Effect of wetting and drying processes on ultramafic and mafic tailing minerals amended with topsoil
Lewis Fausak






A
Abstract
Mine tailings are a mixture of fine materials obtained after crushing, processing and extracting the valuable minerals from ore. Ultramafic and mafic mine tailings have the potential to mineralise carbon, offering a solution to offset greenhouse gas emissions from the mining sector. The study revealed that the effects of wetting and drying ultramafic and mafic mine tailings under atmospheric conditions have the potential for carbon sequestration and acid mine drainage.
As the result of their carbon mineralisation potential, there is an increasing interest in using ultramafic and mafic (U+M) mine tailings as a feedstock for carbon (C) sequestration. However, little is known about the relative chemical stability of U+M minerals, and it is unclear whether acid mine drainage may be generated during weathering.
This study determined the relative stability of the constituent minerals of several U+M tailings from mines in British Columbia, Canada, and Australia using selective chemical extractions and an 18-week laboratory experiment of cycles of wetting and drying to simulate conditions that may be experienced under field conditions. Tailings were mixed with topsoil to investigate the potential use as a soil amendment.
Initially, the tailing sample’s pH was 8.4–9.7 and decreased to 7.7–9.1 over the 18 weeks. Soil additions lowered the initial pH and converged with the tailing’s pH over 18 weeks. Sequentially weathered minerals determined by X-ray diffraction were consistent with the empirical Goldich weathering sequence. Metal concentrations from inductively coupled plasma–mass spectrometry supported X-ray diffraction results, confirming the stability of the U+M tailings using different methods and the need for future studies on potential metal contamination.
Minor concentrations of sulfur seemed to have resulted in larger fluctuations in pH when low amounts of carbonates were present. However, reactive oxides and basic cations in the U+M tailings maintained pH above 7. Therefore, C sequestration was likely supported, although there was a slight reduction in total C content for almost all samples. This study suggests future research is required under field conditions to confirm C sequestration and to investigate the use of U+M tailings for restoration applications.
Keywords: carbon sequestration, CO2 mineralisation, hydroxide minerals, mafic tailings, magnesium silicates, soil amendments, ultramafic tailings, weathering.
Introduction
Approximately 419 × 106 Mg of ultramafic and mafic (U+M) mine tailings are produced yearly from the global production of metals and minerals (Power et al. 2013). Mine tailings are the mixture of fine materials obtained after crushing, processing and extracting an ore’s valuable minerals (Power et al. 2020). U+M tailings can support climate change mitigation with a long-term and stable form of carbon (C) sequestration obtained through a process called carbon mineralisation, due to their high magnesium and iron content, whereby carbon dioxide (CO2) reacts with the divalent metal-bearing oxides groups in U+M tailings (magnesium silicates and hydroxide minerals) to produce carbonate minerals (Power et al. 2020). However, little is known about the relative chemical stability of the U+M minerals, especially in relation to acid mine drainage (AMD), over the long term (Baumeister 2012; Power et al. 2020; Lu et al. 2022).
Silicate minerals found in U+M tailings include olivine (forsterite-fayalite solid solution, (Mg, Fe)2SiO4), pyroxenes including clinopyroxene and orthopyroxene (diopside, CaMgSi2O6; and enstatite, (Mg, Fe2+)(SiO3)), serpentine (Mg3(Si2O5)(OH)4), chromite (FeCr2O4), amphiboles (such as anthophyllite, (Mg, Fe2+)7(Si8O22)(OH, F)2; and tremolite, Ca2(Mg, Fe2+)5(Si8O22)(OH, F)2), garnet including pyrope (Mg3Al2Si3O12) and grossular (Ca3Al2Si3O12), and spinel (MgAl2O4). Chakravarthy et al. (2020) and Power et al. (2020) have documented that these minerals and their chemical composition influence the environment in which they are found, and their importance for climate change mitigation. U+M minerals were formed under conditions very different to those at the Earth’s surface, where they encounter oxidation and hydrolysis reactions. Thus, they are chemically unstable when exposed to a surficial oxidising environment and are subject to chemical weathering. Although U+M tailings are potential feedstock to sequester C, the weathering process may lead to AMD, once the basic cations become exhausted to neutralise the acid-generating minerals. AMD can lead to metal leaching causing adverse environmental impacts including contamination of groundwater, surface water and soil (Saria et al. 2006).
The classical weathering sequence proposed by Goldich (1938) describes the relative stability of the dominant mafic minerals from least to more stable as olivine, pyroxene, hornblende and biotite. As weathering of minerals occurs, U+M tailing minerals contribute to metal leaching and release of heavy metals which are present in accessory minerals or included as trace metals within silicate minerals, including iron (Fe), cadmium (Cd), arsenic (As), chromium (Cr), thallium (Th) and lead (Pb), as well as other potentially harmful elements including aluminium (Al), cobalt (Co), nickel (Ni) and vanadium (V) (Saria et al. 2006; Evans 2013; Malli et al. 2015).
Weathering of sulfide containing minerals, notably pyrite, is the primary source of acid generation responsible for AMD (Hayes et al. 2014). Although acid-producing minerals such as pyrite are not abundant in U+M ore bodies, they can occur in small amounts and potentially contribute to acid generation in the long term (Keays 1987). AMD results from the oxidation of sulfides in the presence of water and oxygen and is accelerated by microorganisms to release sulfate (SO42−), metals (Fe2+) and protons (H+) into solution (Schippers et al. 2010). Additionally, U+M minerals, when subjected to prolonged saturation with water, can become acidic and dissolve iron (Fe), resulting in Fe sulfide oxidation (Center for Science in Public Participation 2014; Kefeni et al. 2017). These low pH and metal enriched waters can negatively affect the quality of the ecosystem and aquatic life, largely affecting nearby rivers, lakes, estuaries and coastal waters (Lottermoser 2010). Once initiated, AMD and metal leaching from tailings can continue long after the cessation of mining activities, posing a long-term liability to mining operations (Malli et al. 2015). However, simulations of rate reaction calculations show that silicate minerals have some level of neutralising capacity, which can aid with AMD (Eary and Williamson 2006).
Acid neutralisation also occurs if basic cations are released during AMD and may mitigate the acidification process. However, the rate at which acid or basic cations are released may not be congruent and commonly results in an acidic condition (Cruz-Hernández et al. 2019). These processes have been studied by sequential dissolution techniques as relative measures of the release of basic elements, such as calcium (Ca) and sodium (Na), that promote acid neutralisation (Davidson et al. 2004; Saria et al. 2006; Kefeni et al. 2017). Additionally, soils can be added as an amendment to mine tailings to dilute metal concentrations, inoculate tailings with microbes, or alter pH (Dvořáčková et al. 2022). However, this amendment has not been thoroughly investigated for use in mitigating AMD of U+M tailings. Soils with high clay or organic matter content could be particularly useful in stabilising the mine tailing's physical, chemical and biological characteristics, including changes in pH, electrical conductivity, metal contents, soil C and soil organisms (Feng et al. 2019).
To investigate the effects of weathering on U+M tailings, an 18-week laboratory experiment of wetting and drying cycles was conducted under atmospheric conditions to simulate chemical weathering of U+M tailings with the overall goal of assessing U+M tailing's potential for C sequestration and AMD.
The objectives of this study were to:
To determine the relative stability of the constituent minerals of U+M tailings by selective chemical extractions with an increasing degree of strength of extractability – using mineral acid (HCl), complexing extractant (ammonium oxalate acid, AAO) and ‘total’ (aqua regia).
To quantify changes in the pH, chemical and mineralogical composition of U+M tailings (with and without topsoil amendments) subjected to repeated wetting and drying cycles.
Experimental
Sample selection and preparations
Five U+M mine tailings with different mineralogical compositions were selected from Western Australia and western Canada (Table 1). Mining operations did not remove sulfides during processing of ore. The topsoil used was collected from the 0–30 cm of cultivated surface of an agricultural field on Westham Island, Delta, BC. The soil texture was silt loam – silty clay loam with total soil C content of 0.97%, total sulfur (S) of 0.12%, a pH of 4.1–4.7 and soil mineralogical composition was dominated by smectite clay (Table 1).
Samples | Location | Mineralogical composition | |
---|---|---|---|
Serpentinite (MKKNi) | Mount Keith Nickel Mine, Australia | Serpentine minerals, antigorite, lizardite and minor chrysotile (Mg3(Si2O5)(OH)4), with hydrotalcite-group minerals including iowaite (Mg6Fe2(OH)16Cl2·4H2O) and woodallite (Mg6Cr2(OH)16Cl2·4H2O) with occasional pyroaurite (Mg6Fe2(OH)16CO3·4H2O), stichtite (Mg6Cr2(OH)16CO3·4H2O) and uncommon mountkeithite ((Mg, Ni)11(Fe, Cr)3(OH)24(SO4, CO3)3.5·11H2O) (Wilson et al. 2014). | |
Fine processed kimberlite (FPK) | Gahcho Kue Diamond Mine, Northwest Territories, Canada | Calcite (CaCO3), chrysotile (Mg3(Si2O5)(OH)4), forsterite (Mg2SiO4), microcline (KAlSi3O8), montmorillonite ((Na, Ca)0.3(Al, Mg)2Si4O10(OH)2·nH2O), phlogopite (KMg3(AlSi3O10)(OH)2), quartz (SiO2) and talc (Mg6(Si8O20)(OH)4) (Johnson and Pilotto 2018; Cutts et al. 2020). | |
Nickel smelter granulated slag (SS) | Kalgoorlie, Western Australia | Major phases include: pentlandite ((Fe, Ni)9S8), heazlewoodite (Ni3S2) and awaruite (Ni3Fe), magnetite (Fe·Fe2O4), bornite (Cu5FeS4), chromite (FeCr2O4) and fayalite (Fe2(SiO4)) constitute the minor and trace phases (Page 1982). | |
Gabbro deposit (GT) | Tulameen, British Columbia, Canada | Dunite (dominantly olivine, (Mg, Fe)2SiO4), olivine clinopyroxenite (Mg2SiO4), hornblende clinopyroxenite (Ca2(Mg, Fe, Al)5(Al, Si)8O22(OH)2), peridotite, clinopyroxenite, hornblende-olivine clinopyroxenite, hornblendite and pegmatite (dominantly quartz, SiO2; muscovite, K2Al4(Si6Al2O20)(OH, F)4; and feldspar, (K, Na)(AlSi3O8)) (Findlay 1969; Nixon and Rublee 1988). | |
Serpentinised intrusive (GINi) | Turnagain Nickel Cobalt Mine, British Columbia, Canada | Forsterite (Mg2SiO4), lizardite (Mg3(Si2O5)(OH)4), magnetite (Fe·Fe2O4) and maghemite (γ-Fe2O3), diopside (CaMgSi2O6) and minor minerals (quartz, SiO2; brucite, Mg(OH)2; clinochlore, (Mg5Al)(AlSi3)O10(OH)8; troillite, FeS; and sjoegrenite, MgAl2O4(OH)4·6H2O) (Cutts et al. 2020). | |
Delta Soil (Topsoil) | Westham Island, Delta, British Columbia, Canada | Smectite (½(Ca, Na)0.7(Al, Mg, Fe)4((Si, Al)8O20)(OH)6·nH2O), vermiculite ((Mg, Ca)0.7(Mg, Fe2+, Al)6((Al, Si)8O20)(OH)4·8(H2O)), mica (illite) (K1−1.5Al4(Si7−6.5Al1−1.5O20)(OH)4), chlorite (Mg, Al, Fe)12((Si, Al)8O20)(OH)16), plagioclase (Na(AlSi3O8)–Ca(Al2Si2O8)) and quartz (SiO2) (Luttmerding 1981). |
These data were gathered from several different sources.
The soil was air-dried, sieved through a 10-mesh (2-mm) sieve and stored at room temperature (~20°C) until used in the simulated chemical weathering experiment (SCW). Prior to chemical analysis, mine tailings were ground using a ceramic mortar and pestle and passed through a 120-mesh (125-µm) sieve.
Selective chemical extraction and digestion
HCl and acid ammonium oxalate (AAO) extractions, and aqua regia (AR) digestion, were used to assess the relative mineral stability and elemental release from the tailing samples (Fig. 1) (Fanfani et al. 1997; Moncur and Smith 2012). Based on the manual from the Canadian Council of Ministers of the Environment (2016), samples were extracted using: 0.1 M HCl for an estimate of the readily soluble elements and used to measure mobile or plant available elements; AAO, a complexing agent, to estimate the poorly crystalline material, and amorphous inorganic and organically complexed forms of iron, aluminium and manganese; and AR to provide an estimate of total elemental concentration, except for elements held within aluminosilicate compounds (McKeague and Day 1966; Chen and Ma 2001; Evans 2013; Weaver et al. 2018). Extractions and digestion were conducted in duplicate.
Experiment set up and respective analyses related to the two study objectives – the selective chemical extraction and digestion and the simulated chemical weathering experiment (SCW).
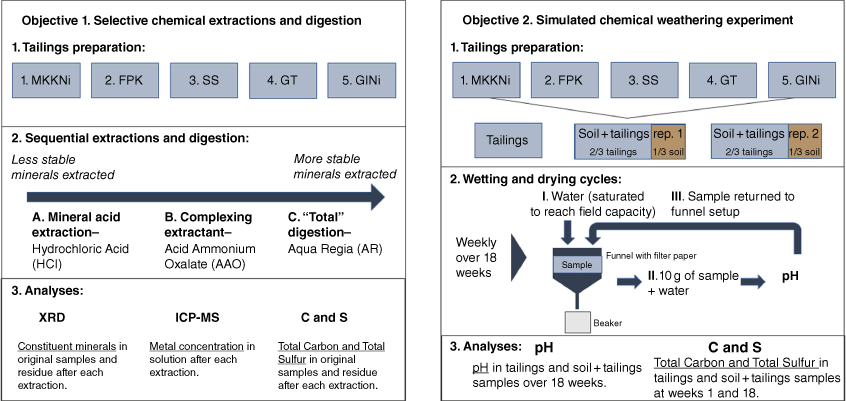
A 5.00-g sample (±0.05 g) of each tailing was measured into a 100-mL Falcon tube with 50 mL of 0.1 M HCl and placed onto a shaker with 5 cm, 240 oscillations per minute for 1 h. The suspension was filtered (Whatman number 42, pore size ~2.5 µm), placed into clean 100 mL Falcon tubes and stored at 4°C until analysed by inductively coupled plasma–mass spectrometry (ICP-MS) (Chen and Ma 2001). The residue in the filter paper was air-dried and stored for X-ray diffraction (XRD) and C and S analysis.
A 1.00-g sample (±0.05 g) of each tailing was weighed into 100-mL Falcon tubes and mixed with 40 mL of AAO solution (0.2 M ammonium oxalate and 0.2 M oxalic acid). The tubes were stoppered and placed on a shaker with 240 oscillations per minute for 4 h. The suspension was filtered (Whatman number 42, pore size ~2.5 µm) and stored at 4°C until analysed by ICP-MS, as above. The residue on the filter paper was treated as above.
A 0.5-g sample (±0.05 g) of each tailing was weighed into a 500-mL Erlenmeyer flask and 12 mL of AR was added (3:1 ratio of HCl/HNO3 (v/v)). The suspension was heated on a hot plate to 110°C for 3 h or until dry. In total, 5 mL of AR was added to the mixture and heated until dry. Finally, 20 mL of 4% HNO3 solution was added to the dried samples, the samples were then filtered (Whatman number 42, pore size ~2.5 µm), placed into a 100-mL volumetric flask and made to volume with 4% HNO3. The filtrate was stored at 4°C until analysed by ICP-MS analysis. The residue on the filter paper was treated as above.
Mineralogical identification
The tailings and filter residues from the three extractions were milled and analysed using a Bruker D8 Focus diffractometer equipped with a Lynx Eye detector and fine-focus Co X-ray tube operating at 35 kV and 40 mA (Fig. 1). Data were collected over a range of 3–80° 2θ with a step size of 0.03° 2θ and a counting time of 0.07 s per step. Identification of minerals was performed using DIFFRAC. EVA V.5 (Bruker AXS) and referencing patterns from the ICDD PDF-4+ database using the Rietveld (2014) method.
Metal analysis was conducted using ICP-MS to detect Al, Ca, Cd, Co, Cr, Cu, Fe, K, Mg, Mn, Na, Ni, P, Pb, Si, V and Zn in leachate samples and extracts (Fig. 1). Ten standards of varying concentrations and one blank were prepared in 2% HNO3 and the samples were analysed using a Varian 725ES Optical Emission Spectrometer (Varian, Santa Clara, CA, USA).
The SCW was set up to assess how tailings vary in pH after repeated wetting and drying cycles that simulated normal atmospheric conditions at room temperature (21°C) and to determine how topsoil amendments may affect changes in pH and mineral composition (Fig. 1). As an exploratory process-focused study, only one tailing sample of 300 g and two replicates for each soil + tailing sample were used. For the soil + tailing samples, 200 g of each mine tailing were homogenised with 100 g of topsoil. To simulate redox conditions encountered during weathering, we created conditions under which oxidation and reduction were likely to occur by saturating samples with deionised water and air drying on a weekly cycle. Büchner funnels lined with filters (Whatman number 42, pore size ~2.5 µm) were used, to which the samples were added and placed on a rack with Erlenmeyer flasks below (Fig. 1). Once per week (for 18 weeks), the samples were saturated with deionised water and mixed to ensure saturation (reducing conditions). Water was drained to allow samples to reach field capacity. To measure pH, 10 g of the sample at field capacity was added to 20 mL of deionised water and shaken at 240 oscillations per minute for 1 min. The sample was allowed to settle for 20 min, and the pH was measured using an Oakton PC700 series benchtop meter. The samples were returned to the funnels, mixed thoroughly and left to dry at room temperature (22–25°C) for 1 week between pH measurements to create oxidising conditions. The deionised water used was made fresh daily and had a pH range between 6 and 7.
The tailings, sample residues from the three extractions and samples after the 18-week SCW were ground with a mortar and pestle, sieved to <150 µm (100-mesh sieve) and analysed for C and S using a Heraeus Micro Analyser (The Elementar Vario MICRO cube) (Fig. 1). As AAO contains C, these extracts were not analysed for carbon. The C content of the soil + tailing samples were estimated using the ratio of soil to tailing (1:2) based on the C content of the Delta soil and tailings samples.
Data presentation and statistical analysis
As the objectives of the study were by design exploratory, the experimental design was not amenable to the application of parametric statistics, thus non-parametric methods were used to calculate the standard error (s.e.) using the formula:
where s.d. is the standard deviation and n is the number of replicates.
Where concentrations were below the detection limit for ICP-MS and the C and S analysis, non-detected (n.d.) was assigned. Concentrations of Cd and Pb were below the detection limit for all samples and values were not used in further analysis. Metals with total concentrations from AR extraction below 1000 ppm were classified as trace metals and total concentrations above 1000 ppm as macro metals. The total metal concentrations were compared to Canadian Council of Ministers of the Environment (2022) guidelines for the Protection of Environmental and Human Health for Agricultural and Residential–Parkland purposes to identify any metals that may be released at unsafe amounts.
Results
X-Ray diffraction mineral identification
Most tailings showed no noticeable dissolution of minerals with AAO and HCl extraction, except for brucite, hydrotalcite and hydromagnesite for MKKNi and GINi (Table 2). The AR dissolved most minerals in the majority of tailings, and transformed the mineral into amorphous–precipitate materials (Scott et al. 2021). However, quartz, feldspar, mica and serpentine minerals remained after all three extractions.
Sample | Tailing minerals | Minerals dissolved relative to starting material | |||
---|---|---|---|---|---|
HCl | AAO | AR | |||
Serpentinite (MKKNi) | Brucite | ✓ | ✓ | ||
Hydromagnesite | ✓ | ✓ | ✓ | ||
Hydrotalcite | ✓ | ✓ | |||
Magnesite | ✓ | ||||
Magnetite | ✓ | ||||
Serpentine | ✓ A | ||||
Talc | ✓ A | ||||
Fine processed kimberlite (FPK) | Calcite | ✓ | |||
Chlorite | ✓ | ||||
Pyrophyllite | ✓ | ||||
Dolomite | ✓ | ||||
Feldspar | |||||
Mica | ✓ A | ||||
Quartz | |||||
Serpentine | ✓ | ||||
Talc | ✓ | ||||
Nickel smelter granulated slag (SS) | Magnetite | ✓ | |||
Olivine | ✓ | ||||
Amorphous material | ✓ | ||||
Gabbro deposit (GT) | Amphibole | ||||
Calcite | ✓ | ||||
Chlorite | ✓ | ||||
Dolomite | |||||
Feldspar | |||||
Mica | |||||
Quartz | |||||
Serpentinised intrusive (GlNi) | Brucite | ✓ | ✓ | ✓ | |
Chlorite | ✓ | ||||
Hydrotalcite | ✓ | ✓ | ✓ | ||
Magnetite | ✓ | ||||
Mica | ✓ | ||||
Olivine | ✓ | ||||
Serpentine | ✓ A |
Calcite, brucite, hydrotalcite and hydromagnesite were more easily weathered than other secondary minerals. Other minerals with relatively high weathering potentials in the mine tailings included calcite, chlorite, olivine, clays, magnesite and magnetite. The primary minerals amphibole, feldspar, mica and quartz in some of the tailings are more stable and exhibited little dissolution (Table 2). The main Mg sources were brucite, hydrotalcite, hydromagnesite, magnesite, olivine and magnetite (Table 2). Main Fe sources were magnetite, serpentine and olivine. The main source of Ca was calcite and the Al main sources were hydrotalcite, clay and serpentine (Table 2).
Metal analysis
For the bioavailable extraction (HCl), the elemental concentrations of Cr, Cu, V and Zn were the lowest, whereas the highest were Fe, Mg and Ca (Table 3). For the AAO extraction (Table 4), Ca and Mn had concentrations below the detection limit for most samples. Fe had the highest concentrations, followed by Mg and Si. The results of the AR digestion (Table 5) were used to determine whether a metal was present in macro (>1000 mg kg–1) or trace (<1000 mg kg–1) concentrations. For AR digestion (Table 5), most samples had low concentrations of Si, all samples had high concentrations of Mg and Fe and most had a high concentration of Al.
Sample | Macro metals (CCME) (mg kg–1) | Trace metals (CCME) (mg kg–1) | ||||||||||||||
---|---|---|---|---|---|---|---|---|---|---|---|---|---|---|---|---|
Al | Fe | K | Ca | Mg | Na | Ni (45) | P | Co (40) | Cr (64) | Si | Cu (63) | Mn | V (130) | Zn (250) | ||
MKKNi | n.d. | 23.0 | 21.0 | 33.0 | 2160 | 462 | 11.0 | n.d. | 1.59 | 0.89 | n.d. | 0.85 | 6.62 | 0.50 | 0.40 | |
FPK | 35.0 | 157 | 172 | 1460 | 536 | 190 | 10.0 | 60 | 1.69 | 0.15 | 280 | 1.59 | 8.17 | 1.74 | 1.05 | |
SS | 178 | 2550 | 2.20 | 347 | 212 | 21.0 | 69.0 | n.d. | 9.58 | 1.80 | 1040 | 3.04 | 0.70 | 1.10 | 10.2 | |
GT | 113 | 419 | 52.0 | 1040 | 84.0 | 22.0 | 1.00 | 264 | 1.44 | 0.89 | 82.0 | 6.26 | 7.94 | 1.54 | 0.65 | |
GINi | 2.80 | 289 | 26.0 | 138 | 1260 | 8.00 | 18.0 | n.d. | 1.99 | 0.00 | 129 | 4.39 | 10.5 | 0.75 | 0.90 |
Serpentinised intrusive (GINi), serpentinite (MKKNi), fine processed kimberlite (FPK), gabbro deposit (GT) and nickel smelter granulated slag (SS). n.d. indicates the concentration was below detection limits and CCME indicates Soil quality guidelines for protection of environmental and human health: agricultural, residential–parkland, and commercial–industrial (Canadian Council of Ministers of the Environment 2022).
Sample | Macro metals (CCME) (mg kg–1) | Trace metals (CCME) (mg kg–1) | ||||||||||||||
---|---|---|---|---|---|---|---|---|---|---|---|---|---|---|---|---|
Al | Fe | K | Ca | Mg | Na | Ni (45) | P | Co (40) | Cr (64) | Si | Cu (63) | Mn | V (130) | Zn (250) | ||
MKKNi | 8.00 | 2170 | n.d. | n.d. | 2350 | 423 | 47.0 | n.d. | 5.58 | 64.9 | 145 | 2.90 | n.d. | 2.50 | 1.34 | |
FPK | 97.0 | 709 | 50.0 | n.d. | 1230 | 189 | 23.0 | 37.0 | 5.57 | 1.19 | 287 | 2.98 | 0.60 | 3.98 | 1.00 | |
SS | 284 | 3190 | n.d. | n.d. | 293 | 115 | 68.0 | n.d. | 13.7 | 7.12 | 3350 | 0.00 | n.d. | 3.56 | 2.57 | |
GT | 103 | 1440 | n.d. | n.d. | 61.0 | 73.0 | 1.00 | 145 | 5.57 | 3.58 | 7.00 | 3.18 | n.d. | 6.96 | 2.98 | |
GINi | n.d. | 1220 | n.d. | n.d. | 1350 | n.d. | 20.0 | n.d. | 5.44 | 1.55 | 658 | 0.19 | n.d. | 2.52 | 0.00 |
Serpentinised intrusive (GINi), serpentinite (MKKNi), fine processed kimberlite (FPK), gabbro deposit (GT) and nickel smelter granulated slag (SS). n.d. indicates the concentration was below detection limits and CCME indicates Soil quality guidelines for protection of environmental and human health: agricultural, residential–parkland, commercial, industrial (Canadian Council of Ministers of the Environment 2022).
Sample | Macro metals (CCME) (mg kg–1) | Trace metals (CCME) (mg kg–1) | ||||||||||||||
---|---|---|---|---|---|---|---|---|---|---|---|---|---|---|---|---|
Al | Fe | K | Ca | Mg | Na | Ni (45) | P | Co (40) | Cr (64) | Si | Cu (63) | Mn | V (130) | Zn (250) | ||
MKKNi | 1200 | 35 400 | 25 500 | 1280 | 102 000 | 3600 | 1510 | n.d. | 64.7 | 456 | 206 | 62.8 | 386 | 22.6 | 26.5 | |
FPK | 16 300 | 27 600 | 39 000 | 11 500 | 75 400 | 3100 | 783 | 870 | 59.0 | 130 | n.d. | 72.0 | 369 | 82.0 | 58.0 | |
SS | 10 600 | 233 000 | 501 | 15 300 | 29 500 | 1260 | 4760 | n.d. | 1160 | 268 | 24.0 | 392 | 204 | 71.3 | 423 | |
GT | 14 400 | 27 100 | 1000 | 11 200 | 11 800 | 1770 | 46.2 | 1820 | 34.3 | 65.8 | n.d. | 94.1 | 258 | 145 | 39.1 | |
GINi | 1830 | 47 200 | 227 | 1060 | 128 000 | 432 | 2470 | n.d. | 120 | 110 | n.d. | 157 | 604 | 35.0 | 51.5 |
Serpentinised intrusive (GINi), serpentinite (MKKNi), fine processed kimberlite (FPK), gabbro deposit (GT) and nickel smelter granulated slag (SS). n.d. indicates the concentration was below detection limits and CCME indicates Soil quality guidelines for protection of environmental and human health: agricultural, residential–parkland, commercial, industrial (Canadian Council of Ministers of the Environment 2022).
Changes in pH
At the beginning of the SCW, the pH of the tailings was between 8.4 and 9.7 (Fig. 2). Most pH values remained within this range until week 12, fluctuated and then decreased slightly (Fig. 2). The soil + tailing samples had lower initial pH values than the tailing samples alone (Fig. 2). However, the pH for most of the tailing and soil + tailing samples converged by week 18.
Changes in pH of tailing and soil + tailing samples. Serpentinised intrusive (GINi), serpentinite (MKKNi), fine processed kimberlite (FPK), gabbro deposit (GT) and nickel smelter granulated slag (SS) over the 18-week experiment. Error bars represent standard error.
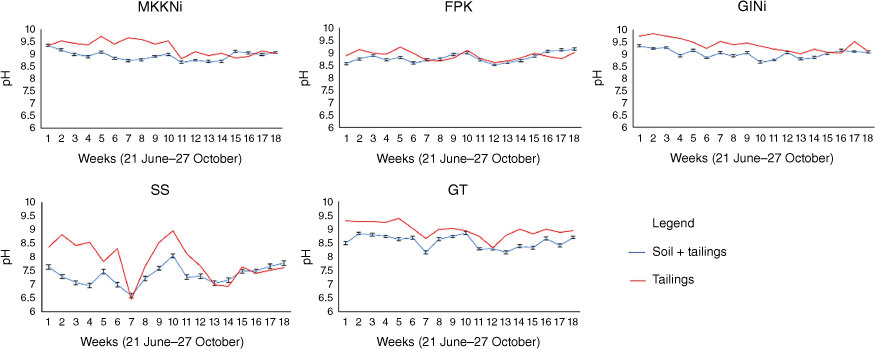
Initial C content was low (0.03–1.37%) and decreased by the end of the SCW in all tailing and soil + tailing samples (0.01–0.68%), except for GINi tailings, which increased by 0.07% (Table 6). The highest C content was found in MKKNi tailings, whereas the lowest was detected in GINi and SS. The soil amendment seemed to increase C content in the soil + tailing samples. The total S content was also low (0.13–0.41%) and seemed to decrease (0.05–0.29%) after the SCW in tailing and soil + tailing samples (Table 6). Total S content was generally higher in the tailing samples compared to the soil + tailing samples. The highest percentage of S was found in GINi and SS tailings, whereas the other tailings had similar amounts.
Tailing sample | Treatment | C (%) | S (%) | |||||
---|---|---|---|---|---|---|---|---|
Week 1 | Week 18 | Change | Week 1 | Week 18 | Change | |||
MKKNi | T | 1.37 | 0.68 | −0.69 | 0.18 | 0.09 | −0.09 | |
S + T | 1.23 | 0.59 | −0.64 | 0.16 | 0.08 | −0.08 | ||
FPK | T | 0.33 | 0.17 | −0.17 | 0.14 | 0.06 | −0.07 | |
S + T | 0.63 | 0.33 | −0.3 | 0.14 | 0.06 | −0.09 | ||
SS | T | 0.03 | 0.01 | −0.02 | 0.36 | 0.17 | −0.19 | |
S + T | 0.34 | 0.24 | −0.10 | 0.28 | 0.10 | −0.17 | ||
GT | T | 0.14 | 0.07 | −0.08 | 0.13 | 0.05 | −0.08 | |
S + T | 0.42 | 0.24 | −0.18 | 0.13 | 0.06 | −0.07 | ||
GINi | T | 0.05 | 0.12 | +0.07 | 0.41 | 0.29 | −0.12 | |
S + T | 0.36 | 0.26 | −0.1 | 0.31 | 0.21 | −0.10 |
Tailing samples were serpentinite (MKKNi), fine processed kimberlite (FPK), nickel smelter granulated slag (SS), gabbro deposit (GT) and serpentinised intrusive (GINi).
The HCl extraction reduced total C and S in all tailings and AAO extractions also reduced S in all tailings (Table 7). HCl extracted a larger proportion of C in MKKNi and FPK (around 90%), and larger proportions of S in MKKNi, FPK and SS (around 60%). Comparing both extractions for total S content, AAO dissolved S more than HCl in general (Table 7).
Tailing sample | C (%) | S (%) | ||||
---|---|---|---|---|---|---|
Before extraction | HCl extraction | Before extraction | HCl extraction | AAO extraction | ||
MKKNi | 1.37 | 0.04 | 0.18 | 0.05 | 0.07 | |
FPK | 0.33 | 0.03 | 0.14 | 0.06 | 0.04 | |
SS | 0.03 | 0.02 | 0.36 | 0.15 | 0.15 | |
GT | 0.14 | n.d. | 0.13 | 0.11 | 0.05 | |
GINi | 0.05 | 0.03 | 0.41 | 0.32 | 0.26 |
Total C was not analysed after AAO extraction. Tailing samples were serpentinite (MKKNi), fine processed kimberlite (FPK), nickel smelter granulated slag (SS), gabbro deposit (GT) and serpentinised intrusive (GINi).
Discussion
Relative chemical stability
Generally, mine tailing minerals gradually break down from primary to secondary minerals according to their relative stability and environmental factors such as temperature, pressure, oxidising conditions and microbial activity (Jackson and Sherman 1953; Hayes et al. 2014). Our results were consistent with Stokreef et al. (2022) who also reported calcite, brucite, hydrotalcite and hydromagnesite being more easily weathered than other secondary minerals in ultramafic silicate minerals.
The metal concentrations from each extraction were consistent with the mineral composition found by the XRD analysis, confirming the stability of the U+M tailings using different methods. Mg carbonates and Mg hydroxides were less stable, with Mg showing high concentrations after the HCl extraction. By contrast, aluminosilicates are relatively stable and were only extracted after AAO extraction and AR digestion. The AAO dissolved more Fe and Al compounds, with higher silica concentrations than the other extractions, and AR extracted almost all minerals, except ‘silicates’ as expected (Evans 2013).
Soil quality guidelines for protection of environmental and human health: agricultural, residential–parkland, commercial, industrial were used to assess any potential harm from the use of tailings (Canadian Council of Ministers of the Environment 2022). The AR extraction found that Ni, Cr and Cu were present in levels above the Canadian Council of Ministers of the Environment guidelines for all tailings, Co was above for most samples except GT, and V and Zn were above guidelines levels for GT and SS respectively (Table 5). The AAO extraction found that Ni was above guideline levels for MKKNi and SS samples, and Cr was above guidelines for MKKNi (Table 4). The HCl extraction found that only Ni was above Canadian Council of Ministers of the Environment guidelines for the SS sample (Table 3). These heavy metals in high concentrations are potential metal leaching sources and could be potential sources of contamination of soil, surface water or groundwater (Concas et al. 2006; Huang et al. 2013). Therefore, using these tailings with soil as an amendment would need further studies to confirm potential risk of contamination.
Effects of wetting and drying (simulated redox) on pH
Not unexpectedly, the chemical composition of the minerals had a major influence on the pH trends of the tailings during the 18-week SCW. The reasonably high initial pH of the tailings suggests that samples contained a high amount of basic cation containing minerals. Kandji et al. (2017) found similar results with pH values ranging between 9 and 10 in a column test for mafic tailings from Dumont Nickel Project (RNC Minerals, Québec, QC, Canada). According to the selective extractions, as predicted, the least stable minerals were calcite, brucite, hydrotalcite and hydromagnesite. These minerals contain both Ca and Mg carbonates and hydroxides, contributing to the relatively higher pH of MKKNi and GiNi. Stokreef et al. (2022) also found that these minerals were less stable in comparison to other common mafic minerals and found that other carbonates, such as magnesite and dolomite, are less reactive. Calcite content may also have contributed to the FPK and GT tailings’ high pH during the SCW. A study by Garcíea-Arreola et al. (2018) on gold and silver mine tailings also reported higher neutralisation capacity in tailings with higher concentrations of calcite.
According to the empirical Goldich sequence, basic minerals are consumed and weathered faster, while acid-generating silicate minerals continue to react more slowly over a long time period (Goldich 1938; Franke and Teschner-Steinhardt 1994). Sulfur is the major contributor to pH changes in mine tailings (Price and Errington 1998). Sulfur containing minerals were not detected in XRD results, but other studies have found that GlNi and SS tailings contained minor amounts of sulfide minerals (Page 1982; Cutts et al. 2020). SS contained relatively lower content of carbonates and two S containing minerals, pentlandite and bornite. As a result, SS tailings exhibited the largest fluctuation and difference in pH between the tailing and soil + tailing samples among the samples studied.
The variability of the minerals in each sample was consistent with the XRD results. GINi and MKKNi had the two highest pH values throughout the SCW, which is consistent with the mineral composition. These tailings were similar among all extractions and were associated with higher levels of Mg and lower Al concentrations. By contrast, SS had the lowest pH average throughout the SCW (Fig. 2), with higher total Fe and Ni concentrations and relatively low total Mg concentrations compared to GINi and MKKNi (Tables 3–5). For the SS sample, Fe and Al were higher in the HCl (Table 3) and AAO (Table 4) extractions than in other tailings, suggesting that the Fe and Al were present in more available forms and could contribute to changes in pH over time. Higher Fe and Al contents could be potential acid-generating components in the tailings, such as in minerals containing Fe (magnetite, serpentine and olivine) and Al (serpentine and clay), by the oxidation of sulfides with Fe and the precipitation of hydroxides and oxides of Al and Fe (Chadwick and Chorover 2001; Hayes et al. 2009).
The use of soil amendment seemed to slightly decrease the pH of the soil + tailing samples for the first 12 weeks of the SCW. However, the pH for most of the tailings and soil + tailings samples converged and became more stable toward week 18. Since the soil had a lower pH, the mix of soil and tailing lowered the initial pH. The soil contained smectite, vermiculite and mica which may have buffered any changes in pH due to their cation exchange capacity. However, this was likely exhausted starting by week 7 in some tailings due to the high basic cation content of the U+M tailings and the reasonably low amount of acid-producing minerals (Table 2). As the U+M tailings weather, the basic cation containing minerals should increase the pH. However, all tailing and soil + tailing samples had a pH above 7 during the 18-week experiment, suggesting there were sufficient basic cations to neutralise acid-generating minerals and that the tailings likely continue to sequester C.
Kelemen et al. (2020) found that U+M tailings were a sink for CO2 when exposed to weathering under atmospheric conditions; however, our results showed a slight reduction in total C and S content for almost all samples except GINi (Table 6). There are several reasons that could help explain the decrease in total C. It may be that an 18-week incubation experiment under normal atmospheric conditions is not enough time for C to become sequestered. Further research is needed to determine the contribution of organic and inorganic C that could account for C sequestration over longer periods of time. However, this study did not differentiate between organic and inorganic C and did not analyse microbial populations, which requires elucidation. Furthermore, it could be that a passivating layer of crystalline nesquehonite was formed on brucite or a silica layer on olivine, which would have impeded C sequestration (Béarat et al. 2006). Using optimal conditions of partial pressure, temperature, CO2 concentration, water availability and grain size have been shown to increase the C sequestration potential of mafic tailings (Stokreef et al. 2022).
Conclusions
Contamination from mine tailings and CO2 emissions from operations are critical environmental issues facing the mining industry. This research provides information about the use of U+M mine tailings as a feedstock for C sequestration and the potential of mineral soil as an amendment in U+M tailing materials. This study assessed the relative chemical stability of minerals among five U+M mine tailings and evaluated the potential environmental impacts, specifically metal release and potential for acid-generating substances, using a SCW in the laboratory.
Sequential extractions and digestion, XRD and ICP-MS results revealed that the elemental variations and the minerals identified were consistent with the Goldich stability series. The total concentration of the heavy metals, including Ni, Cr and Cu, were above the Soil quality guidelines for protection of environmental and human health: agricultural, residential–parkland, commercial, industrial (2022). These U+M tailings are therefore potential sources for metal leaching and require further research to use in soil amendment applications.
In general, the C analysis showed a slight reduction in C content over time, suggesting that 18 weeks may not be a sufficient time period to have C sequestration. Total S was detected in minor concentrations and was associated with increased variability in pH. However, the pH of the five tailings analysed, with and without topsoil, remained above 7 during the SCW. Therefore, the presence of reactive oxides and basic cations in the U+M tailings was neutralising any acid-generating components and likely still supporting C sequestration. Further research is needed to quantify the C sequestration potential with and without varying amounts and types of topsoil, differentiating between organic and inorganic carbon and the assessment of microbial populations, to better address the use of U+M tailings for restoration applications. Future studies should also include XRD analyses of minerals at more regular intervals throughout the experiment or examine leachate from samples to understand how mineralogy affects the pH and C sequestration.
Supplementary material
Supplemental material contains five figures with XRD data. Supplementary material is available online.
Acknowledgements
Sincere appreciation and thanks are extended to Dr Greg Dipple, Professor of Geological Sciences at the University of British Columbia, for his initiation of the research on assessing the challenges for sequestering C by ultramafic/mafic mine tailing with the potential to off-set CO2 on-site and for his scientific contribution and guidance. The authors thank Dr Connor Turvey (Postdoctoral Research Fellow) for the X-ray diffraction (XRD) analysis and interpretation and Maureen Soon (Research Scientist, Lab Manager) for inductively coupled plasma–mass spectrometry (ICP-MS) and carbon (C)–sulfur (S) analysis at the University of British Columbia (UBC), Department of Earth, Ocean and Atmospheric Sciences.
References
Baumeister JL (2012) Chemical weathering of the mafic minerals serpentine and olivine in natural environments. MSc thesis, University of British Columbia, Vancouver, BC, Canada. 10.34917/4332517
Béarat H, Mckelvy MJ, Chizmeshya AVG, Gormley D, Nunez R, Carpenter RW, Squires K, Wolf GH (2006) Carbon sequestration via aqueous olivine mineral carbonation: role of passivating layer formation. Environmental Science & Technology 40, 4802-4808.
| Crossref | Google Scholar | PubMed |
Canadian Council of Ministers of the Environment (2016) Guidance Manual for Environmental Site Characterization in Support of Environmental and Human Health Risk Assessment - Volume 4: Analytical Methods. (CCME) Available at https://ccme.ca/en/res/guidancemanual-environmentalsitecharacterization_vol_4_epn1557.pdf [Verified 20 September 2023]
Canadian Council of Ministers of the Environment (2022) Soil quality guidelines for protection of environmental and human health: agricultural, residential/parkland, commercial, industrial. Available at https://ccme.ca/en/summary-table [Verified 20 April 2022]
Center for Science in Public Participation (2014) The potential for acid mine drainage and other water quality problems at modern copper mines using state-of-the-art prevention, treatment, and mitigation methods. Available at https://www.savetheboundarywaters.org/sites/default/files/attachments/chambers_2014_-_ne_mn_copper_mining_ard_contamination_potential_report_-_csp2_20nov14.pdf [Verified 8 July 2022]
Chadwick OA, Chorover J (2001) The chemistry of pedogenic thresholds. Geoderma 100, 321-353.
| Crossref | Google Scholar |
Chakravarthy C, Chalouati S, Chai YE, Fantucci H, Santos RM (2020) Valorization of kimberlite tailings by carbon capture and utilization (CCU) method. Minerals 10, 611.
| Crossref | Google Scholar |
Chen M, Ma LQ (2001) Comparison of three aqua regia digestion methods for twenty Florida soils. Soil Science Society of America Journal 65, 491-499.
| Crossref | Google Scholar |
Concas A, Ardau C, Cristini A, Zuddas P, Cao G (2006) Mobility of heavy metals from tailings to stream waters in a mining activity contaminated site. Chemosphere 63, 244-253.
| Crossref | Google Scholar | PubMed |
Cruz-Hernández P, Carrero S, Pérez-López R, Fernandez-Martinez A, Lindsay MBJ, Dejoie C, Nieto JM (2019) Influence of As(V) on precipitation and transformation of schwertmannite in acid mine drainage-impacted waters. European Journal of Mineralogy 31(2), 237-245.
| Crossref | Google Scholar |
Cutts JA, Dipple GM, Hart CJR, Milidragovic D (2020) Assessment of the carbon mineralization potential of British Columbia by quantifying the response of physical properties to the alteration of ultramafic rocks (NTS 092H/08, 10, 093K/13, 14, 094C/05, 104I, 104N). In ‘Summary of Activities 2019: Minerals’. pp, 137–144. (Geoscience BC) Available at https://cdn.geosciencebc.com/pdf/SummaryofActivities2019/Minerals/Project%202018-038_Minerals_SOA2019.pdf [Verified 8 July 2022]
Davidson CM, Hursthouse AS, Tognarelli DM, Ure AM, Urquhart GJ (2004) Should acid ammonium oxalate replace hydroxylammonium chloride in step 2 of the revised BCR sequential extraction protocol for soil and sediment? Analytica Chimica Acta 508, 193-199.
| Crossref | Google Scholar |
Dvořáčková H, Dvořáček J, Hueso González P, Vlček V (2022) Effect of different soil amendments on soil buffering capacity. PLoS One 17(2), e0263456.
| Crossref | Google Scholar | PubMed |
Eary LE, Williamson MA (2006) Simulations of the neutralizing capacity of silicate rocks in acid mine drainage environments. Journal American Society of Mining and Reclamation 2, 564-577.
| Crossref | Google Scholar |
Evans E (2013) Effects of weathering on chemical and mineralogical properties of the Mount Polley mine tailings: preliminary implications for long-term ecosystem health. MSc thesis, University of British Columbia, Vancouver, BC, Canada. 10.14288/1.0365937
Fanfani L, Zuddas P, Chessa A (1997) Heavy metals speciation analysis as a tool for studying mine tailings weathering. Journal of Geochemical Exploration 58, 241-248.
| Crossref | Google Scholar |
Feng Y, Wang J, Bai Z, Reading L (2019) Effects of surface coal mining and land reclamation on soil properties: a review. Earth-Science Reviews 191, 12-25.
| Crossref | Google Scholar |
Findlay DC (1969) Origin of the Tulameen ultramafic-gabbro complex, southern British Columbia. Canadian Journal of Earth Sciences 6, 399-425.
| Crossref | Google Scholar |
Franke WA, Teschner-Steinhardt R (1994) An experimental approach to the sequence of the stability of rock-forming minerals towards chemical weathering. Catena 21, 279-290.
| Crossref | Google Scholar |
García-Arreola ME, Flores-Vélez LM, Loredo-Tovías M, Aguillón-Robles A, López-Doncel RA, Cano-Rodríguez I, Soriano-Pérez SH (2018) Assessment of the acid drainage neutralization capacity and the toxic metals lixiviation of tailing from Guanajuato mining district, Mexico. Environmental Earth Sciences 77, 355.
| Crossref | Google Scholar |
Goldich SS (1938) A study in rock-weathering. Journal of Geology 46, 17-58.
| Crossref | Google Scholar |
Hayes SM, White SA, Thompson TL, Maier RM, Chorover J (2009) Changes in lead and zinc lability during weathering-induced acidification of desert mine tailings: coupling chemical and micro-scale analyses. Applied Geochemistry 24, 2234-2245.
| Crossref | Google Scholar | PubMed |
Hayes SM, Root RA, Perdrial N, Maier RM, Chorover J (2014) Surficial weathering of iron sulfide mine tailings under semi-arid climate. Geochimica et Cosmochimica Acta 141, 240-257.
| Crossref | Google Scholar | PubMed |
Huang LM, Deng CB, Huang N, Huang X-J (2013) Multivariate statistical approach to identify heavy metal sources in agricultural soil around an abandoned Pb–Zn mine in Guangxi Zhuang Autonomous Region, China. Environmental Earth Sciences 68, 1331-1348.
| Crossref | Google Scholar |
Jackson ML, Sherman GD (1953) Chemical weathering of minerals in soils. Advances in Agronomy 5, 219-318.
| Crossref | Google Scholar |
Johnson DD, Pilotto D (2018) Gahcho Kué Mine. NI 43–101 Technical Report, NWT, Canada. (JDS Energy & Mining Inc.: Vancouver, BC, Canada) Available at https://filecache.investorroom.com/mr5ircnw_mntprovincetest/2553/download/Gahcho%20Kue%20Mine%20NI%2043-101%20Technical%20Report%20-%20March%201%202020.pdf
Kandji EHB, Plante B, Bussière B, Beaudoin G, Dupont PP (2017) Geochemical behavior of ultramafic waste rocks with carbon sequestration potential: a case study of the Dumont Nickel Project, Amos, Québec. Environmental Science and Pollution Research International 24, 11734-11751.
| Crossref | Google Scholar | PubMed |
Keays RR (1987) Principles of mobilization (dissolution) of metals in mafic and ultramafic rocks – the role of immiscible magmatic sulphides in the generation of hydrothermal gold and volcanogenic massive sulphide deposits. Ore Geology Reviews 2, 47-63.
| Crossref | Google Scholar |
Kefeni KK, Msagati TAM, Mamba BB (2017) Acid mine drainage: prevention, treatment options, and resource recovery: a review. Journal of Cleaner Production 151, 475-493.
| Crossref | Google Scholar |
Kelemen PB, McQueen N, Wilcox J, Renforth P, Dipple G, Vankeuren AP (2020) Engineered carbon mineralization in ultramafic rocks for CO2 removal from air: review and new insights. Chemical Geology 550, 119628.
| Crossref | Google Scholar |
Lu X, Carroll KJ, Turvey CC, Dipple GM (2022) Rate and capacity of cation release from ultramafic mine tailings for carbon capture and storage. Applied Geochemistry 140, 105285.
| Crossref | Google Scholar |
Luttmerding HA (1981) Soils of the Langley–Vancouver Map Area. Volume 3: Description of the Soils, RAB Bulletin 18. (Ministry of Environment: Victoria, BC, Canada) Available at https://www.env.gov.bc.ca/esd/distdata/ecosystems/Soils_Reports/BC15/bc15-v3_report.pdf [Verified 7 July 2022]
McKeague JA, Day JH (1966) Dithionite- and oxalate-extractable Fe and Al as aids in differentiating various classes of soils. Canadian Journal of Soil Science 46, 13-22.
| Crossref | Google Scholar |
Malli H, Timms A, Bouzalakos S (2015) Integration of ultramafic mine tailings and acid mine drainage for carbon sequestration and mine waste management. Journal of Research Projects Review 4, 11-20.
| Google Scholar |
Moncur MC, Smith LJD (2012) Processed kimberlite porewater geochemistry from Diavik Diamond Mines, Inc. In ‘Proceedings from 9th International Conference on Acid Rock Drainage’, 20–26 May 2012, Ottawa, ON, Canada. (Ed. WA Price, C Hogam, G Tremblay) pp. 1196–1307. (Curran Associates, Inc.: Red Hook, NY, USA)
Nixon GT, Rublee VJ (1988) Alaskan-type ultramafic rocks in British Columbia: new concepts of the structure of the Tulameen complex. British Columbia Geological Survey Geological Fieldwork 1987, 281-294.
| Google Scholar |
Page ML (1982) A mineralogical study of nickel mattes from the Kalgoorlie Nickel Smelter, Kalgoorlie, Western Australia. Metallurgical Transactions B 13, 141-152.
| Crossref | Google Scholar |
Power IM, Harrison AL, Dipple GM, Wilson S, Kelemen PB, Hitch M, Southam G (2013) Carbon mineralization: from natural analogues to engineered systems. Reviews in Mineralogy and Geochemistry 77, 305-360.
| Crossref | Google Scholar |
Power IM, Dipple GM, Bradshaw PMD, Harrison AL (2020) Prospects for CO2 mineralization and enhanced weathering of ultramafic mine tailings from the Baptiste nickel deposit in British Columbia, Canada. International Journal of Greenhouse Gas Control 94, 102895.
| Crossref | Google Scholar |
Price WA, Errington JC (1998) Guidelines for metal leaching and acid rock drainage at minesites in British Columbia. (Ministry of Energy and Mines) Available at https://www2.gov.bc.ca/assets/gov/farming-natural-resources-and-industry/mineral-exploration-mining/documents/permitting/ml-ard_guidelines.pdf [Verified 4 July 2022]
Rietveld HM (2014) The Rietveld method. Physica Scripta 89, 098002.
| Crossref | Google Scholar |
Saria L, Shimaoka T, Miyawaki K (2006) Leaching of heavy metals in acid mine drainage. Waste Management & Research 24, 134-140.
| Crossref | Google Scholar | PubMed |
Schippers A, Breuker A, Blazejak A, Bosecker K, Kock D, Wright TL (2010) The biogeochemistry and microbiology of sulfidic mine waste and bioleaching dumps and heaps, and novel Fe(II)-oxidizing bacteria. Hydrometallurgy 104, 342-350.
| Crossref | Google Scholar |
Scott A, Oze C, Shah V, Yang N, Shanks B, Cheeseman C, Marshall A, Watson M (2021) Transformation of abundant magnesium silicate minerals for enhanced CO2 sequestration. Communications Earth & Environment 2, 25.
| Crossref | Google Scholar |
Stokreef S, Sadri F, Stokreef A, Ghahreman A (2022) Mineral carbonation of ultramafic tailings: A review of reaction mechanisms and kinetics, industry case studies, and modelling. Cleaner Engineering and Technology 8, 100491.
| Crossref | Google Scholar |
Weaver RW, Angle JS, Bottomley PJ (2018) ‘Methods of Soil Analysis, Part 2: Microbiological and Biochemical Properties.’ (Eds RW Weaver, S Angle, P Bottomley, D Bezdicek, S Smith, A Tabatabai, A Wollum) (Wiley) 10.2136/sssabookser5.2
Wilson S, Harrison AL, Dipple GM, Power IM, Barker SLL, Ulrich Mayer K, Fallon SJ, Raudsepp M, Southam G (2014) Offsetting of CO2 emissions by air capture in mine tailings at the Mount Keith Nickel Mine, Western Australia: rates, controls and prospects for carbon neutral mining. International Journal of Greenhouse Gas Control 25, 121-140.
| Crossref | Google Scholar |