cacodylic acid (Limmer and Seyfferth 2020). There is a concern about human exposure to As, particularly for infants, through the consumption of rice and processed rice products (Sohn 2014; Maher et al. 2018).
Arsenic is phytotoxic to plants at high concentrations (Tang et al. 2016a, 2016b) and can result in lower plant growth, crop yields and seed germination (Murugaiyan et al. 2021). High As concentrations are also associated with straight-head disease (Hua et al. 2013). This disease is a physiological disorder in rice with symptoms of sterile spikelets, distorted husks and erect panicles (Tang et al. 2020) and results in yield losses of up to 90% (Rahman et al. 2008). Two As species are commonly found in most rice species, inorganic arsenic (Asi), arsenite (AsIII) and arsenate (AsV), and dimethylarsenic (DMA) (Maher et al. 2018). Asi is transported to roots by phosphate transporters and through two silicon transporters, Lsi1 (the aquaporin NIP2;1) and Lsi2 (an efflux carrier) (Bienert et al. 2008; Li et al. 2009; Abedi and Mojiri 2020). AsV is mostly reduced to AsIII in flooded organic-rich paddy fields (Takahashi et al. 2004; Xu XY et al. 2008) and within rice plants, effluxed or complexed with phytochelatins (PC) and AsIII–PC complexes sequestered within vacuoles (Duan et al. 2011; Lemos Batista et al. 2014). DMA is produced by bacterial methylation of Asi in reduced organic-rich soils (Jia et al. 2013; Chen C et al. 2019; Geng et al. 2023); rice plants cannot methylate As (Ye et al. 2012). DMA also enters roots by a silicon transporter, Lsi1 (Li et al. 2009; Abedi and Mojiri 2020). DMA uptake into roots is lower than Asi; however, unlike Asi, DMA is efficiently transported to reproductive organs (Zheng et al. 2013). DMA is in a dissociated state (Sarwar et al. 2021), thus it cannot form PC complexes and poorly interacts with SH groups (Abedin et al. 2002). This results in higher DMA concentrations in grain compared to that of Asi (Abedin et al. 2002; Shinde and Kumar 2021). DMA is also more toxic to rice (Zheng et al. 2013; Tang et al. 2016a). When this study was undertaken, DMA was suspected of causing straighthead disease, but little direct evidence was available.
Soil properties (pH, REDOX potential, organic matter, S, N, P, Fe, and Mn content) affect the uptake of As by rice plants (Zhao et al. 2009; Abedi and Mojiri 2020), thus we conducted hydroponic experiments to eliminate the complexity associated with soils. We used a commercial rice species, Doongara, that is susceptible to straighthead disease (Martin et al. 2023) to understand the connection between As and straighthead disease. The aims of the study were to: (a) measure how the uptake and accumulation of As in Doongara varies when exposed to different AsIII and DMA concentrations and (b) measure how exposure to AsIII and DMA affects developing Doongara plants in terms of their growth and health.
Experimental
Hydroponic system
The system was a self-contained reservoir, with individual plants growing in individual reservoirs (Fig. 1). The system consisted of three main components: a reservoir, plant housing and aerating system. The reservoir was constructed using 90-mm diameter polyvinyl chloride (PVC) pipes cut to 370-mm lengths. Along the edge, five holes (22-mm diameter) were drilled 55 mm apart. The five large holes were used to insert the plant housing units. A sixth hole was also bored, with a diameter of 10 mm. The smaller hole was used to insert an air hose to aerate the nutrient solution and as an access point to take pH measurements. The capacity of the system was 1.6 L per reservoir.
Hydroponics set up. (a) The complete system with growing rice plants. (b) An example of the plant housing tube with a rice plant placed in the styrofoam disc.
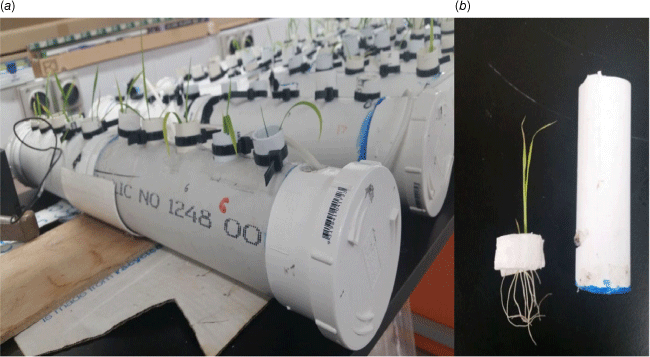
The plant housing units were constructed using 20-mm diameter piping cut to 80 mm in length to fit into the reservoirs. Each housing unit held a single plant, and the spacing between the plants allowed the plant to grow without the roots becoming entangled with neighbouring plants. In previous hydroponic systems, it has been found that if the plants were not separated, direct competition occurred, leading to roots becoming entangled, resulting in significant experimental variation within treatments. Plants were held in place using styrofoam discs. Styrofoam was used due to its inert properties, thus avoiding the leaching of unwanted contaminants into the nutrient solution. Each nutrient solution and treatment was aerated by pumping compressed air through Teflon tubing and bubbling it into the nutrient solution by air stones through the sixth hole.
The plants were grown in a temperature-controlled laboratory between 20 and 24°C with two ATI T5 power module light systems using T5 full-spectrum fluorescent bulbs suspended from the roof above the plants. The light field was measured using an irradiance sensor (Biospherical Instruments QSL 2102) across the surface of the hydroponic growing tubes to ensure an even light distribution across the surface.
Seed germination and growth conditions
Rice seeds were surface sterilised utilising a technique adapted from Kim et al. (2005). The seeds were sterilised by rinsing with 10% v/v H2O2 for 10 min, followed by 70% w/w ethanol for 5 min and a final rinse with deionised water. After surface sterilisation, the seeds were placed in deionised water and then placed in an incubator at 30°C for 48 h to break dormancy and promote germination of the seedlings.
Seedlings were transplanted into the hydroponic system after germination. For the first 14 days following germination, the plants were exposed to a half-strength nutrient treatment to allow the plants to acclimate, followed by full strength. The nutrient media composition was as follows: 396 μmol L−1 of KNO3, 360 μmol L−1 of Ca(Cl)2, 290 μmol L−1 of MgSO4·H2O, 38 μmol L−1 of NaH2PO42H2O, 230 μmol L−1 of K2SO4, 2.5 μmol L−1 of MnCl2·4H2O, 3.2 μmol L−1 of H3BO3, 0.04 μmol L−1 of (NH4)6Mo7O24·4H2O, 0.035 μmol L−1 of ZnSO4·7H2O, 0.04 μmol L−1 of CuSO4·5H2O, 0.037 μmol L−1 of FeCl3·6H2O and 0.04 μmol L−1 of Na2SiO2·5H2O. The pH of the nutrient solution was 5.5. The nutrient solution was similar to that used by Yoshida et al. (1976), with the addition of silicon at 0.04 μmol L−1 and EDTA at 3.4 μmol L−1. The ETDA was added to prevent the precipitation of iron oxyhydroxide and plaque formation on roots (Jacobson 1951). The nutrient solution and As species were renewed every 7 days. This involved discarding the old nutrient solution and cleaning the tubes to ensure no build-up of mould or algae. The tubes were then topped up with 1.6 L of nutrient solution and appropriate arsenic species. Plants were grown on a 14:10-h day:night cycle at a light intensity of 180 µE m−2 s−1.
Experimental design
Two hydroponic growth experiments were conducted with Doongara, a long-grain rice variety that was selected due to its susceptibility to As and straighthead disease (Martin et al. 2023). The rice plants were exposed to AsIII and DMA at four different concentrations: 0.0, 0.8, 1.6, 3.5 and 6.7 µmol L−1. The arsenic concentrations selected were based on the study by Shaibur et al. (2006), where Asi toxicity in hydroponically cultivated rice plants was found to be severe above 6.7 µmol L−1 of AsIII. No experiments have reported direct toxicity for DMA in rice, so for this study, the DMA concentrations were matched to that of AsIII to allow comparison between the two As species. For quality control, the highest arsenic treatment (6.7 µmol L−1) of the alternative arsenic species was included in the AsIII and DMA experiments.
Treatments were carried out in triplicate for a total of 38 days to allow for substantial growth to occur and to observe As accumulation throughout the vegetative growth stage. The experiment was terminated before the plants reached flowering, thus allowing us to observe the early accumulation of As because studies have shown that the uptake of nutrients and As changes at different growth stages for rice (Zheng et al. 2011).
Harvested plants were lightly rinsed with deionised water, and height was recorded from the base of the stem to the tip of the highest leaf. Plant biomass was determined from plant dry mass. Plant roots were also inspected for iron plaque formation; however, no plaque was observed on any of the root systems (Fig. 2).
Photos of rice plants being processed at the end of the experiments. (a) A rice plant from the hydroponic experiments. Note the white root structure. (b) Plants grown in the field (Martin et al. 2023) – the top trays hold the above-ground biomass, and the bottom trays are the roots of the plant that show iron plaque (Chen Z et al. 2005). Panel (a) illustrates that the rice grown hydroponically exhibited no iron plaque formation (typically an orange colour) on the roots.
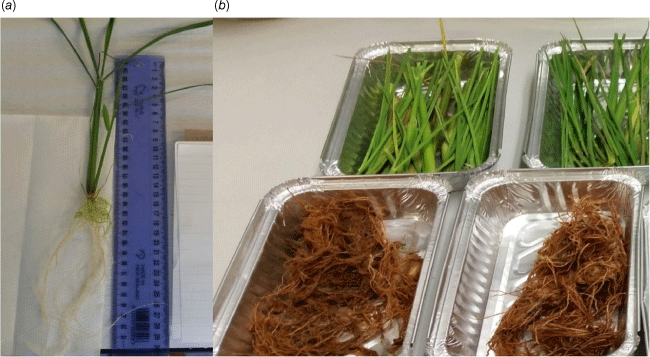
Arsenic measurement
Plant samples were digested in a microwave oven (CEM, MARS) with 2 mL of concentrated HNO3 and 1 mL of H2O2 (30% v/v). Samples were digested in batches of 40, containing 37 samples, 2 certified reference materials (CRMs) and 1 blank. Digests were diluted to 10 mL with deionised water. Before analyses, digests were further diluted to 1 in 100 (v/v) with deionised water and internal standards added before analysis by inductively coupled plasma–mass spectrometry (ICP-MS) (Perkin Elmer DRC II) (Maher et al. 2013).
Plant samples were extracted with 2% v/v HNO3 and diluted to 10 mL with deionised water. The extracts were centrifuged for 10 min at 4000 rpm (Eppendorf, 5804R) at room temperature and filtered through 0.45-μm polyethersulfone (PES) syringe filters before analysis. Samples were analysed using high-performance liquid chromatography (HPLC)-ICP-MS (Perkin Elmer) employing a PRPX-100 anion exchange column (Hamilton) with a mobile phase containing 20 mM (NH4)3PO4 buffer at a flow rate of 1.5 mL min−1 and a column temperature of 40°C (Maher et al. (2013). Results below are reported as Asi (the sum of AsIII and AsV). Nearly all the Asi is AsIII.
The measured total As concentrations in reference materials (NIES 10a Rice Flour and NIES SRM 1568a) and As speciation concentrations (NIST 1568a Rice Flour) were in agreement with published concentrations (Table 1).
Total arsenic and arsenic speciation | Measured (μg g−1) | Certified (μg g−1) | |
---|---|---|---|
Total As concentrations in certified reference material | |||
NIES 10a Rice Flour | 0.18 ± 0.03 (n = 11) | 0.17 | |
NIST 1568a Rice Flour | 0.279 ± 0.001 (n = 10) | 0.29 ± 0.03 | |
Arsenic species in NIST 1568a Rice Flour | |||
AsIII | 0.088 ± 0.015 (n = 15) | 0.062 ± 0.009 | |
DMA | 0.157 ± 0.022 (n = 15) | 0.163 ± 0.009 | |
AsV | 0.034 ± 0.013 (n = 15) | 0.039 ± 0.005 | |
MA | 0.010 ± 0.002 (n = 15) | 0.010 ± 0.003 |
Results and discussion
Arsenic accumulation
Plants exposed to Asi accumulated more As as exposure concentrations increased, and the amount of As accumulated was much higher than in plants exposed to a comparable concentration of DMA (Fig. 3). Because plants were grown for the same period of time, 38 days, accumulated As amounts are directly comparable. When the plant was exposed to either As species, the majority of the As was in the roots. Specifically, 92 ± 5% of Asi and 52 ± 15% of DMA were found in the roots. Plants exposed to Asi or DMA both showed a linear response to increasing As concentration. The response to increasing As exposure, however, showed two distinct accumulation patterns. The roots of the rice plants accumulated Asi to much higher concentrations than the shoots, whereas plants exposed to DMA had a similar distribution of As between shoots and roots with increasing As concentration (Fig. 3). The differences in uptake observed between As species are in agreement with previously published data (Abedin et al. 2002).
Arsenic accumulation within different plant segments of rice grown hydroponically with increasing exposure to Asi (a) and DMA (b). Linear regressions were fitted, as shown by dotted lines, with 95% confidence intervals represented by the shaded areas.
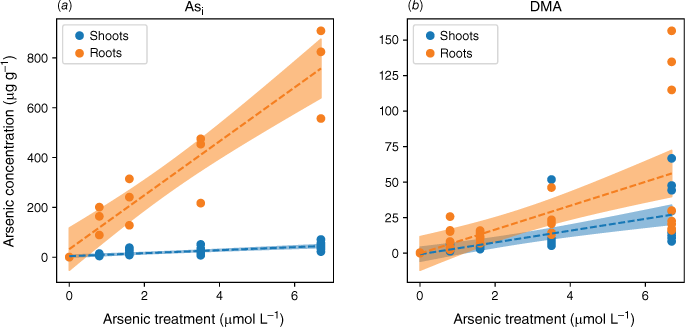
Plants exposed to Asi contained up to 13–19 fold higher As concentrations in the roots and 1.6–4 fold higher As concentrations in the shoots than when exposed to DMA. The lower uptake rate of DMA is in accordance with results published for other rice species (Abedin et al. 2002). Visual observations at the end of the experiment show that iron plaque did not form on the roots (Fig. 2). In the absence of iron plaque, the high As concentrations measured in the roots for the Asi treatments suggest active sequestration by root cells or As absorption followed by incorporation into root cells (Vázquez Reina et al. 2005; Moore et al. 2011).
Although root cells took up more Asi, the translocation of As from roots to shoots was significantly lower for Asi than DMA. The ratios of shoot to root As concentrations were 0.06–0.08 and 0.3–0.6 for Asi and DMA respectively. The accumulation of As in the shoots of the plants exposed to Asi remained fairly constant at low Asi concentrations (0.8–3.5 µmol L−1) (Fig. 4). It was only through exposure to the highest Asi treatment (6.7 µmol L−1) that the As concentration significantly increased within shoots (P < 0.05). The As concentrations in the shoots increased from 21 ± 14 to 45 ± 14 µg g−1 for the 3.5 to 6.7 µmol L−1 treatments, whereas for the lower Asi treatments (0.8 and 1.6 µmol L−1), mean As concentrations were 11 ± 3 and 21 ± 14 µg g−1 respectively. This poses the question, is there a threshold for Asi exposure before plants lose the ability to sequester As in roots (Hartley-Whitaker et al. 2001)?
Mean arsenic concentrations throughout the rice plant for plants exposed to Asi (a) and DMA (b). Error bars represent 1 s.d.
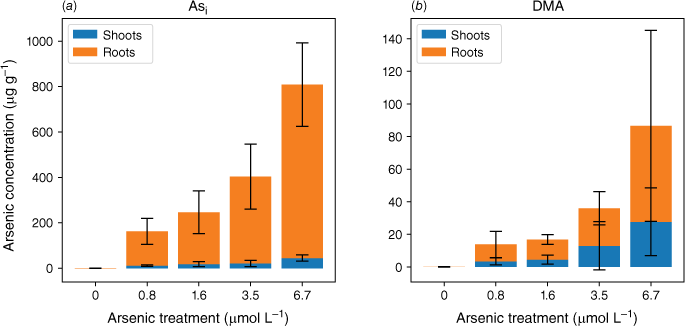
As previously described, detoxification of Asi involves either the sequestration of As by the formation of AsIII–thiol complexes with glutathione (GSH) or phytochelatins (PCs) (Raab et al. 2005) in vacuoles (Song et al. 2010, 2014) or As being pumped out of cells into the external medium via efflux pathways (Xu J et al. 2017). An efficient Asi efflux transporter within rice plants has not yet been identified; however, Lsi1 has been identified as a bi-directional transporter that can efflux a small amount of AsIII (Zhao et al. 2010). Sequestration of Asi in the roots is an effective way to limit the transport of Asi to above-ground tissues, although Asi still has some mobility throughout the plant (Zhao et al. 2012). Unlike Lsi1, Lsi2 can control the efflux of AsIII towards the stele and restrict xylem loading (Ma et al. 2008). Further transport is limited by additional vascular sequestration (Chen Y et al. 2015). These plant nodes play a critical role in storing Asi and controlling further Asi distribution (Yamaji and Ma 2014; Zhao et al. 2014; Chen Y et al. 2015).
In plants exposed to DMA, the As concentrations in the roots and shoots are fairly consistent across all As exposures (as shown in Fig. 3 and 4). Specifically, the roots contain 10–23 µg g−1, and the shoots contain 3–13 µg g−1 for a DMA treatment concentration range from 0.8 to 3.5 µmol L−1. Approximately half of the arsenic is translocated from the roots to the shoots. Similar to the highest Asi concentration tested, significantly greater amounts of DMA (28 µg g−1) are accumulated in shoots. Our current understanding is that rice plants lack the ability to either efflux or sequester DMA into vacuoles to reduce its mobility within the plant. Mishra et al. (2017) exposed rice plants to AsV, monomethyl arsenic (MA) and DMA for 7 days, and DMA had the lowest shoot and root translocation factors, and no DMA–PC complexes were detected (Raab et al. 2007a). DMA, however, was efficiently translocated between the roots and shoots (Raab et al. 2007b) by both xylem and phloem (Carey et al. 2010), with phloem transport believed to be the main pathway for As transport to the grain (Carey et al. 2010; Zhao et al. 2012; Kumarathilaka et al. 2018).
A peptide transporter (OsPRT7) has been identified as being potentially involved in the translocation of DMA (Tang et al. 2017). Peptide transporters play an essential role in the transport and remobilisation of nitrogen throughout the plant (Tsay et al. 2007) and can affect germination, plant growth and grain yield (Fang et al. 2013, 2017). If DMA is transported by peptide transporters, this can offer a plausible mechanism for the translocation of DMA within rice plants (Tang et al. 2017). During growth and nitrogen utilisation, DMA could continually accumulate to high concentrations throughout the plant, leading to DMA stress and, in turn, straighthead in rice plants.
Plant growth
Plants exposed to Asi showed a significant decrease in plant height (F(5,47) = 13.04, P = 5.65 × 10−8) over the As concentration range tested. At Asi concentrations below 1.6 µmol L−1, plant height was not affected (Fig. 5); however, when Asi concentrations exceeded this value, a significant decrease in plant height occurred (1.6 µmol L−1 = 155 ± 84 mm, 3.5 µmol L−1 = 61 ± 23 mm, 6.7 µmol L−1 = 95 ± 31) when compared to the control, (230 ± 73 mm). For the plants exposed to DMA, there was no significant change in plant height across treatments (F(5,46) = 2.37, P = 0.053); however, when DMA concentrations exceeded 1.6 µmol L−1, the mean plant heights decreased (Fig. 5).
Plant height at the completion of the growth experiments. Error bars represent 1 standard deviation, n = 45 (Asi), n = 44 (DMA).
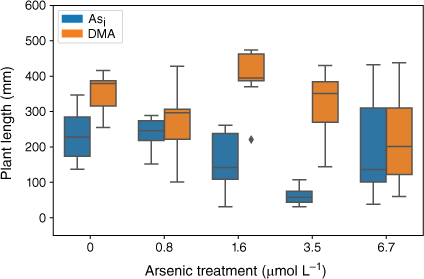
The effect of exposure to Asi and DMA on rice plant biomass showed a similar trend to that observed for plant heights (Fig. 6). Plants exposed to Asi showed a significant decrease (P < 0.05) in plant mass when exposed to increasing Asi concentrations (F(5,84) = 11.04, P = 3.43 × 10−8). Like plant heights, exposure to the lower Asi concentrations resulted in no significant decrease in mean plant biomass, whereas the higher Asi exposures showed a significant decrease in plant biomass relative to controls.
Plant dry mass at the completion of the growth experiments Error bars represent 1 standard deviation, n = 45 (Asi), n = 44 (DMA).
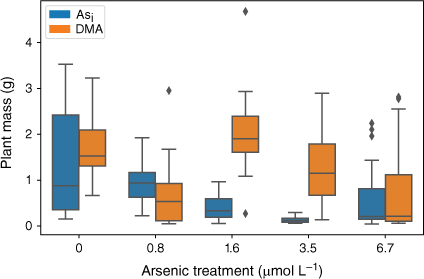
Plants exposed to DMA also showed a significant decrease in plant mass when exposed to increasing DMA concentrations (F(5,66) = 3.133, P = 0.0134). Again, when DMA concentrations exceeded 1.6 µmol L−1, the mean plant biomass decreased (Fig. 6).
To determine if exposure to the As species had any effect on plant health, the final plant heights were used as a measure of plant fitness. Both modelled dose–response curves and Z scores were used to investigate the effects on plant health (see Eqn 1):
where X̅ and s respectively represent the mean and standard deviation (s.d.) of the control group for each experiment, and X is the individual value. Z scores were used to normalise plant height to the control in each experiment. The percentage of plants that fell below the Z-scores for each treatment were deemed to be unhealthy (Table 2).
Arsenic species | Treatment (µmol L−1) | Percentage of unhealthy plants (−2.0 Z-score) | |
---|---|---|---|
AsIII | 0 | 0 | |
0.8 | 0 | ||
1.6 | 22 | ||
3.5 | 89 | ||
6.7 | 22 | ||
Reference (DMA, 6.7) | 25 | ||
DMA | 0 | 11 | |
0.8 | 38 | ||
1.6 | 11 | ||
3.5 | 22 | ||
6.7 | 25 | ||
Reference (AsIII, 6.7) | 44 |
Plants are deemed unhealthy when the height falls below a Z-score of 2.
Plant height significantly decreased with increasing Asi dosage with r2 = 0.89 when a second-order polynomial was fitted (Fig. 7). The DMA treatments displayed no significant change with increasing DMA concentration, r2 = 0.21, when a second-order polynomial was fitted (Fig. 7). A 10% reduction in plant height (EC10) is predicted when plants are exposed to 0.7 mmol L−1 Asi, and a 50% reduction in height (EC50) is predicted for Asi concentrations above 2.5 mmol L−1 Asi (Fig. 7). The rice plants treated with DMA demonstrated no overall consistent reduction in height with increasing DMA exposure (Fig. 7); however, due to the high variability within each DMA concentration treatment, it was not possible to calculate meaningful EC10 and EC50 results.
Dose–response curve for rice plants treated with Asi (blue) and DMA (orange). Plant height (%) relative to the control v. arsenic concentration. Error bars represent 1 standard deviation, n = 45 (Asi), n = 44 (DMA).
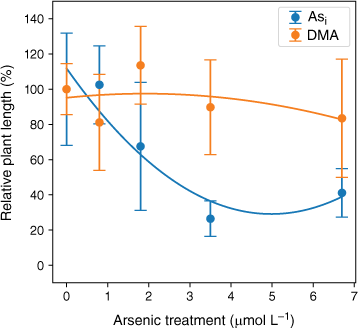
Plants exposed to an Asi concentration greater than 1.6 µmol L−1 showed an increasing number of unhealthy plants (Fig. 8). Plants exposed to DMA had a consistent number of unhealthy plants across all DMA exposures (Fig. 8) with a greater number of unhealthy plants at the higher exposure concentrations that contain higher As concentrations.
Arsenic concentration in the shoots of healthy and unhealthy rice plants exposed to (a) Asi (n = 45) and (b) DMA (n = 44). Divided by healthy (blue) and unhealthy (orange) plants.
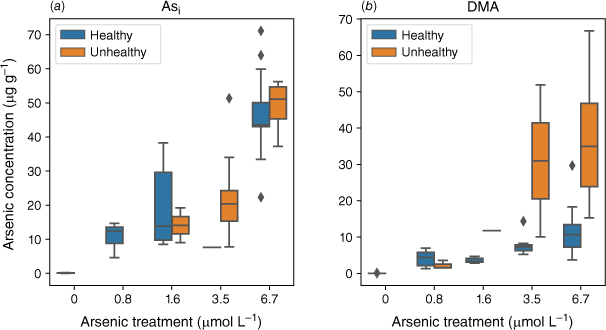
Arsenic exposure has been reported to cause a reduction in both plant growth and root elongation (Han et al. 2015; Seneviratne et al. 2019). In this study, a delayed response has been observed with plant heights and mass decreasing after exposure to As concentrations greater than 1.6 µmol L−1, indicating either that the rice plant has some tolerance to Asi (Song et al. 2014; Xu J et al. 2017) or that Asi is interrupting key metabolic pathways where the effects are not immediately observed (Kamiya et al. 2013). The rice plants exposed to the lower concentrations of Asi appear to display a degree of tolerance. Asi is most likely sequestered into the vacuoles as part of a detoxification mechanism (Song et al. 2014). Although Asi can cause oxidative stress to the rice plant, rice appears to handle low-level exposure, between 0.8 and 1.6 µmol L−1. When exposed to between 1.6 and 3.5 µmol L−1, reduction in growth and plant mass are observed; however, these plants still show the ability to regulate and limit As transport to above-ground tissues. A significant reduction in growth and increased As concentrations in the shoots was measured for the 6.7 µmol L−1 Asi treatment, corresponding to a substantial increase of As transport from the roots to shoots (and other plant tissues). Thus, the higher Asi concentrations used in this study could be approaching concentrations that are toxic for hydroponically grown rice. Shaibur et al. (2006) also found Asi toxicity to be induced in hydroponically grown rice at 6.7 µmol L−1.
It is well documented that AsIII uses silicon transporters (Ma et al. 2008; Katsuhara et al. 2014; Chen Y et al. 2017) and AsV uses phosphate transporters (Wang P et al. 2016) for uptake and translocation (Kumarathilaka et al. 2018), thus As can lead to the disruption of signalling or metabolic pathways. AsIII also has a high affinity to sulfhydryl groups (–SH), and readily reacts with enzymes and proteins (Dixit et al. 2015), inhibiting enzyme activity (Chen W et al. 2010) affecting plant growth and metabolism (Jha and Dubey 2004). AsV can cause a reduction in photosynthetic activity and may delay the effects of arsenic on the health of the plant (Mateos-Naranjo et al. 2012; Abbas et al. 2018). The reduction in height and biomass (Fig. 5 and 6) observed may also be caused by an increase and imbalance in reactive oxygen species (ROS), resulting in oxidative stress throughout the plant (Stoeva et al. 2005; Shri et al. 2009). ROS are signalling compounds and intermediates produced by metabolic pathways in cells that play essential roles throughout the plants’ lifecycle, including plant growth, germination and grain development (Mhamdi and Van Breusegem 2018). Other studies have reported how plants respond to high Asi concentrations, with both AsV and AsIII inducing the production of ROS (Finnegan and Chen 2012) and inducing oxidative stress in the plant and eventual cell death (Hartley‐Whitaker et al. 2001; Tripathi et al. 2012), with AsIII typically having a more pronounced effect (Pessarakli and Tan 2010). Oxidative stress can cause a range of effects in plants (Finnegan and Chen 2012). Generally, this is a function of excess ROS production, leading to DNA damage, protein modification or lipid peroxidation, which results in impaired cellular function and potential cell death (Pessarakli and Tan 2010).
The effects DMA has on rice health are less clear and have not been thoroughly studied. Elevated DMA concentrations in soil have been linked to straighthead disease in rice (Yan et al. 2005); however, the critical role DMA plays has not been established (Meharg and Zhao 2012). Recently, several studies have found that DMA is more phytotoxic to plants than Asi (Tang et al. 2016a, 2016b), primarily due to its mobility within plants (Raab et al. 2007b; Carey et al. 2011) and the plant’s inability to detoxify DMA (Tang et al. 2016a). For each DMA exposure, many plants displayed a significant reduction in growth (Fig. 8). At the higher DMA treatment concentrations, the plants with reduced growth had higher DMA concentrations in their shoots. Tang et al. (2016a) found that plants exposed to DMA exhibited significantly more oxidative stress (lipid peroxidation), particularly in the shoots, compared to plants exposed to AsV or MA.
The higher translocation of DMA could cause stress to plants by localised accumulation of DMA in different sections of the plant. This could also explain the varying effects of DMA on plant health documented in the literature (Finnegan and Chen 2012). In this study, when plant height was used as a proxy for plant health, we observed that unhealthy plants had higher DMA concentrations compared to healthy plants (Fig. 8). This trend was not observed for Asi-exposed plants. This may indicate that different As species cause different types of stress within plants, or it could demonstrate that some plants can detoxify Asi to a certain extent compared to DMA. Once a specific As concentration is reached, the production of ROS results in oxidative stress and, in some cases, cell death. Unlike Asi, which has limited translocation throughout the plant, the high mobility of DMA could result in many different parts of the plant being susceptible to DMA-induced oxidative stress, which has the potential to disrupt vital metabolic pathways.
The exact mechanism of how DMA induces toxicity in plants is still unclear. Typically, DMAV is being analysed when DMA is quantified. DMAIII is unstable under aerobic conditions and is oxidised to the more stable DMAV (Jiang et al. 2003). The toxicity of DMA may be induced by DMAIII; however, changes in DMAIII concentrations cannot be detected using standard methods (Garbinski et al. 2019; Kerl et al. 2019). In animal cells, the trivalent organic arsenic species (DMAIII and MAIII) are more cytotoxic than AsIII and AsV (Petrick et al. 2000; Styblo et al. 2000). Oxidative stress can be induced through the redox cycling of DMAIII and DMAV (Naranmandura et al. 2007). In this hydroponic experiment, due to the nutrient solution being continuously aerated DMAIII was unlikely to be present.
DMA may be the major cause of straighthead disease. Zheng et al. (2013) found that DMA was toxic to reproductive tissues, and Carey et al. (2011) showed that DMA is remobilised and transported to reproductive tissues at the beginning of grain formation. Under these conditions, highly localised DMA accumulation is likely to occur and cause stress to the plant.
Conclusions
Doongara rice plants responded differently when exposed to either Asi or DMA. Asi was taken up at a much faster rate into roots than DMA. Rice plants, however, were able to limit the internal transport of Asi, potentially sequestering a large amount of arsenic into vacuoles. This mechanism allowed the plant to tolerate ‘low’ concentrations of Asi. Rice plants exposed to DMA showed reduced ability to control the distribution of DMA within the plant once accumulated, resulting in greater translocation from roots to shoots, thus illustrating the high mobility and relative lack of detoxification strategies for DMA. Rice plants exposed to Asi and DMA both showed an overall decrease in mean plant heights and masses when exposed to increasing As concentrations, although rice plants exposed to the lower Asi concentrations display a degree of tolerance.
The results presented here highlight that DMA may have a more significant effect on rice plants than previously thought. DMA has the potential to influence the plant’s overall health and fitness, and these effects are different from the stress induced by Asi.
References
Abbas G, Murtaza B, Bibi I, Shahid M, Niazi NK, Khan MI, Amjad M, Hussain M, Natasha (2018) Arsenic uptake, toxicity, detoxification, and speciation in plants: physiological, biochemical, and molecular aspects. International Journal of Environmental Research and Public Health 15, 59.
| Crossref | Google Scholar | PubMed |
Abedi T, Mojiri A (2020) Arsenic uptake and accumulation mechanisms in rice species. Plants 9, 129.
| Crossref | Google Scholar | PubMed |
Abedin MJ, Feldmann J, Meharg AA (2002) Uptake kinetics of arsenic species in rice plants. Plant Physiology 128, 1120-1128.
| Crossref | Google Scholar | PubMed |
Bienert GP, Thorsen M, Schüssler MD, Nilsson HR, Wagner A, Tamás MJ, Jahn TP (2008) A subgroup of plant aquaporins facilitate the bi-directional diffusion of As(OH)3 and Sb(OH)3 across membranes. BMC Biology 6, 26.
| Crossref | Google Scholar |
Carey A-M, Scheckel KG, Lombi E, Newville M, Choi Y, Norton GJ, Charnock JM, Feldmann J, Price AH, Meharg AA (2010) Grain unloading of arsenic species in rice. Plant Physiology 152, 309-319.
| Crossref | Google Scholar | PubMed |
Carey AM, Norton GJ, Deacon C, Scheckel KG, Lombi E, Punshon T, Guerinot ML, Lanzirotti A, Newville M, Choi Y, Price AH, Meharg AA (2011) Phloem transport of arsenic species from flag leaf to grain during grain filling. New Phytologist 192, 87-98.
| Crossref | Google Scholar | PubMed |
Chen C, Li L, Huang K, Zhang J, Xie W-Y, Lu Y, Dong X, Zhao F-J (2019) Sulfate-reducing bacteria and methanogens are involved in arsenic methylation and demethylation in paddy soils. The ISME Journal 13, 2523-2535.
| Crossref | Google Scholar | PubMed |
Chen W, Chi Y, Taylor NL, Lambers H, Finnegan PM (2010) Disruption of ptLPD1 or ptLPD2, genes that encode isoforms of the plastidial lipoamide dehydrogenase, confers arsenate hypersensitivity in Arabidopsis. Plant Physiology 153, 1385-1397.
| Crossref | Google Scholar | PubMed |
Chen Y, Moore KL, Miller AJ, McGrath SP, Ma JF, Zhao F-J (2015) The role of nodes in arsenic storage and distribution in rice. Journal of Experimental Botany 66, 3717-3724.
| Crossref | Google Scholar | PubMed |
Chen Y, Sun S-K, Tang Z, Liu G, Moore KL, Maathuis F, Miller AJ, McGrath SP, Zhao F-J (2017) The Nodulin 26-like intrinsic membrane protein OsNIP3; 2 is involved in arsenite uptake by lateral roots in rice. Journal of Experimental Botany 68, 3007-3016.
| Crossref | Google Scholar | PubMed |
Chen Z, Zhu YG, Liu WJ, Meharg AA (2005) Direct evidence showing the effect of root surface iron plaque on arsenite and arsenate uptake into rice (Oryza sativa) roots. New Phytologist 165, 91-97.
| Crossref | Google Scholar | PubMed |
Dixit G, Singh AP, Kumar A, Singh PK, Kumar S, Dwivedi S, Trivedi PK, Pandey V, Norton GJ, Dhankher OP, Tripathi RD (2015) Sulfur mediated reduction of arsenic toxicity involves efficient thiol metabolism and the antioxidant defense system in rice. Journal of Hazardous Materials 298, 241-251.
| Crossref | Google Scholar | PubMed |
Duan G-L, Hu Y, Liu W-J, Kneer R, Zhao F-J, Zhu Y-G (2011) Evidence for a role of phytochelatins in regulating arsenic accumulation in rice grain. Environmental and Experimental Botany 71, 416-421.
| Crossref | Google Scholar |
Fang Z, Xia K, Yang X, Grotemeyer MS, Meier S, Rentsch D, Xu X, Zhang M (2013) Altered expression of the PTR/NRT 1 homologue Os PTR 9 affects nitrogen utilization efficiency, growth and grain yield in rice. Plant Biotechnology Journal 11, 446-458.
| Crossref | Google Scholar | PubMed |
Fang Z, Bai G, Huang W, Wang Z, Wang X, Zhang M (2017) The rice peptide transporter OsNPF7.3 is induced by organic nitrogen, and contributes to nitrogen allocation and grain yield. Frontiers in Plant Science 8, 1338.
| Crossref | Google Scholar | PubMed |
Finnegan PM, Chen W (2012) Arsenic toxicity: the effects on plant metabolism. Frontiers in Physiology 3, 182.
| Crossref | Google Scholar | PubMed |
Garbinski LD, Rosen BP, Chen J (2019) Pathways of arsenic uptake and efflux. Environment International 126, 585-597.
| Crossref | Google Scholar | PubMed |
Geng A, Lian W, Wang X, Chen G (2023) Regulatory mechanisms underlying arsenic uptake, transport, and detoxification in rice. International Journal of Molecular Sciences 24, 11031.
| Crossref | Google Scholar | PubMed |
Han D, Xiong S, Tu S, Liu J, Chen C (2015) Interactive effects of selenium and arsenic on growth, antioxidant system, arsenic and selenium species of Nicotiana tabacum L. Environmental and Experimental Botany 117, 12-19.
| Crossref | Google Scholar |
Hartley-Whitaker J, Ainsworth G, Vooijs R, Ten Bookum W, Schat H, Meharg AA (2001) Phytochelatins are involved in differential arsenate tolerance in Holcus lanatus. Plant Physiology 126, 299-306.
| Crossref | Google Scholar | PubMed |
Hua B, Yan W, Yang J (2013) Response of rice genotype to straighthead disease as influenced by arsenic level and water management practices in soil. The Science of the Total Environment 442, 432-436.
| Crossref | Google Scholar | PubMed |
Jacobson L (1951) Maintenance of iron supply in nutrient solutions by a single addition of ferric potassium ethylenediamine tetra-acetate. Plant Physiology 26, 411-413.
| Crossref | Google Scholar | PubMed |
Jha AB, Dubey RS (2004) Carbohydrate metabolism in growing rice seedlings under arsenic toxicity. Journal of Plant Physiology 161, 867-872.
| Crossref | Google Scholar | PubMed |
Jia Y, Huang H, Zhong M, Wang F-H, Zhang L-M, Zhu Y-G (2013) Microbial arsenic methylation in soil and rice rhizosphere. Environmental Science & Technology 47, 3141-3148.
| Crossref | Google Scholar | PubMed |
Jiang G, Lu X, Gong Z, Cullen WR, Chris Le X (2003) Chapter 4 - Trivalent arsenic species: analysis, stability, and interaction with a protein. In ‘Arsenic Exposure and Health Effects V’. (Eds WR Chappell, CO Abernathy, RL Calderon, DJ Thomas) pp. 51–68. (Elsevier Science B.V.: Amsterdam, Netherlands)
Kamiya T, Islam R, Duan G, Uraguchi S, Fujiwara T (2013) Phosphate deficiency signaling pathway is a target of arsenate and phosphate transporter OsPT1 is involved in As accumulation in shoots of rice. Soil Science and Plant Nutrition 59, 580-590.
| Crossref | Google Scholar |
Katsuhara M, Sasano S, Horie T, Matsumoto T, Rhee J, Shibasaka M (2014) Functional and molecular characteristics of rice and barley NIP aquaporins transporting water, hydrogen peroxide and arsenite. Plant Biotechnology 31, 213-219.
| Crossref | Google Scholar |
Kerl CF, Schindele RA, Brüggenwirth L, Colina Blanco AE, Rafferty C, Clemens S, Planer-Friedrich B (2019) Methylated thioarsenates and monothioarsenate differ in uptake, transformation, and contribution to total arsenic translocation in rice plants. Environmental Science & Technology 53, 5787-5796.
| Crossref | Google Scholar | PubMed |
Kim D-W, Rakwal R, Agrawal GK, Jung Y-H, Shibato J, Jwa N-S, Iwahashi Y, Iwahashi H, Kim DH, Shim I, Usui K (2005) A hydroponic rice seedling culture model system for investigating proteome of salt stress in rice leaf. Electrophoresis 26, 4521-4539.
| Crossref | Google Scholar | PubMed |
Kumarathilaka P, Seneweera S, Meharg A, Bundschuh J (2018) Arsenic accumulation in rice (Oryza sativa L.) is influenced by environment and genetic factors. Science of The Total Environment 642, 485-496.
| Crossref | Google Scholar | PubMed |
Lemos Batista B, Nigar M, Mestrot A, Alves Rocha B, Barbosa Júnior F, Price AH, Raab A, Feldmann J (2014) Identification and quantification of phytochelatins in roots of rice to long-term exposure: evidence of individual role on arsenic accumulation and translocation. Journal of Experimental Botany 65, 1467-1479.
| Crossref | Google Scholar |
Li R-Y, Ago Y, Liu W-J, Mitani N, Feldmann J, McGrath SP, Ma JF, Zhao F-J (2009) The rice aquaporin Lsi1 mediates uptake of methylated arsenic species. Plant Physiology 150, 2071-2080.
| Crossref | Google Scholar | PubMed |
Limmer MA, Seyfferth AL (2020) The role of small molecules in restricting rice accumulation of dimethylarsinic acid. Plant and Soil 447(1–2), 599-609.
| Crossref | Google Scholar |
Ma JF, Yamaji N, Mitani N, Xu X-Y, Su Y-H, McGrath SP, Zhao F-J (2008) Transporters of arsenite in rice and their role in arsenic accumulation in rice grain. Proceedings of the National Academy of Sciences 105, 9931-9935.
| Crossref | Google Scholar | PubMed |
Maher W, Foster S, Krikowa F, Donner E, Lombi E (2013) Measurement of inorganic arsenic species in rice after nitric acid extraction by HPLC-ICPMS: verification using XANES. Environmental Science & Technology 47, 5821-5827.
| Crossref | Google Scholar | PubMed |
Maher W, Duncan E, Martin H, Snell P, Krikowa F, Jagtap R, Foster S, Ezaz T, Ellwood MJ (2018) Arsenic concentrations and speciation in Australian and imported rice and commercial rice products. Environmental Chemistry 15, 387-402.
| Crossref | Google Scholar |
Martin HP (2020) Investigating the role of dimethyl-arsenic in inducing straighthead disease in rice. PhD thesis, Australian National University, Canberra, ACT, Australia. doi:10.25911/SH8V-EN25
Martin HP, Maher WA, Snell PJ, Philpot KJ, Ellwood MJ (2023) The uptake of arsenic species by commonly grown Australian rice varieties cultivated utilising two widely used agronomic practices (straw incorporation and nitrogen fertilisation) and the role dimethyl arsenic plays in inducing straighthead disease. Environmental Chemistry 20, 83-94.
| Crossref | Google Scholar |
Mateos-Naranjo E, Andrades-Moreno L, Redondo-Gómez S (2012) Tolerance to and accumulation of arsenic in the cordgrass Spartina densiflora Brongn. Bioresource Technology 104, 187-194.
| Crossref | Google Scholar | PubMed |
Meharg AA, Zhao FJ (2012) ‘Arsenic & Rice.’ (Springer: Dordrecht, Netherlands) doi:10.1007/978-94-007-2947-6
Mhamdi A, Van Breusegem F (2018) Reactive oxygen species in plant development. Development 145, dev164376.
| Crossref | Google Scholar | PubMed |
Mishra S, Mattusch J, Wennrich R (2017) Accumulation and transformation of inorganic and organic arsenic in rice and role of thiol-complexation to restrict their translocation to shoot. Scientific Reports 7, 40522.
| Crossref | Google Scholar | PubMed |
Moore KL, Schröder M, Wu Z, Martin BG, Hawes CR, McGrath SP, Hawkesford MJ, Feng Ma J, Zhao F-J, Grovenor CR (2011) High-resolution secondary ion mass spectrometry reveals the contrasting subcellular distribution of arsenic and silicon in rice roots. Plant Physiology 156, 913-924.
| Crossref | Google Scholar | PubMed |
Murugaiyan V, Zeibig F, Anumalla M, Siddiq SA, Frei M, Murugaiyan J, Ali J (2021) Arsenic stress responses and accumulation in rice. In ‘Rice Improvement: Physiological, Molecular Breeding and Genetic Perspectives’. (Eds J Ali, SH Wani) pp. 281–313. (Springer International Publishing: Cham, Switzerland) doi:10.1007/978-3-030-66530-2_9
Naranmandura H, Ibata K, Suzuki KT (2007) Toxicity of dimethylmonothioarsinic acid toward human epidermoid carcinoma A431 cells. Chemical Research in Toxicology 20, 1120-1125.
| Crossref | Google Scholar | PubMed |
Palmer MJ, Jamieson HE, Borčinová Radková A, Maitland K, Oliver J, Falck H, Richardson M (2021) Mineralogical, geospatial and statistical methods combined to estimate geochemical background of arsenic in soils for an area impacted by legacy mining pollution. Science of the Total Environment 776, 145926.
| Crossref | Google Scholar | PubMed |
Petrick JS, Ayala-Fierro F, Cullen WR, Carter DE, Vasken Aposhian H (2000) Monomethylarsonous acid (MMAIII) is more toxic than arsenite in Chang human hepatocytes. Toxicology and Applied Pharmacology 163, 203-207.
| Crossref | Google Scholar | PubMed |
Raab A, Schat H, Meharg AA, Feldmann J (2005) Uptake, translocation and transformation of arsenate and arsenite in sunflower (Helianthus annuus): formation of arsenic–phytochelatin complexes during exposure to high arsenic concentrations. New Phytologist 168, 551-558.
| Crossref | Google Scholar | PubMed |
Raab A, Ferreira K, Meharg AA, Feldmann J (2007a) Can arsenic–phytochelatin complex formation be used as an indicator for toxicity in Helianthus annuus? Journal of Experimental Botany 58, 1333-1338.
| Crossref | Google Scholar | PubMed |
Raab A, Williams PN, Meharg A, Feldmann J (2007b) Uptake and translocation of inorganic and methylated arsenic species by plants. Environmental Chemistry 4, 197-203.
| Crossref | Google Scholar |
Rahman MA, Hasegawa H, Rahman MM, Miah MAM, Tasmin A (2008) Straighthead disease of rice (Oryza sativa L.) induced by arsenic toxicity. Environmental and Experimental Botany 62, 54-59.
| Crossref | Google Scholar |
Sarwar T, Khan S, Muhammad S, Amin S (2021) Arsenic speciation, mechanisms, and factors affecting rice uptake and potential human health risk: a systematic review. Environmental Technology & Innovation 22, 101392.
| Crossref | Google Scholar |
Seneviratne M, Rajakaruna N, Rizwan M, Madawala HMSP, Ok YS, Vithanage M (2019) Heavy metal-induced oxidative stress on seed germination and seedling development: a critical review. Environmental Geochemistry and Health 41, 1813-1831.
| Crossref | Google Scholar | PubMed |
Shaibur MR, Kitajima N, Sugawara R, Kondo T, Huq SMI, Kawai S (2006) Physiological and mineralogical properties of arsenic-induced chlorosis in rice seedlings grown hydroponically. Soil Science and Plant Nutrition 52, 691-700.
| Crossref | Google Scholar |
Shinde A, Kumar K (2021) Mechanisms of arsenic transport, accumulation, and distribution in rice. In ‘Arsenic Toxicity: Challenges and Solutions’. (Ed. N Kumar) pp. 279–300. (Springer: Singapore) doi:10.1007/978-981-33-6068-6_11
Shri M, Kumar S, Chakrabarty D, Trivedi PK, Mallick S, Misra P, Shukla D, Mishra S, Srivastava S, Tripathi RD, Tuli R (2009) Effect of arsenic on growth, oxidative stress, and antioxidant system in rice seedlings. Ecotoxicology and Environmental Safety 72, 1102-1110.
| Crossref | Google Scholar | PubMed |
Sohn E (2014) Contamination: the toxic side of rice. Nature 514, S62-S63.
| Crossref | Google Scholar |
Song W-Y, Park J, Mendoza-Cózatl DG, Suter-Grotemeyer M, Shim D, Hörtensteiner S, Geisler M, Weder B, Rea PA, Rentsch D, Schroeder JI, Lee Y, Martinoia E (2010) Arsenic tolerance in Arabidopsis is mediated by two ABCC-type phytochelatin transporters. Proceedings of the National Academy of Sciences 107, 21187-21192.
| Crossref | Google Scholar | PubMed |
Song W-Y, Yamaki T, Yamaji N, Ko D, Jung K-H, Fujii-Kashino M, An G, Martinoia E, Lee Y, Ma JF (2014) A rice ABC transporter, OsABCC1, reduces arsenic accumulation in the grain. Proceedings of the National Academy of Sciences 111, 15699-15704.
| Crossref | Google Scholar | PubMed |
Stoeva N, Berova M, Zlatev Z (2005) Effect of arsenic on some physiological parameters in bean plants. Biologia Plantarum 49, 293-296.
| Crossref | Google Scholar |
Styblo M, Del Razo LM, Vega L, Germolec DR, LeCluyse EL, Hamilton GA, Reed W, Wang C, Cullen WR, Thomas DJ (2000) Comparative toxicity of trivalent and pentavalent inorganic and methylated arsenicals in rat and human cells. Archives of Toxicology 74, 289-299.
| Crossref | Google Scholar | PubMed |
Takahashi Y, Minamikawa R, Hattori KH, Kurishima K, Kihou N, Yuita K (2004) Arsenic behavior in paddy fields during the cycle of flooded and non-flooded periods. Environmental Science & Technology 38, 1038-1044.
| Crossref | Google Scholar | PubMed |
Tang Z, Kang Y, Wang P, Zhao F-J (2016a) Phytotoxicity and detoxification mechanism differ among inorganic and methylated arsenic species in Arabidopsis thaliana. Plant and Soil 401, 243-257.
| Crossref | Google Scholar |
Tang Z, Lv Y, Chen F, Zhang W, Rosen BP, Zhao F-J (2016b) Arsenic methylation in Arabidopsis thaliana expressing an algal arsenite methyltransferase gene increases arsenic phytotoxicity. Journal of Agricultural and Food Chemistry 64, 2674-2681.
| Crossref | Google Scholar | PubMed |
Tang Z, Chen Y, Chen F, Ji Y, Zhao F-J (2017) OsPTR7 (OsNPF8. 1), a putative peptide transporter in rice, is involved in dimethylarsenate accumulation in rice grain. Plant & Cell Physiology 58, 904-913.
| Crossref | Google Scholar | PubMed |
Tang Z, Wang Y, Gao A, Ji Y, Yang B, Wang P, Tang Z, Zhao F-J (2020) Dimethylarsinic acid is the causal agent inducing rice straighthead disease. Journal of Experimental Botany 71, 5631-5644.
| Crossref | Google Scholar | PubMed |
Tripathi P, Mishra A, Dwivedi S, Chakrabarty D, Trivedi PK, Singh RP, Tripathi RD (2012) Differential response of oxidative stress and thiol metabolism in contrasting rice genotypes for arsenic tolerance. Ecotoxicology and Environmental Safety 79, 189-198.
| Crossref | Google Scholar | PubMed |
Tsay Y-F, Chiu C-C, Tsai C-B, Ho C-H, Hsu P-K (2007) Nitrate transporters and peptide transporters. FEBS Letters 581, 2290-2300.
| Crossref | Google Scholar | PubMed |
Vázquez Reina S, Esteban E, Goldsbrough P (2005) Arsenate‐induced phytochelatins in white lupin: influence of phosphate status. Physiologia Plantarum 124, 41-49.
| Crossref | Google Scholar |
Wang P, Zhang W, Mao C, Xu G, Zhao F-J (2016) The role of OsPT8 in arsenate uptake and varietal difference in arsenate tolerance in rice. Journal of Experimental Botany 67, 6051-6059.
| Crossref | Google Scholar | PubMed |
Xu J, Shi S, Wang L, Tang Z, Lv T, Zhu X, Ding X, Wang Y, Zhao FJ, Wu Z (2017) OsHAC4 is critical for arsenate tolerance and regulates arsenic accumulation in rice. New Phytologist 215, 1090-1101.
| Crossref | Google Scholar | PubMed |
Xu XY, McGrath SP, Meharg AA, Zhao FJ (2008) Growing rice aerobically markedly decreases arsenic accumulation. Environmental Science & Technology 42, 5574-5579.
| Crossref | Google Scholar | PubMed |
Yamaji N, Ma JF (2014) The node, a hub for mineral nutrient distribution in graminaceous plants. Trends in Plant Science 19, 556-563.
| Crossref | Google Scholar | PubMed |
Yan W, Dilday RH, Tai TH, Gibbons JW, McNew RW, Rutger JN (2005) Differential response of rice germplasm to straighthead induced by arsenic. Crop Science 45, 1223-1228.
| Crossref | Google Scholar |
Ye J, Rensing C, Rosen BP, Zhu Y-G (2012) Arsenic biomethylation by photosynthetic organisms. Trends in Plant Science 17, 155-162.
| Crossref | Google Scholar | PubMed |
Zhao F-J, Ma JF, Meharg AA, McGrath SP (2009) Arsenic uptake and metabolism in plants. New Phytologist 181, 777-794.
| Crossref | Google Scholar | PubMed |
Zhao F-J, Ago Y, Mitani N, Li R-Y, Su Y-H, Yamaji N, McGrath SP, Ma JF (2010) The role of the rice aquaporin Lsi1 in arsenite efflux from roots. New Phytologist 186, 392-399.
| Crossref | Google Scholar | PubMed |
Zhao F-J, Stroud JL, Khan MA, McGrath SP (2012) Arsenic translocation in rice investigated using radioactive 73As tracer. Plant and Soil 350, 413-420.
| Crossref | Google Scholar |
Zhao F-J, Moore KL, Lombi E, Zhu Y-G (2014) Imaging element distribution and speciation in plant cells. Trends in Plant Science 19, 183-192.
| Crossref | Google Scholar | PubMed |
Zheng M-Z, Cai C, Hu Y, Sun G-X, Williams PN, Cui H-J, Li G, Zhao F-J, Zhu Y-G (2011) Spatial distribution of arsenic and temporal variation of its concentration in rice. New Phytologist 189, 200-209.
| Crossref | Google Scholar | PubMed |
Zheng M-Z, Li G, Sun G-X, Shim H, Cai C (2013) Differential toxicity and accumulation of inorganic and methylated arsenic in rice. Plant and Soil 365, 227-238.
| Crossref | Google Scholar |