Characterisation of volatile organic compounds in dingo scat and a comparison with those of the domestic dog
Tyler J. Lepan


A Department of Environment and Genetics, SABE, La Trobe University, Melbourne, Vic. 3086, Australia.
B Proteomics and Metabolomics Platform, School of Agriculture, Biomedicine and Environment, La Trobe University, Melbourne, Vic. 3086, Australia.
C Biosecurity and Animal Welfare, Department of Industry, Trade and Tourism, Northern Territory Government, Darwin, NT, Australia.
D School of Biotechnology and Biomolecular Sciences, University of New South Wales, Sydney, NSW, Australia.
E School of Biosciences, University of Melbourne, Royal Parade, Parkville, Vic. 3052, Australia.
Australian Journal of Zoology 70(5) 142-152 https://doi.org/10.1071/ZO23001
Submitted: 19 January 2023 Accepted: 22 March 2023 Published: 24 May 2023
© 2022 The Author(s) (or their employer(s)). Published by CSIRO Publishing. This is an open access article distributed under the Creative Commons Attribution-NonCommercial-NoDerivatives 4.0 International License (CC BY-NC-ND)
Abstract
Olfaction is a widespread mode of communication in mammals. The volatile organic compounds (VOCs) in biological samples such as scat and urine give them either a repellent property to warn prey or an attractant property to communicate reproductive, social, and territorial status to conspecifics. The aim of this study was to determine whether there are VOC differences in the scat of a dingo compared to that of a domestic German Shepherd Dog (GSD). We standardised the diet for 10 days and then collected scat from 14 dingoes and 11 GSDs. Using headspace solid phase microextraction (HS-SPME) with gas chromatography–mass spectrometry (GC-MS), we characterised VOCs present in the dingo scat and compared the composition with those of GSDs. We identified 58 significant VOCs out of a total of 154 VOCs identified in both dingoes and GSDs. Of these, 12 were significantly higher in the scat of dingoes and 46 elevated in the GSD. Of the 12 elevated in the dingo, three were unique and present in high concentration (>10 ng/g), four were elevated and present in high concentration and five were present at low concentrations (<10 ng/g). We suggest that the detected differences show potential to be incorporated into dingo management strategies.
Keywords: canids, dingo, German Shepherd dog, HS-SPME-GC/MS, native animal management, odours, olfaction, scat, untargeted volatilomics, volatile organic compounds.
Introduction
Olfaction is a critical means of communication in the animal kingdom. Terrestrial animals often deposit scat, urine, and specialised gland secretions to mark their territories, locate prey and communicate with conspecifics (Bradbury 1998; Brennan and Kendrick 2006; Parsons et al. 2018). Predator odours lead to altered activity patterns, behavioural changes and habitat shifts in the prey species (Gittleman 1989; Ache and Young 2005; Apfelbach et al. 2005; Webster et al. 2018). In canids, odours are commonly used to communicate an individual’s social and reproductive status, sex, and age, and inform their presence to the other competing predators (Vogt et al. 2016; McLean et al. 2021). The communication signals are conveyed by scent cues such as sulfur-containing volatiles, hydrocarbons, carboxylic acids, ketones, phenols, and esters released in the scat and urine (Soso and Koziel 2017; Noonan et al. 2019; Jones et al. 2021). A diverse range of these odoriferous compounds, such as aromatic organic compounds, aldehydes, fatty acids and alcohols, have been detected in the scat of wild Iberian wolves (Canis lupus signatus) (Martín et al. 2010). The volatiles (VOCs) identified from African wild dog (Lycaon pictus) urine, scat and gland secretion show a considerable overlap with compounds detected in other mammals. Nevertheless, the chemical profiles markedly vary from other canids (Apps et al. 2012), indicating the usage of some unique population-specific chemicals in olfaction. Understanding the composition of the volatilome in the biological samples is essential to provide insights into olfactory communication between and among species.
The Australian dingo (Canis (familiaris) dingo) went through a population bottleneck when it colonised Australia (Ballard and Wilson 2019). It is the continent’s only indigenous canid and terrestrial top-order predator (Smith et al. 2015). Due to livestock predation, the dingo is subject to lethal control methods (Fleming et al. 2014). However, non-lethal approaches such as barrier fencing are also in use (Smith and Appleby 2018). Like other wild animals, dingoes use scent marking for social communication and territory defence and have been reported to increase scent marking prior to the mating season (Thomson 1992). Dingoes urinate and defaecate on particular sites to communicate with the members of their own and other packs (Thomson 1992). The dingo body odour and scat interfere with the acquisition of food and water by red foxes (Vulpes vulpes) more strongly than conspecific odour (Leo et al. 2015).
Similarly, dingo olfactory scents from urine and scat induce fear-based responses and reduce foraging in the macropod marsupials (Parsons et al. 2007; Parsons and Blumstein 2010). Dingoes are occasionally sympatric with feral dogs (Canis familiaris) and have been shown to respond to the olfactory cues of other canids. For instance, dingoes engage in olfactory communication with the livestock guardian dogs’ (Maremmas’) urine and might recognise territorial boundaries established by guardian dogs (van Bommel and Johnson 2017). The difference in the chemical profile of the predators’ odour can generate a distinct response in the prey species. For instance, Eurasian beavers (Castor fiber) showed reduced foraging in the presence of odours from the wolf (Canis lupus) but not to those from domestic dogs (Rosell and Czech 2000). A comparison of the chemical profile of sympatric predators could provide insights into population-specific olfactory cues.
Wolves, dingoes, and modern-day dog breeds shared a common ancestor and inherited the ability to use olfaction for specific tasks. Domestication and artificial breeding, however, have mainly influenced the utilisation of olfaction in domestic dogs, predominantly in populations bred for enhanced olfactory abilities (Polgár et al. 2016; Jamieson et al. 2017). Domestication may also have reduced the need to efficiently metabolise or absorb nutrients as food is provided regularly. Relaxation of selective constraint since domestication has been found in domestic dogs (Bjornerfeldt et al. 2006; Cruz et al. 2008) and changes in intestinal structure have been observed in mutant mice fed a high fat diet (Lackey et al. 2020).
Along with artificial selection for olfactory traits, canine domestication has been associated with diet change, human-assisted mating, and relaxed environmental pressures. A previous study has shown a significant difference in the plasma metabolome between the dingo and domestic dog breeds and the dingo and dingo–dog hybrid, likely an outcome of the carbohydrate-rich diet in domestic dogs (Yadav et al. 2021). Diet and gut microbiome composition are associated with the faecal VOC composition, as shown in several human studies (De Angelis et al. 2015; Karu et al. 2018). Therefore, the domestic dog may show a distinct composition of the VOCs in the scat compared to the wild canids like dingoes. Nevertheless, studies comparing the VOCs in faeces and urine of the dingo and domestic dog are rare (Robley et al. 2015; Carthey et al. 2017). Such comparisons could detect dingo-specific scent markers with a potential application in the non-lethal management of human–dingo conflict.
Here we compare the dingo and German Shepherd Dog (GSD) volatilome profiles. The GSD was developed 200 years ago (Field et al. 2020) and is one domestic breed that has been extensively used for olfaction-based detection and scent discrimination tasks (Jezierski et al. 2014). The GSD’s large body size translates into a sizeable olfactory epithelium and thus a larger area for sensory neurons (Jamieson et al. 2017), providing it with a benefit for scent detection tasks (Kokocińska-Kusiak et al. 2021). Further, the positive effects of nucleotide substitution in an olfactory receptor gene on the binding affinity of some odours in the sniffer dogs, including GSDs, suggests a genotypic basis of enhanced olfaction (Lesniak et al. 2008).
Bioanalytical techniques can help create volatilome profiles of the VOCs present in the biological sample, even if present in low concentrations. Headspace solid phase microextraction gas chromatography–mass spectrometry (HS-SPME-GC/MS) is currently among the most sensitive and accurate analysis methods (Vas and Vékey 2004). Using HS-SPME-GC/MS, we aim to characterise VOCs in the dingo scat and compare them with those in the domestic GSD. We predict that dingoes will show significant differences in the profile and quantities of the compounds compared with domestic dogs due to a difference in olfactory communication in the natural versus domestic settings and distinct selection pressures.
Data presented here suggest that population-specific chemical cues have the potential to be integrated into current integrated pest management strategies to minimise biodiversity loss and agricultural impact. We detected 12 VOCs that were significantly higher in the dingo scats and 46 in the GSD implying a relaxation of metabolic efficiency in the domestic breed. Of these 12, seven were present in high (>10 ng/g) and five in low concentrations (<10 ng/g). These VOCs have the potential to be ecologically important and may have translational importance in the management of pure dingoes.
Materials and methods
Sample collection
Scat samples were collected once from 14 pure dingoes (seven of each sex) from Dingo Sanctuary Bargo and 11 GSDs (eight females and three males) from Kingvale Kennels in December 2018 (Supplementary Table S1). All animals were healthy with no known signs of illness or disease. No females were in oestrus, and none came into season for at least five weeks after the study was completed.
The purity of all dingoes was tested using the microsatellite marker-based dingo purity genetic test (Wilton 2001). Sanctuary dingoes were either wild born and humanised before six weeks or were sanctuary born. The sanctuary is rural, with Eucalyptus gum trees providing shade. GSDs were registered with the Australian Kennel Club and roamed open grassland with pine trees providing cover. The mean age of dingoes was 4.18 ± 0.61 (s.e.) years, and the mean age of GSDs was 3.7 ± 0.53 (s.e.) years (Table S1). If metabolic efficiency is equal, we predict similar numbers of VOCs will be elevated in the dingo and the GSD. Alternatively, if there has been a relaxation of selective constraint in the GSD, we predict an elevated number of VOCs in the domestic breed.
The individuals were fed with an antibiotic and then a probiotic at the commencement of the study to standardise the microbiome (Field et al. 2022). They were fed chicken, rice and Blackhawk kibble (Masterpet, NSW, Australia) to standardise their diet (Field et al. 2022). This diet was selected as it was the standard diet for Dingo Sanctuary Bargo canids. Fresh untreated rainwater was used in sanctuaries and kennels during the experiment. Scat samples were collected carefully to avoid contamination from soil and plant material. The samples were stored in sterile sealed plastic bags and were immediately stored at −80°C until assayed.
Reagents
High purity (>90%) sodium chloride, nitrobenzene (internal standard), 2-butanone, 2-heptanone, 2-nonanone, 1-octen-3-ol, 2-phenylethanol, benzyl alcohol, delta-valerolactam, methyl salicylate, quinaldine, octanal, acetophenone, benzaldehyde, hexanal, indole, nonyl aldehyde, phenylacetaldehyde, volatile free acids mix and 1000 μg/mL saturated n-alkane mix (C7–C30) were supplied by Sigma-Aldrich (Melbourne, Australia). GC/MS-grade methanol and dichloromethane were purchased from Thermo Fischer (Melbourne, Australia). Individual stock standard solutions (1 mg/mL) were prepared in methanol and stored in the amber glass vials at −20°C and diluted as required for quality control (QC) standard mix (20 mg/L). The n-alkane mix was diluted using dichloromethane (20 mg/L). The saturated sodium chloride solution was freshly prepared by diluting 8 g of NaCl in 20 mL of Milli-Q water.
HS-SPME-GC/MS analysis of all the samples was completed over six consecutive days of batch runs (for example, see Tables S1, S5). The total HS-SPME-GC/MS run time was 126.5 min for each HS vial and 15 vials were run each day. Each day, six selected scat samples were placed in a cooler of ice, homogenised in their bags and promptly weighed (300 mg) into headspace (HS) amber vials (20 mL). A total of 2 mL of the saturated NaCl solution was added to each vial before adding 10 μL of 20 mg/L nitrobenzene (internal standard) and immediately capped with magnetic crimped caps with silicone septa. NaCl solution was added to the samples to enhance their volatility (Peng and Wan 1998) and to instantly quench any enzymatic reactions that may cause changes to the volatilome should the scats have thawed. A bulk sample of dog scat was homogenised and divided into three groups: (1) untreated dog samples (n = 3), (2) treated dog samples with 100 μL of 5M sulfuric acid (Aggarwal et al. 2020) (pH = 2, n = 3), and (3) treated dog samples with 2 mL saturated NaCl solution (n = 3). They were spiked with 10 μL of 20 mg/L VOC standard mix, analysed by HS-SPME-GC/MS, and the resulting peak areas of the standards were compared. The saturated NaCl solution gave the most consistent and reliable results (data not shown). QC-spiked samples and QC-pooled samples were included to account for sample matrix effects and retention time shifts for each HS-SPME-GC/MS batch run. QC-spiked samples were prepared each day in two 20 mL HS vials containing 2 mL NaCl. From here, 50 mg of randomly selected scat from the same batch run was transferred into the designated QC vials and spiked with the QC standards (10 μL of nitrobenzene, n-alkanes, and VOC standard mix (20 mg/L)). QC-pooled samples were prepared using an additional 50 mg of scat from every biological replicate included in a batch run. For the QC-pooled samples, NaCl and nitrobenzene were added to two additional 20 mL HS vials containing the accumulated total of 300 mg of scat per batch run. During the study, we found that the n-alkanes added to the spiked samples were masking the detection of some of the VOCs. As additional controls, 50 mg of scat from all pooled samples were transferred to a separate HS vial containing 2 mL NaCl and stored at −20°C each day. These additional samples were not spiked and thus facilitated the analysis of VOCs masked by the alkanes in the QC-pooled sample sets. To enable background subtractions during data analyses two blanks and two QC laboratory blanks with 2 mL NaCl solution were left uncapped and exposed to the ambient laboratory environment during sample and standard preparation.
HS-SPME-GC/MS analysis
We used a Triplus RHS multipurpose sampler affixed to a Trace 1300 Series gas chromatograph (GC) with a TSQ 8000 Series triple quadrupole mass spectrometer (Thermo Fisher Scientific, Massachusetts, USA). The GC had a split/spitless injector, and the spitless mode was used with a 1.0 mm straight no-wool liner. Following the optimised HS-SPME-GC/MS methodology (from Rivers et al. 2019) with some modifications, scat samples, QC samples, laboratory blanks and standards were block randomised and incubated at 73°C for 15 min with continuous shaking at 350 rpm to release the VOCs into the headspace of the 20 mL HS vial. The VOCs were extracted and adsorbed onto a general-purpose SPME Arrow fibre (1.10 mm DVB/C-WR/PDMS 110 μm × 20 mm, Thermo Scientific) for 40 min at 73°C. Then the fibre was retracted from the vial and promptly inserted into the heated 260°C GC inlet for 10 min for VOC desorption. The SPME Arrow fibre was preconditioned and postconditioned for 10 min in the SPME fibre conditioning module at 230°C before sample extraction and after desorption to prevent carry-over contamination. Chromatographic separation of the VOC analytes was achieved using ultra-high purity helium (Coregas, Australia) as the carrier gas at 1.0 mL/min along in a semistandard non-polar 30 m × 0.25 mm × 0.25 μm Agilent J&W VF-5 ms column, equipped with a 10 m EZ-Guard column. The oven temperature program was initially set at 35°C for 6 min, then ramped at 5°C/min to 150°C and held for 2 min, then ramped to 320°C at 20°C/min and held for 3 min. The total HS-SPME-GC/MS run time was 126.5 min. The GC/MS was operated in full MS scan acquisition using electron impact ionisation (EI) at 70 eV from m/z 35–450 with a solvent delay of 2 min. Temperatures for the aux transfer line was 320°C, the ion source 250°C and the quadrupoles 248°C.
Data analysis
The Gerstel Maestro software (ver. 1.5, Gerstel GmbH & Co. KG, Germany) controlled the Thermo Triplus RSH multipurpose sampler’s operations, and the Thermo Fisher Scientific Xcalibur software (ver. 4.5.) was used for GC/MS data acquisition. Thermo TraceFinder software (ver. 5.1) and FreeStyle (ver. 1.8) were used for data analysis. Statistical analysis was performed using the free online MetaboAnalyst 5.0. The NIST/EPA/NIH Mass Spectral Library (ver. 2020) was used for mass spectral matching (≥70% confidence) and peak annotation. Kovats non-isothermal retention indices (RIs) were calculated for all identified peaks using n-alkanes (C7–C30) and compared against reference semistandard non-polar RIs from the National Institute of Standards and Technology (NIST) and PubChem databases.
For VOC concentration in scat sample relative to the nitrobenzene internal standard:
where X (ng/g) is the VOC concentration in the sample, VOC area is the peak area of the analyte in the sample, Istd area is the peak area of the internal standard in the sample, v is the volume of the HS vial (mL), m is the sample weight (g), and C is the concentration of the internal standard (ng/mL). Note: peak area is unitless.
Results
Untargeted volatilomics of scats from dingoes and German Shepherd dogs
In total, 159 VOCs, including n-alkanes, were detected by HS-SPME-GC/MS (Table S2). Following the set criteria set by the Metabolomics Standards Initiative (MSI), identification confidence levels of these VOCs were established as follows: (1) confirmed identification with standard, NIST library mass spectra and Kovats non-isothermal retention index (RI), (2) identification with NIST reference mass spectra and Kovats RI, or (3) putative NIST reference spectra match score (≥70% confidence). Therefore, QC standards were included in this study to confirm mass spectral matches of metabolic VOCs with similar characteristic mass spectra of mass-to-charge ion fragments. VOCs without authentic reference standards were matched to well-known NIST and PubChem mass spectral databases for identification (≥70% confidence) and peak annotation.
Following exclusion of controls, 154 VOCs were detected, identified, and quantified from the dingo and GSD scats (Table S3). Based on sample type, peak response and detection rate, the quality assurance/quality control (QA/QC) percentage relative standard deviation cut-off for VOCs detected in the pooled (n = 8) samples was ≤40%. Therefore, VOCs detected in these QC samples that were >40% were removed. VOCs in the pooled samples were used to normalise data across datasets for statistical analysis. Background subtractions were carried out on scat samples with VOCs matching those detected in the QC laboratory blanks to remove false positive metabolites. Samples, QC blanks, QC samples, QC standard spiked samples and QC standards were statistically block-randomised to account for possible systemic fluctuations during HS-SPME-GC/MS analysis.
Principal component analysis (PCA) illustrates the robustness of the HS-SPME-GCMS methodology (Fig. 1). The PCA scores plot shows Component 1 captured the most variation (19.8%), suggesting that the GSD highly influenced the metabolomic VOC profile.
Log-transformed principal component analysis of scat metabolome from female and male dingoes and German Shepherd dogs (GSD). Green and red data points represent the male and female dingoes, and purple and blue data points represent the female and male GSDs, respectively. Pink data points represent the QC samples (pooled).
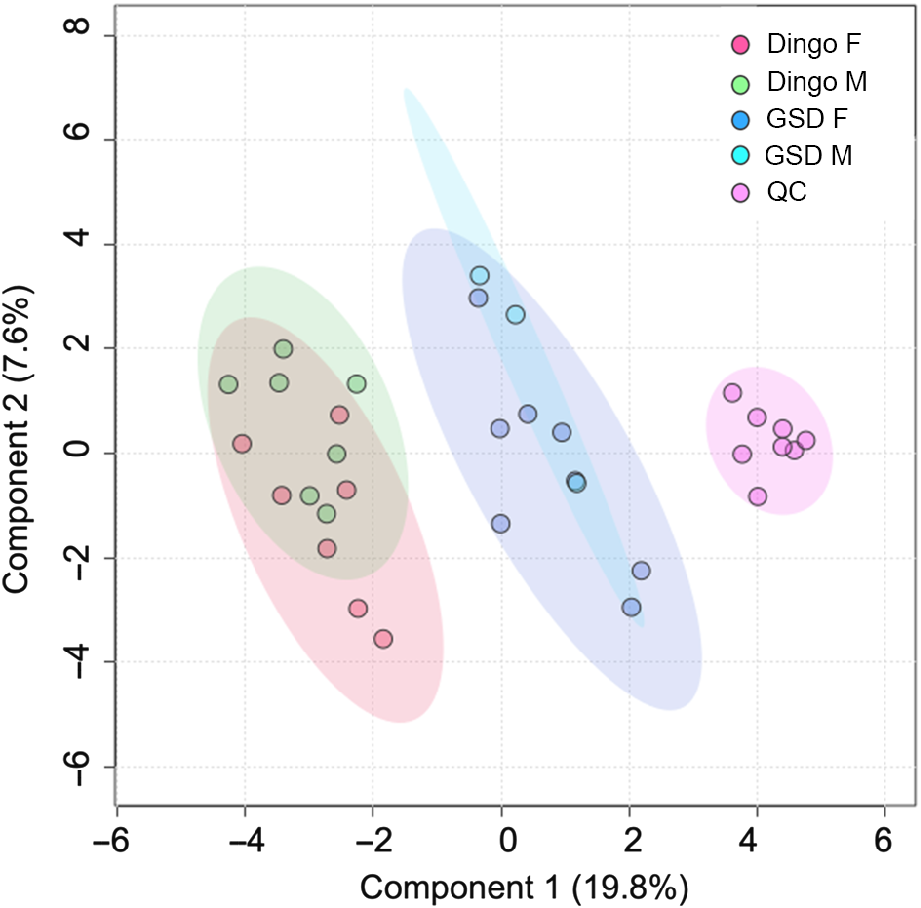
Stack plots of the 154 VOC concentrations show the diverse profiles in the females (dingoes versus GSDs), and males (dingoes versus GSDs) (Fig. 2; Table S3). The VOCs were vastly different in their chemical structures and volatility: aldehydes, ketones, alcohols, hydrocarbons, carboxylic acids, esters, volatile phenols, benzenoids, terpenoids, sulfur-containing compounds and nitrogen-containing compounds, as well as miscellaneous (Table S3).
Stack plot showing the number of metabolic VOCs detected in the males of dingoes (n = 7) versus German Shepherd dogs (GSDs n = 3); and females of dingoes (n = 7) versus GSDs (n = 8); both males and females equate to 154 VOCs detected (Note: 13, 14 and 10 VOCs were found in both dingoes and GSDs for females, males and combined, respectively so they are counted once). However, 147 VOCs were detected in the two collective groups, dingoes (n = 14) and domestic dogs (n = 11), where VOCs with missing values greater than 50% were removed. Blue represents the total number of VOCs showing the highest concentrations. Orange represents the number of unique VOCs detected for each group, and grey indicates the shared VOCs found in both female and male groups.
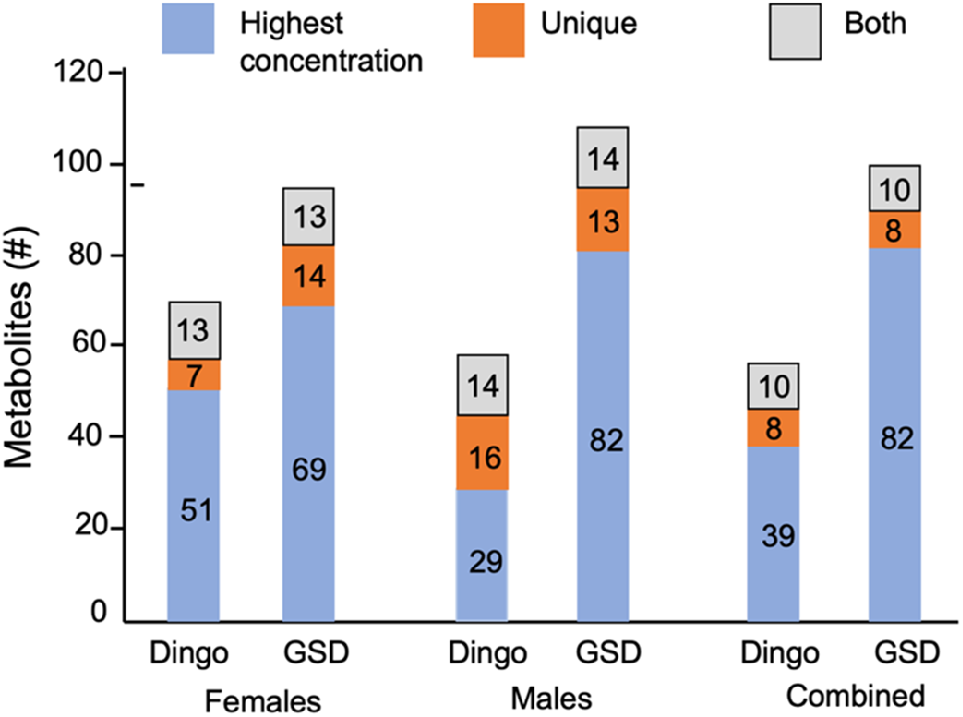
From the 154 VOCs, 58 were significant using univariate statistical analysis based on fold-changes (>1.5; also shown as log2(FC)) and t-tests between dingoes and GSDs. Corresponding false discovery rate (FDR) and P-values less than 0.5 and 0.05, respectively, were also determined (Table 1). Relative concentrations (ng/g) and the relative standard deviations of the VOCs are also shown in Table 1 and divided by sex in Table S4.
Metabolite | P-value (dingo/GSD) | FDR (dingo/GSD) | Fold change (FC) (dingo/GSD) | log2 (FC) (dingo/GSD) | GSD (ng/g, n = 11) | %RSD | Dingo (ng/g, n = 14) | %RSD |
Methyl 2-oxohexadecanoate* | 1.24E-06 | 2.74E-05 | 12.683 | 3.6648 | 2.36 | 66.62 | ||
2,4-Tridecanedione* | 1.13E-05 | 1.34E-04 | 5.0029 | 2.3228 | 27.39 | 40.62 | ||
Benzeneacetic acid* | 1.54E-03 | 1.13E-02 | 3.0696 | 1.6181 | 20.71 | 71.66 | 42.0 | 78.30 |
Mequinol* | 2.63E-03 | 1.84E-02 | 12.386 | 3.6306 | 5.23 | 72.11 | ||
gamma-Eudesmol* | 5.10E-03 | 3.14E-02 | 4.6683 | 2.2229 | 3.72 | 316.08 | ||
3-Octen-2-one* | 6.28E-03 | 3.45E-02 | 1.5582 | 0.6398 | 8.01 | 63.44 | 12.47 | 31.94 |
3-Undecanone* | 9.49E-03 | 5.04E-02 | 4.0761 | 2.0272 | 26.95 | 81.71 | ||
Eucalyptol* | 1.51E-02 | 6.42E-02 | 55.5 | 5.7944 | 41.21 | 198.83 | ||
Mesitaldehyde* | 1.86E-02 | 6.75E-02 | 1.6503 | 0.7227 | 2.92 | 62.70 | ||
Phenylethyl alcohol* | 7.30E-04 | 4.99E-03 | 2.3402 | 0.3693 | 193.96 | 114.01 | 453.93 | 49.64 |
Benzeneacetaldehyde* | 6.80E-05 | 5.40E-04 | 2.7123 | 0.4333 | 21.52 | 49.61 | 58.36 | 39.30 |
Butyl phenylacetate* | 3.33E-02 | 1.05E-01 | 5.2111 | 2.3816 | 2.99 | 58.27 | ||
2,6-Di-tert-butylbenzoquinone | 2.46E-11 | 2.71E-09 | 0.0034 | −8.216 | 140.4 | 94.65 | ||
2-[(2-Ethoxy-3,4-dimethyl-2-cyclohexen-1-ylidene)methyl]furan | 3.52E-11 | 2.71E-09 | 0.0215 | −5.5382 | 4.5 | 95.63 | ||
Acetophenone | 2.48E-08 | 9.66E-07 | 0.0648 | −3.948 | 213.57 | 48.47 | 15.96 | 61.83 |
3,5-ditert-butyl-4-hydroxycyclohexa-2,4-dien-1-one | 2.51E-08 | 9.66E-07 | 0.0718 | −3.7994 | 13.94 | 53.57 | ||
3-Phenylpropanol | 8.20E-08 | 2.53E-06 | 0.0578 | −4.1136 | 128.26 | 110.06 | 7.41 | 107.11 |
4-Formyl-2,6-di-tert-butylphenol | 1.60E-07 | 4.11E-06 | 0.0036 | −8.1153 | 15.36 | 95.84 | ||
2-Methoxy-4-vinylphenol | 2.43E-06 | 4.25E-05 | 0.3819 | −1.3889 | 83.1 | 28.83 | 31.73 | 37.48 |
2-tert-Butyl-4-methoxyphenol | 2.58E-06 | 4.25E-05 | 0.0148 | −6.0817 | 2155.96 | 117.40 | 31.83 | 68.54 |
Benzenepropanal | 2.76E-06 | 4.25E-05 | 0.1794 | −2.4788 | 17.49 | 97.77 | 3.14 | 40.81 |
Khinaldin | 7.19E-06 | 9.66E-05 | 0.1892 | −2.4024 | 1400.92 | 38.51 | 305.79 | 63.93 |
Hydrocinnamic acid | 7.53E-06 | 9.66E-05 | 0.088 | −3.5061 | 583.41 | 69.61 | 55.23 | 76.29 |
Methyl 3-phenylpropanoate | 1.87E-05 | 2.05E-04 | 0.1525 | −2.7136 | 1048.73 | 60.21 | 159.88 | 125.73 |
2,6-Di-tert-butyl-p-cresol | 2.09E-05 | 2.08E-04 | 0.0235 | −5.4111 | 191.7 | 112.08 | 5.17 | 24.57 |
trans-beta-Ionone | 2.16E-05 | 2.08E-04 | 0.3518 | −1.5072 | 25.8 | 63.29 | 9.08 | 27.34 |
3-tert-Butyl-4-methoxyphenol | 2.81E-05 | 2.54E-04 | 0.0004 | −11.316 | 35.6 | 115.94 | ||
Dihydroactinidolide | 3.63E-05 | 3.11E-04 | 0.3067 | −1.7051 | 11.39 | 61.73 | 3.49 | 30.20 |
Pentadecane | 3.76E-04 | 3.05E-03 | 0.2647 | −1.9178 | 16.61 | 53.49 | 5.08 | 68.01 |
2-Ethylhexanol | 1.00E-03 | 7.70E-03 | 0.5857 | −0.7717 | 49.88 | 27.97 | 29.22 | 42.49 |
1-Nonanol | 4.07E-03 | 2.73E-02 | 0.5627 | −0.8295 | 78.30 | 55.63 | 44.06 | 33.14 |
1-Heptanol | 4.78E-03 | 3.07E-02 | 0.4893 | −1.0312 | 23.02 | 46.67 | 12.91 | 34.06 |
3,3-Methylenebis-1H-indole | 5.86E-03 | 3.37E-02 | 0.3251 | −1.621 | 33.33 | 64.58 | 11.63 | 71.04 |
2-Pentadecanone | 5.90E-03 | 3.37E-02 | 0.344 | −1.5395 | 27.88 | 87.17 | 11.09 | 53.25 |
2-Thienaldehyde | 1.13E-02 | 5.78E-02 | 0.6164 | −0.698 | 0.8 | 16.03 | 0.56 | 23.35 |
Heptadecane | 1.23E-02 | 6.10E-02 | 0.4828 | −1.0505 | 16.27 | 57.44 | 9.04 | 42.55 |
Indole | 1.35E-02 | 6.42E-02 | 0.493 | −1.0205 | 22 957.11 | 41.64 | 13 155.1 | 65.31 |
Isocaproic acid | 1.39E-02 | 6.42E-02 | 0.2805 | −1.8338 | 29.4 | 37.62 | ||
Octadecane | 1.54E-02 | 6.42E-02 | 0.4452 | −1.1675 | 5.39 | 68.90 | 2.77 | 67.66 |
2-Heptenal | 1.55E-02 | 6.42E-02 | 0.5383 | −0.8934 | 7.16 | 50.60 | 3.85 | 39.93 |
2-Nonylthiophene | 4.32E-02 | 3.12E-01 | 0.5078 | −0.97769 | 6.14 | 91.93 | ||
Tridecane | 1.60E-02 | 6.42E-02 | 0.187 | −2.4189 | 11.76 | 141.62 | 3.08 | 57.88 |
Hexadecane | 1.63E-02 | 6.42E-02 | 0.4962 | −1.0111 | 6.94 | 63.85 | 4.31 | 60.75 |
2-Tridecanone | 1.63E-02 | 6.42E-02 | 1.5782 | 0.6583 | 29.16 | 33.72 | 26.60 | 46.29 |
2-Piperidinone | 1.70E-02 | 6.42E-02 | 0.5419 | −0.8839 | 1843.31 | 52.74 | 998.88 | 57.74 |
Methanethiol isovalerate | 1.71E-02 | 6.42E-02 | 0.4191 | −1.2545 | 23.14 | 103.31 | 14.89 | 114.88 |
Heptanal | 1.89E-02 | 6.75E-02 | 0.6297 | −0.6672 | 7.81 | 20.38 | 5.63 | 21.74 |
Methyl cis-9-tetradecenoate | 1.93E-02 | 6.75E-02 | 0.1709 | −2.5489 | 4.98 | 78.52 | ||
Diethyltoluamide | 2.39E-02 | 8.17E-02 | 3.1727 | 1.6657 | 1.65 | 53.72 | 5.23 | 82.35 |
5,6-beta-Ionone epoxide | 2.49E-02 | 8.33E-02 | 0.2726 | −1.8752 | 35.33 | 48.19 | 10.58 | 32.71 |
Phenol | 2.60E-02 | 8.53E-02 | 0.4891 | −1.0317 | 2166.57 | 82.75 | 1059.74 | 148.78 |
Tetradecane | 3.27E-02 | 1.05E-01 | 0.1266 | −2.9815 | 4.5 | 140.73 | 0.80 | 71.57 |
Ethylmethylmaleimide | 3.47E-02 | 1.05E-01 | 0.488 | −1.0351 | 91.23 | 69.11 | 44.52 | 51.01 |
1-Hexanol | 3.47E-02 | 1.05E-01 | 0.6177 | −0.695 | 77.36 | 46.18 | 54.71 | 53.47 |
Geranylacetone | 3.60E-02 | 1.07E-01 | 0.6372 | −0.6501 | 42.45 | 62.76 | 27.05 | 26.26 |
2-Decanol | 3.88E-02 | 1.13E-01 | 0.5259 | −0.9273 | 21.97 | 62.28 | 11.55 | 112.86 |
Nonanal | 4.09E-02 | 1.17E-01 | 0.618 | −0.6944 | 18.49 | 35.50 | 13.23 | 36.98 |
p-Cymene | 4.57E-02 | 1.28E-01 | 8.9411 | 3.1604 | 10.04 | 239.6 | 66.66 | 215.16 |
Fold change (FC), log2(FC), false discovery rate (FDR) and P-values and relative concentrations (ng/g) with relative standard deviations (%) are shown for only the 58 statistically significant VOCs. Asterisks denotes VOCs significantly higher in dingoes.
Looking at the fold changes (FC) of the 58 statistically significant VOCs, 12 were significantly higher in the dingoes and 46 in the GSDs (Table 1). We used a Chi-square test to test the hypothesis that the numbers of VOCs that were significantly higher were equal in the dingo and the GSD. This test shows that significantly fewer metabolites were found in the dingo than the GSD (χ2 = 19.93, P < 0.0001), suggesting a relaxation of selective constraint in the domestic breed.
We group the 12 VOCs that were significantly higher into three categories. Category 1 is present in high concentration (>10 ng/g) and unique to the dingo: 2,4-tridecanedione (FC 5.00), 3-undecanone (FC 4.08) and eucalyptol (FC 55.50). The elevation in eucalyptol levels is entirely due to a single individual (Pinchi) but is still considered biologically important as eucalyptol levels were detected only in the female dingoes. With Pinchi included = 41.21 ng/g ± 1.99; without Pinchi = 10.56 ng/g ± 1.23. Category 2 is present in high concentration and significantly elevated in the dingo: phenylethyl alcohol (FC 2.34), benzeneacetic acid (FC 3.07), benzeneacetaldehyde (FC 2.71), octen-2-one (FC 3.07). Category 3 is present in low concentrations (<10 ng/g): mequinol (FC 12.39), methyl 2-oxohexadecanoate (FC 12.68), butyl phenylacetate (FC 5.21), gamma-eudesmol (FC 4.67) and mesitaldehyde (FC 1.65).
For a quick visual overview, the top 48 of the 58 most significant VOCs were represented in the hierarchal cluster analysis (HCA) and heat maps (Fig. 3a, b) created by MetaboAnalyst 5.0. The dingo females and males clustered in the HCA dendrogram, shown as red and green respectively, illustrated a relatively strong discrimination of the dingo volatilome to the GSDs’ females and males (dark blue and light blue clusters) (Fig. 3a). The heat maps show the VOCs for males and females of each population (Fig. 3b).
Log transformed two-way hierarchal cluster analysis (HCA) and heat maps of the metabolic Volatile Organic Compound differences in all the female and male dingo and German Shephard Dog (GSD) samples. The red and blue colour spectrum scale (3 to −3 for the overview heat map and 1 to −1 for the averaged heat map) denotes the intensity of the VOC concentrations, with dark red (crimson) indicating concentrated to dark blue as being non-detected in the sample(s). (a) Overview heat map of the 48 most concentrated VOCs in all samples tested for dingoes (female and male) and GSDs (female and male), and (b) heat map showing the average linkage in the clustering of the mean concentrations of all the female and male dingo samples observed, respectively, likewise for the GSD females and males. For both (a) and (b) heat maps: green and red cluster points represent the male and female dingoes, and purple and blue cluster points represent the female and male GSDs. The dendrograms of hierarchal clustering used the Pearson correlation for the distance measured and the clustering Ward algorithm statistics.
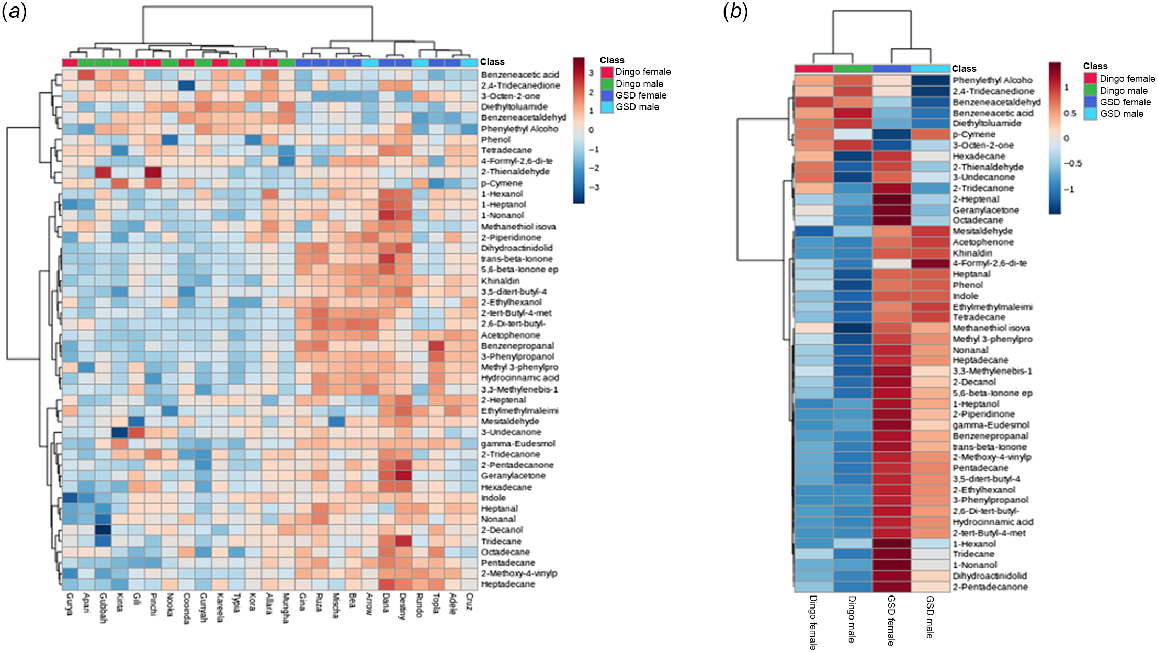
Discussion
The results from scat VOCs data support the hypothesis that there has been a relaxation of selective constraint in GSDs compared to dingoes. We found 12 statistically significant VOCs that were elevated in the dingo scats. In comparison we found 46 VOCs were elevated in the GSD, implying relaxation of metabolic efficiency in the domestic breed. Relaxation of selective constraint in domestic dogs has previously been reported. Bjornerfeldt et al. (2006) sequenced the complete mitochondrial DNA genome in 14 dogs, 6 wolves, and 3 coyotes. They show that domestic dogs have accumulated non-synonymous changes at a faster rate than wolves, leading to elevated levels of variation in their proteins. Cruz et al. (2008) compared variation in dog and wolf genes using whole-genome single nucleotide polymorphism data. The d(N)/d(S) ratio (omega) was approximately 50% greater in dogs than in wolves, indicating that a higher proportion of non-synonymous alleles segregate in dogs, suggesting that slightly deleterious mutations are accumulating in the domesticated dog.
Accumulation of non-synonymous mutations in metabolic genes may influence absorption and digestion of food. Zhang et al. (2020) identified 50 positively selected genes associated with digestion and metabolism, suggesting dietary adaptation in dingoes. Lackey et al. (2020), studied fatty acid binding protein mutant mice and showed marked alterations in intestinal morphology and secretory cell abundance as well as malabsorption of a multitude of nutrients in addition to lipid. One alternate explanation is that differences in the population history of dingoes and GSDs has affected their metabolic efficiency. Dingoes went through a population bottleneck when they arrived in Australia some 5000 year ago whereas GSDs were developed just 200 years ago (Ballard and Wilson 2019; Field et al. 2020).
Of the 12 VOCs that were elevated in the dingo, three were present in high concentrations and were unique to the dingo: 2,4-tridecanedione, 3-undecanone and eucalyptol. Four VOCs were present in high concentrations and upregulated in the dingo compared to the GSD: phenylethyl alcohol, benzeneacetic acid, benzeneacetaldehyde, and octen-2-one. Five VOCs were present in low concentrations (<10 ng/g): mequinol, methyl 2-oxohexadecanoate, gamma-eudesmol, mesitaldehyde, and butyl phenylacetate (Table 1). VOCs are diverse in their chemical structures and volatility and, therefore, are ubiquitously present in mammals. This result suggests that the uniqueness of the scent for olfaction communication is not likely determined by their diet alone. Notable, however, is that multiple VOCs are expected to have antioxidant activity (Gibka et al. 2009; Tanapichatsakul et al. 2018).
As mammals share standard metabolic processes, it is unsurprising that the dingo’s volatile compounds overlapped with other canines’ chemical profiles. The similar chemical composition of the dingo and domestic dog observed in our study is consistent with the observations made in a previous study on five domestic dingoes, two sanctuary dingoes and six domestic dogs (Carthey et al. 2017). The study suggested that native prey can recognise predation risk from domestic dogs due to a similar chemical profile with the dingo, despite only ~150 years of exposure (Frank et al. 2016). A study with dingo urine detected 10 functional groups, including indole, alkenes, phenols, ketones, epoxides, amines, alcohols, alkanes, disulfides, and aldehydes. Of these 10, seven were shared by domestic dogs (Robley et al. 2015). Previous studies comparing the faecal chemical composition of the wild Iberian wolf and domestic dog faeces have also reported a considerable overlap in the chemicals (Arnould et al. 1998; Martín et al. 2010).
There is considerable overlap in the functional groups of compounds present in the scent markings of different canid species and breeds. The majority of the functional groups detected here, such as indole, phenol, ketone, amine, carboxylic acid, alcohols and aldehydes, are commonly present in the urine or scat of other canines such as wolf (Martín et al. 2010), African wild dog (Apps et al. 2012), coyote (Schultz et al. 1988), domestic dog (Schultz et al. 1985; Dzięcioł et al. 2018) and the red fox (McLean et al. 2021). We hypothesise that the relative proportion of biological material within these functional groups confers each species its unique olfactory signals, as shown in other mammal species such as spotted hyenas (Burgener et al. 2009; Liberles 2014). Notably, most canine studies have reported the sulfide group and its derivatives as a dominant functional group. For instance, one-third of the urine VOCs in the red fox (Vulpes vulpes) was odiferous sulfur compounds (McLean et al. 2021). Similarly, the disulfide group was the most abundant compound in the dingo urine (Robley et al. 2015). However, this was not the case in the scat, where most VOCs were aliphatic, consisting of diverse groups of hydrocarbons, ketones, aldehydes, esters, alcohols, and acids.
The VOCs 2,4-tridecanedione, 3-undecanone and eucalyptol were present in high concentrations and unique to the dingo. 2,4-tridecanedione is structurally like 3-undecanone with an additional carbonyl functional group. Thus far, no literature has been reported on 2,4-tridecanedione. Structurally, it is a diketone variant of 2-tridecanone, a plant metabolite with antibacterial (López-Lara et al. 2018) and insecticidal properties (Kimps et al. 2011; Gu et al. 2022). 3-undecanone is a terpene with antimicrobial function (Gibka et al. 2009). It has been determined as an attraction pheromone of the ant Oecophylla longinoda (Francke and Schulz 1999) and the butterfly Heliconius erato (Ehlers et al. 2021). Plausibly, elevated levels of 3-undecanone could be due to the diet, as it is often added to canine kibble (Chen et al. 2017). Eucalyptol, a monoterpenoid, was present in all dingo females and in high concentrations in a single individual. Eucalyptol was first identified in the oils of Eucalyptus globulus by French chemist François Stanislas Cloez in 1870. It constitutes 90% of eucalyptus oil, has been found to have insecticidal and insect-repellent properties, and has also been used in food flavourings, including meat products and baked goods (Boland et al. 1991). Plausibly, elevated levels of eucalyptol in dingo females are due to them chewing gum tree branches in the sanctuary.
Four VOCs were upregulated in the dingo compared to the GSD. Phenylethyl alcohol is an uncommon compound in other canids except for its detection in dog urine and faeces of the African wild dog and the anal gland and faeces of the black-backed jackal (Apps et al. 2012). It is, however, commonly found in the marking fluid of large cats such as the Bengal tiger (Burger et al. 2008), the Siberian tiger (Soso and Koziel 2016) and the African lion (Soso and Koziel 2017). The VOC is an aromatic alcohol and is known to have antimicrobial activity and antibacterial activity (Corre et al. 1990), particularly inhibiting the growth of gram-negative bacteria (Lilley and Brewer 1953). Phenylethyl alcohol is significantly correlated with metabolically active bacterial OTUs (family and genus levels) such as Clostridiaceae and Lactobacillaceae (De Angelis et al. 2015). Phenylethyl alcohol is a by-product of the incomplete microbial degradation of phenylalanine (Dickinson et al. 2003). Due to the involvement of the microbial communities in the production of phenylethyl alcohol, the difference in the gut microbial composition in the dingo and the domestic dog may change the faecal compound composition (Deschamps et al. 2022).
Three additional VOCs were upregulated in the dingo compared with the GSD. Benzeneacetic acid has been found in the anal excretions of the African wild dog (Apps et al. 2012), the anal sacs of wolverines (Wood et al. 2005) and the red fox (McLean et al. 2021). Benzeneacetaldehyde can be derived from phenylalanine and has been found as communication pheromones in insects (El-Sayed 2017). Octen-2-one is one of the many scents added to dry dog kibble (Chen et al. 2017), which could be due to the diet fed.
Five VOCs were present in low concentrations (<10 ng/g) but higher in the dingo than the GSD (Table 1). Mequinol was only found in dingoes and was previously identified in dingo urine (Robley et al. 2015). Gamma-eudesmol was detected in a male GSD and has been found to have bactericidal activity from the essential oil extract of the baby sage Salvia microphylla (Lima et al. 2012). Methyl 2-oxohexadecanoate and butyl phenylacetate are found in dingoes but not GSDs. Mesitaldehyde was also detected in female GSDs.
An additional chemical of interest is khinaldin. It was significantly lower in dingo scat compared to GSDs but has been previously reported in a SPME-GC/MS of the dingo urine under the synonym quinoline, 2-methyl (Robley et al. 2015). It has a distinct odour and is an electron carrier in several pathways. This VOC is also found in the urine of ferrets (Mustela furo) and the anal sac of four skunk species, suggesting its central role in social behaviour (Jorgenson et al. 1978; Apps 2013; McLean et al. 2021). We detected other quinoline derivatives at high levels in dingo and GSD scats. Bacteria can produce quinoline derivatives, and several dihydroxyquinolines have been identified in human faeces (Hubbard et al. 2019). The potential role of bacteria in scent marking cannot be overlooked, as compounds produced in the anal gland and then deposited in the scat would have utility in territorial marking.
Integrated pest management, including all available control measures implemented in a coordinated manner at a landscape scale, is a recommended control method for wild dogs in Australia. These include baiting, trapping, shooting, exclusion fencing and guardian animals. These strategies can adversely affect the regulating impact of herbivory in an ecosystem (Morris and Letnic 2017; Fisher et al. 2021) or production system (Choquenot and Forsyth 2013). This study takes the first step in identifying potential population-specific chemical cues in scat as a step towards supplementing existing strategies with chemical approaches aimed at excluding pure dingoes from agricultural lands.
Data availability
The data that support this study will be shared upon reasonable request to the corresponding author.
Author contributions
JWOB collected the scat samples and TL prepared samples for VOC analysis. TL and TT carried out solid phase microextraction (SPME) gas chromatography–mass spectrometry (GC-MS). TL and TT carried out the GC/MS data analysis. TL conducted the statistical analyses and wrote the initial draft of the manuscript. SY conducted a preliminary analysis that aided this study. All authors read and approved the final version of the manuscript.
Acknowledgements
We thank Dingo Sanctuary Bargo and Kingvale Kennels for providing animals for the study. We thank Martin Bucknall (UNSW) and William Donald (UNSW) for help with a preliminary study. Mass spectrometric results were obtained at the Centre For AgriBioscience (Bundoora, Melbourne).
References
Ache, BW, and Young, JM (2005). Olfaction: diverse species, conserved principles. Neuron 48, 417–430.| Olfaction: diverse species, conserved principles.Crossref | GoogleScholarGoogle Scholar |
Aggarwal, P, Baker, J, Boyd, MT, Coyle, S, Probert, C, and Chapman, EA (2020). Optimisation of urine sample preparation for headspace-solid phase microextraction gas chromatography-mass spectrometry: altering sample ph, sulphuric acid concentration and phase ratio. Metabolites 10, 482.
| Optimisation of urine sample preparation for headspace-solid phase microextraction gas chromatography-mass spectrometry: altering sample ph, sulphuric acid concentration and phase ratio.Crossref | GoogleScholarGoogle Scholar |
Apfelbach, R, Blanchard, CD, Blanchard, RJ, Hayes, RA, and McGregor, IS (2005). The effects of predator odors in mammalian prey species: a review of field and laboratory studies. Neuroscience & Biobehavioral Reviews 29, 1123–1144.
| The effects of predator odors in mammalian prey species: a review of field and laboratory studies.Crossref | GoogleScholarGoogle Scholar |
Apps, PJ (2013). Are mammal olfactory signals hiding right under our noses? Naturwissenschaften 100, 487–506.
| Are mammal olfactory signals hiding right under our noses?Crossref | GoogleScholarGoogle Scholar |
Apps, P, Mmualefe, L, and McNutt, JW (2012). Identification of volatiles from the secretions and excretions of African wild dogs (Lycaon pictus). Journal of Chemical Ecology 38, 1450–1461.
| Identification of volatiles from the secretions and excretions of African wild dogs (Lycaon pictus).Crossref | GoogleScholarGoogle Scholar |
Arnould, C, Malosse, C, Signoret, J-P, and Descoins, C (1998). Which chemical constituents from dog feces are involved in its food repellent effect in sheep? Journal of Chemical Ecology 24, 559–576.
| Which chemical constituents from dog feces are involved in its food repellent effect in sheep?Crossref | GoogleScholarGoogle Scholar |
Ballard, JWO, and Wilson, LAB (2019). The Australian dingo: untamed or feral? Frontiers in Zoology 16, 2.
| The Australian dingo: untamed or feral?Crossref | GoogleScholarGoogle Scholar |
Bjornerfeldt, S, Webster, MT, and Vila, C (2006). Relaxation of selective constraint on dog mitochondrial DNA following domestication. Genome Research 16, 990–994.
| Relaxation of selective constraint on dog mitochondrial DNA following domestication.Crossref | GoogleScholarGoogle Scholar |
Boland DJ, Brophy JJ, House APN (1991) ‘Eucalyptus leaf oils, use, chemistry, distillation and marketing.’ p. 252. (Inkata Press: Melbourne)
Bradbury JW (1998) ‘Principles of animal communication.’ (Sinauer Associates: Sunderland, MA)
Brennan, PA, and Kendrick, KM (2006). Mammalian social odours: attraction and individual recognition. Philosophical Transactions of the Royal Society B: Biological Sciences 361, 2061–2078.
| Mammalian social odours: attraction and individual recognition.Crossref | GoogleScholarGoogle Scholar |
Burgener, N, Dehnhard, M, Hofer, H, and East, ML (2009). Does anal gland scent signal identity in the spotted hyaena? Animal Behaviour 77, 707–715.
| Does anal gland scent signal identity in the spotted hyaena?Crossref | GoogleScholarGoogle Scholar |
Burger, BV, Viviers, MZ, Bekker, JPI, le Roux, M, Fish, N, Fourie, WB, and Weibchen, G (2008). Chemical characterization of territorial marking fluid of male Bengal tiger, Panthera tigris. Journal of Chemical Ecology 34, 659–671.
| Chemical characterization of territorial marking fluid of male Bengal tiger, Panthera tigris.Crossref | GoogleScholarGoogle Scholar |
Carthey, AJR, Bucknall, MP, Wierucka, K, and Banks, PB (2017). Novel predators emit novel cues: a mechanism for prey naivety towards alien predators. Scientific Reports 7, 16377.
| Novel predators emit novel cues: a mechanism for prey naivety towards alien predators.Crossref | GoogleScholarGoogle Scholar |
Chen, M, Chen, X, Nsor-Atindana, J, Masamba, KG, Ma, J, and Zhong, F (2017). Optimization of key aroma compounds for dog food attractant. Animal Feed Science and Technology 225, 173–181.
| Optimization of key aroma compounds for dog food attractant.Crossref | GoogleScholarGoogle Scholar |
Choquenot, D, and Forsyth, DM (2013). Exploitation ecosystems and trophic cascades in non-equilibrium systems: pasture – red kangaroo – dingo interactions in arid Australia. Oikos 122, 1292–1306.
| Exploitation ecosystems and trophic cascades in non-equilibrium systems: pasture – red kangaroo – dingo interactions in arid Australia.Crossref | GoogleScholarGoogle Scholar |
Corre, J, Lucchini, JJ, Mercier, GM, and Cremieux, A (1990). Antibacterial activity of phenethyl alcohol and resulting membrane alterations. Research in Microbiology 141, 483–497.
| Antibacterial activity of phenethyl alcohol and resulting membrane alterations.Crossref | GoogleScholarGoogle Scholar |
Cruz, F, Vila, C, and Webster, MT (2008). The legacy of domestication: accumulation of deleterious mutations in the dog genome. Molecular Biology and Evolution 25, 2331–2336.
| The legacy of domestication: accumulation of deleterious mutations in the dog genome.Crossref | GoogleScholarGoogle Scholar |
De Angelis, M, Montemurno, E, Vannini, L, Cosola, C, Cavallo, N, Gozzi, G, Maranzano, V, Di Cagno, R, Gobbetti, M, and Gesualdo, L (2015). Effect of whole-grain barley on the human fecal microbiota and metabolome. Applied and Environmental Microbiology 81, 7945–7956.
| Effect of whole-grain barley on the human fecal microbiota and metabolome.Crossref | GoogleScholarGoogle Scholar |
Deschamps, C, Humbert, D, Zentek, J, Denis, S, Priymenko, N, Apper, E, and Blanquet-Diot, S (2022). From Chihuahua to Saint-Bernard: how did digestion and microbiota evolve with dog sizes. International Journal of Biological Sciences 18, 5086–5102.
| From Chihuahua to Saint-Bernard: how did digestion and microbiota evolve with dog sizes.Crossref | GoogleScholarGoogle Scholar |
Dickinson, JR, Salgado, LEJ, and Hewlins, MJE (2003). The catabolism of amino acids to long chain and complex alcohols in Saccharomyces cerevisiae. Journal of Biological Chemistry 278, 8028–8034.
| The catabolism of amino acids to long chain and complex alcohols in Saccharomyces cerevisiae.Crossref | GoogleScholarGoogle Scholar |
Dzięcioł, M, Woszczylo, M, Szumny, A, Jezierski, T, Kupczyński, R, Godzińska, EJ, Pieczewska, B, and Niżański, W (2018). Identification of putative volatile sex pheromones in female domestic dogs (Canis familiaris). Animal Reproduction Science 197, 87–92.
| Identification of putative volatile sex pheromones in female domestic dogs (Canis familiaris).Crossref | GoogleScholarGoogle Scholar |
Ehlers, S, Szczerbowski, D, Harig, T, Stell, M, Hötling, S, Darragh, K, Jiggins, CD, and Schulz, S (2021). Identification and composition of clasper scent gland components of the butterfly Heliconius erato and its relation to mimicry. ChemBioChem 22, 3300–3313.
| Identification and composition of clasper scent gland components of the butterfly Heliconius erato and its relation to mimicry.Crossref | GoogleScholarGoogle Scholar |
El-Sayed AM (2017) ‘The pherobase: database of insect pheromones and semiochemicals. Vol. 2022.’ (Ashraf M. El-Sayed)
Field, MA, Rosen, BD, Dudchenko, O, Chan, EKF, Minoche, AE, Edwards, RJ, Barton, K, Lyons, RJ, Tuipulotu, DE, Hayes, VM, D. Omer, A, Colaric, Z, Keilwagen, J, Skvortsova, K, Bogdanovic, O, Smith, MA, Aiden, EL, Smith, TPL, Zammit, RA, and Ballard, JWO (2020). Canfam_GSD: De novo chromosome-length genome assembly of the German Shepherd Dog (Canis lupus familiaris) using a combination of long reads, optical mapping, and Hi-C. GigaScience 9, .
| Canfam_GSD: De novo chromosome-length genome assembly of the German Shepherd Dog (Canis lupus familiaris) using a combination of long reads, optical mapping, and Hi-C.Crossref | GoogleScholarGoogle Scholar |
Field, MA, Yadav, S, Dudchenko, O, Esvaran, M, Rosen, BD, Skvortsova, K, Edwards, RJ, Keilwagen, J, Cochran, BJ, Manandhar, B, Bustamante, S, Rasmussen, JA, Melvin, RG, Chernoff, B, Omer, A, Colaric, Z, Chan, EKF, Minoche, AE, Smith, TPL, Gilbert, MTP, Bogdanovic, O, Zammit, RA, Thomas, T, Aiden, EL, and Ballard, JWO (2022). The Australian dingo is an early offshoot of modern breed dogs. Science Advances 8, eabm5944.
| The Australian dingo is an early offshoot of modern breed dogs.Crossref | GoogleScholarGoogle Scholar |
Fisher, AG, Mills, CH, Lyons, M, Cornwell, WK, and Letnic, M (2021). Remote sensing of trophic cascades: multi-temporal landsat imagery reveals vegetation change driven by the removal of an apex predator. Landscape Ecology 36, 1341–1358.
| Remote sensing of trophic cascades: multi-temporal landsat imagery reveals vegetation change driven by the removal of an apex predator.Crossref | GoogleScholarGoogle Scholar |
Fleming PJS, Allen BL, Allen LR, Ballard G, Bengsen AJ, Gentle MN, McLeod LJ, Meek PD, Saunders GR (2014) Management of wild canids in Australia: free-ranging dogs and red foxes. In ‘Carnivores of Australia: past, present and future’. (Eds AS Glen, CR Dickman) pp. 105–149. (CSIRO Publishing)
Francke W, Schulz S (1999) Miscellaneous natural products including marine natural products, pheromones, plant hormones, and aspects of ecology. In ‘Comprehensive natural products chemistry. Vol. 8.04’. (Eds D Barton, K Nakanishi, O Meth-Cohn) pp. 197–261. (Elsevier Science: Amsterdam)
Frank, ASK, Carthey, AJR, and Banks, PB (2016). Does historical coexistence with dingoes explain current avoidance of domestic dogs? Island bandicoots are naïve to dogs, unlike their mainland counterparts. PLoS ONE 11, e0161447.
| Does historical coexistence with dingoes explain current avoidance of domestic dogs? Island bandicoots are naïve to dogs, unlike their mainland counterparts.Crossref | GoogleScholarGoogle Scholar |
Gibka, J, Kunicka-Styczyńska, A, and Gliński, M (2009). Antimicrobial activity of undecan-3-one, undecan-3-ol and undec-3-yl acetate. Experimental Immunology 34, 154–157.
Gittleman JL (1989) ‘Carnivore behavior, ecology, and evolution.’ (Comstock Pub. Associates: Ithaca)
Gu, M, Xue, Z, Lv, S, Cai, Y, Zhang, L, and Gao, X (2022). Corynebacterium sp. 2-TD mediated toxicity of 2-tridecanone to Helicoverpa armigera. Toxins 14, 698.
| Corynebacterium sp. 2-TD mediated toxicity of 2-tridecanone to Helicoverpa armigera.Crossref | GoogleScholarGoogle Scholar |
Hubbard, TD, Liu, Q, Murray, IA, Dong, F, Miller, C, Smith, PB, Gowda, K, Lin, JM, Amin, S, Patterson, AD, and Perdew, GH (2019). Microbiota metabolism promotes synthesis of the human ah receptor agonist 2,8-dihydroxyquinoline. Journal of Proteome Research 18, 1715–1724.
| Microbiota metabolism promotes synthesis of the human ah receptor agonist 2,8-dihydroxyquinoline.Crossref | GoogleScholarGoogle Scholar |
Jamieson, LTJ, Baxter, GS, and Murray, PJ (2017). Identifying suitable detection dogs. Applied Animal Behaviour Science 195, 1–7.
| Identifying suitable detection dogs.Crossref | GoogleScholarGoogle Scholar |
Jezierski, T, Adamkiewicz, E, Walczak, M, Sobczyńska, M, Górecka-Bruzda, A, Ensminger, J, and Papet, E (2014). Efficacy of drug detection by fully-trained police dogs varies by breed, training level, type of drug and search environment. Forensic Science International 237, 112–118.
| Efficacy of drug detection by fully-trained police dogs varies by breed, training level, type of drug and search environment.Crossref | GoogleScholarGoogle Scholar |
Jones, MK, Huff, TB, Freeman, EW, and Songsasen, N (2021). Differential expression of urinary volatile organic compounds by sex, male reproductive status, and pairing status in the maned wolf (Chrysocyon brachyurus). PLoS ONE 16, e0256388.
| Differential expression of urinary volatile organic compounds by sex, male reproductive status, and pairing status in the maned wolf (Chrysocyon brachyurus).Crossref | GoogleScholarGoogle Scholar |
Jorgenson, JW, Novotny, M, Carmack, M, Copland, GB, Wilson, SR, Katona, S, and Whitten, WK (1978). Chemical scent constituents in the urine of the red fox (Vulpes vulpes L.) during the winter season. Science 199, 796–798.
| Chemical scent constituents in the urine of the red fox (Vulpes vulpes L.) during the winter season.Crossref | GoogleScholarGoogle Scholar |
Karu, N, Deng, L, Slae, M, Guo, AC, Sajed, T, Huynh, H, Wine, E, and Wishart, DS (2018). A review on human fecal metabolomics: methods, applications and the human fecal metabolome database. Analytica Chimica Acta 1030, 1–24.
| A review on human fecal metabolomics: methods, applications and the human fecal metabolome database.Crossref | GoogleScholarGoogle Scholar |
Kimps, NW, Bissinger, BW, Apperson, CS, Sonenshine, DE, and Roe, RM (2011). First report of the repellency of 2-tridecanone against ticks. Medical and Veterinary Entomology 25, 202–208.
| First report of the repellency of 2-tridecanone against ticks.Crossref | GoogleScholarGoogle Scholar |
Kokocińska-Kusiak, A, Woszczyło, M, Zybala, M, Maciocha, J, Barłowska, K, and Dzięcioł, M (2021). Canine olfaction: physiology, behavior, and possibilities for practical applications. Animals 11, 2463.
| Canine olfaction: physiology, behavior, and possibilities for practical applications.Crossref | GoogleScholarGoogle Scholar |
Lackey, AI, Chen, T, Zhou, YX, Bottasso Arias, NM, Doran, JM, Zacharisen, SM, Gajda, AM, Jonsson, WO, Corsico, B, Anthony, TG, Joseph, LB, and Storch, J (2020). Mechanisms underlying reduced weight gain in intestinal fatty acid-binding protein (IFABP) null mice. American Journal of Physiology – Gastrointestinal and Liver Physiology 318, G518–G530.
| Mechanisms underlying reduced weight gain in intestinal fatty acid-binding protein (IFABP) null mice.Crossref | GoogleScholarGoogle Scholar |
Leo, V, Reading, RP, and Letnic, M (2015). Interference competition: odours of an apex predator and conspecifics influence resource acquisition by red foxes. Oecologia 179, 1033–1040.
| Interference competition: odours of an apex predator and conspecifics influence resource acquisition by red foxes.Crossref | GoogleScholarGoogle Scholar |
Lesniak, A, Walczak, M, Jezierski, T, Sacharczuk, M, Gawkowski, M, and Jaszczak, K (2008). Canine olfactory receptor gene polymorphism and its relation to odor detection performance by sniffer dogs. Journal of Heredity 99, 518–527.
| Canine olfactory receptor gene polymorphism and its relation to odor detection performance by sniffer dogs.Crossref | GoogleScholarGoogle Scholar |
Liberles, SD (2014). Mammalian pheromones. Annual Review of Physiology 76, 151–175.
| Mammalian pheromones.Crossref | GoogleScholarGoogle Scholar |
Lilley, BD, and Brewer, JH (1953). The selective antibacterial action of phenylethyl alcohol. Journal of the American Pharmaceutical Association (Scientific ed.) 42, 6–8.
| The selective antibacterial action of phenylethyl alcohol.Crossref | GoogleScholarGoogle Scholar |
Lima, RK, Cardoso, MdG, Andrade, MA, Guimarães, PL, Batista, LR, and Nelson, DL (2012). Bactericidal and antioxidant activity of essential oils from Myristica fragrans Houtt and Salvia microphylla H.B.K. Journal of the American Oil Chemists’ Society 89, 523–528.
| Bactericidal and antioxidant activity of essential oils from Myristica fragrans Houtt and Salvia microphylla H.B.K.Crossref | GoogleScholarGoogle Scholar |
López-Lara, IM, Nogales, J, Pech-Canul, Á, Calatrava-Morales, N, Bernabéu-Roda, LM, Durán, P, Cuéllar, V, Olivares, J, Alvarez, L, Palenzuela-Bretones, D, Romero, M, Heeb, S, Cámara, M, Geiger, O, and Soto, MJ (2018). 2-Tridecanone impacts surface-associated bacterial behaviours and hinders plant–bacteria interactions. Environmental Microbiology 20, 2049–2065.
| 2-Tridecanone impacts surface-associated bacterial behaviours and hinders plant–bacteria interactions.Crossref | GoogleScholarGoogle Scholar |
Martín, J, Barja, I, and López, P (2010). Chemical scent constituents in feces of wild Iberian wolves (Canis lupus signatus). Biochemical Systematics and Ecology 38, 1096–1102.
| Chemical scent constituents in feces of wild Iberian wolves (Canis lupus signatus).Crossref | GoogleScholarGoogle Scholar |
McLean, S, Nichols, DS, and Davies, NW (2021). Volatile scent chemicals in the urine of the red fox, Vulpes vulpes. PLoS ONE 16, e0248961.
| Volatile scent chemicals in the urine of the red fox, Vulpes vulpes.Crossref | GoogleScholarGoogle Scholar |
Morris, T, and Letnic, M (2017). Removal of an apex predator initiates a trophic cascade that extends from herbivores to vegetation and the soil nutrient pool. Proceedings of the Royal Society B: Biological Sciences 284, 20170111–20170111.
Noonan, MJ, Tinnesand, HV, Müller, CT, Rosell, F, Macdonald, DW, and Buesching, CD (2019). Knowing me, knowing you: anal gland secretion of european badgers (Meles meles) codes for individuality, sex and social group membership. Journal of Chemical Ecology 45, 823–837.
| Knowing me, knowing you: anal gland secretion of european badgers (Meles meles) codes for individuality, sex and social group membership.Crossref | GoogleScholarGoogle Scholar |
Parsons, MH, and Blumstein, DT (2010). Familiarity breeds contempt: kangaroos persistently avoid areas with experimentally deployed dingo scents. PLoS ONE 5, e10403.
| Familiarity breeds contempt: kangaroos persistently avoid areas with experimentally deployed dingo scents.Crossref | GoogleScholarGoogle Scholar |
Parsons, MH, Lamont, BB, Kovacs, BR, and Davies, SJJF (2007). Effects of novel and historic predator urines on semi-wild western grey kangaroos. Journal of Wildlife Management 71, 1225–1228.
| Effects of novel and historic predator urines on semi-wild western grey kangaroos.Crossref | GoogleScholarGoogle Scholar |
Parsons, MH, Apfelbach, R, Banks, PB, Cameron, EZ, Dickman, CR, Frank, ASK, Jones, ME, McGregor, IS, McLean, S, Müller-Schwarze, D, Sparrow, EE, and Blumstein, DT (2018). Biologically meaningful scents: a framework for understanding predator–prey research across disciplines. Biological Reviews 93, 98–114.
| Biologically meaningful scents: a framework for understanding predator–prey research across disciplines.Crossref | GoogleScholarGoogle Scholar |
Peng, J, and Wan, A (1998). Effect of ionic strength on Henry’s constants of volatile organic compound. Chemosphere 36, 2731–2740.
| Effect of ionic strength on Henry’s constants of volatile organic compound.Crossref | GoogleScholarGoogle Scholar |
Polgár, Z, Kinnunen, M, Újváry, D, Miklósi, Á, and Gácsi, M (2016). A test of canine olfactory capacity: comparing various dog breeds and wolves in a natural detection task. PLoS ONE 11, e0154087.
| A test of canine olfactory capacity: comparing various dog breeds and wolves in a natural detection task.Crossref | GoogleScholarGoogle Scholar |
Rivers, JY, Truong, TT, Pogson, BJ, and McQuinn, RP (2019). Volatile apocarotenoid discovery and quantification in Arabidopsis thaliana: optimized sensitive analysis via HS-SPME-GC/MS. Metabolomics 15, 79.
| Volatile apocarotenoid discovery and quantification in Arabidopsis thaliana: optimized sensitive analysis via HS-SPME-GC/MS.Crossref | GoogleScholarGoogle Scholar |
Robley A, Lindeman M, Cook I, Woodford L, Moloney P (2015) Dingo semiochemicals: towards a non-lethal control tool for the management of dingoes and wild dogs in Australia. Arthur Rylah Institute for Environmental Research.
Rosell, F, and Czech, A (2000). Responses of foraging Eurasian beavers Castor fiber to predator odours. Wildlife Biology 6, 13–21.
| Responses of foraging Eurasian beavers Castor fiber to predator odours.Crossref | GoogleScholarGoogle Scholar |
Schultz, TH, Kruse, SM, and Flath, RA (1985). Some volatile constituents of female dog urine. Journal of Chemical Ecology 11, 169–175.
| Some volatile constituents of female dog urine.Crossref | GoogleScholarGoogle Scholar |
Schultz, TH, Flath, RA, Stern, DJ, Mon, TR, Teranishi, R, Kruse, SM, Butler, B, and Howard, WE (1988). Coyote estrous urine volatiles. Journal of Chemical Ecology 14, 701–712.
| Coyote estrous urine volatiles.Crossref | GoogleScholarGoogle Scholar |
Smith, BP, and Appleby, RG (2018). Promoting human–dingo co-existence in Australia: moving towards more innovative methods of protecting livestock rather than killing dingoes (Canis dingo). Wildlife Research 45, 1–15.
| Promoting human–dingo co-existence in Australia: moving towards more innovative methods of protecting livestock rather than killing dingoes (Canis dingo).Crossref | GoogleScholarGoogle Scholar |
Smith B, Appleby R, Kelly J (2015) ‘The dingo debate: origins, behaviour and conservation.’ (CSIRO Publishing: Clayton South, Australia)
Soso, SB, and Koziel, JA (2016). Analysis of odorants in marking fluid of siberian tiger (Panthera tigris altaica) using simultaneous sensory and chemical analysis with headspace solid-phase microextraction and multidimensional gas chromatography-mass spectrometry-olfactometry. Molecules 21, 834.
| Analysis of odorants in marking fluid of siberian tiger (Panthera tigris altaica) using simultaneous sensory and chemical analysis with headspace solid-phase microextraction and multidimensional gas chromatography-mass spectrometry-olfactometry.Crossref | GoogleScholarGoogle Scholar |
Soso, SB, and Koziel, JA (2017). Characterizing the scent and chemical composition of Panthera leo marking fluid using solid-phase microextraction and multidimensional gas chromatography-mass spectrometry-olfactometry. Scientific Reports 7, 5137.
| Characterizing the scent and chemical composition of Panthera leo marking fluid using solid-phase microextraction and multidimensional gas chromatography-mass spectrometry-olfactometry.Crossref | GoogleScholarGoogle Scholar |
Tanapichatsakul, C, Monggoot, S, Gentekaki, E, and Pripdeevech, P (2018). Antibacterial and antioxidant metabolites of Diaporthe spp. Isolated from flowers of Melodorum fruticosum. Current Microbiology 75, 476–483.
| Antibacterial and antioxidant metabolites of Diaporthe spp. Isolated from flowers of Melodorum fruticosum.Crossref | GoogleScholarGoogle Scholar |
Thomson, PC (1992). The behavioural ecology of dingoes in north-western Australia. II. Activity patterns, breeding season and pup rearing. Wildlife Research 19, 519–529.
| The behavioural ecology of dingoes in north-western Australia. II. Activity patterns, breeding season and pup rearing.Crossref | GoogleScholarGoogle Scholar |
van Bommel, L, and Johnson, CN (2017). Olfactory communication to protect livestock: dingo response to urine marks of livestock guardian dogs. Australian Mammalogy 39, 219–226.
| Olfactory communication to protect livestock: dingo response to urine marks of livestock guardian dogs.Crossref | GoogleScholarGoogle Scholar |
Vas, G, and Vékey, K (2004). Solid-phase microextraction: a powerful sample preparation tool prior to mass spectrometric analysis. Journal of Mass Spectrometry 39, 233–254.
| Solid-phase microextraction: a powerful sample preparation tool prior to mass spectrometric analysis.Crossref | GoogleScholarGoogle Scholar |
Vogt, K, Boos, S, Breitenmoser, U, and Kölliker, M (2016). Chemical composition of Eurasian lynx urine conveys information on reproductive state, individual identity, and urine age. Chemoecology 26, 205–217.
| Chemical composition of Eurasian lynx urine conveys information on reproductive state, individual identity, and urine age.Crossref | GoogleScholarGoogle Scholar |
Webster, C, Massaro, M, Michael, DR, Bambrick, D, Riley, JL, and Nimmo, DG (2018). Native reptiles alter their foraging in the presence of the olfactory cues of invasive mammalian predators. Royal Society Open Science 5, 180136–180136.
| Native reptiles alter their foraging in the presence of the olfactory cues of invasive mammalian predators.Crossref | GoogleScholarGoogle Scholar |
Wilton A (2001) DNA methods of assessing dingo purity. In ‘A symposium on the dingo’. (Eds CR Dickman, D Lunney) pp. 49–56. (Royal Zoological Society of New South Wales)
Wood, WF, Terwilliger, MN, and Copeland, JP (2005). Volatile compounds from anal glands of the wolverine, Gulo gulo. Journal of Chemical Ecology 31, 2111–2117.
| Volatile compounds from anal glands of the wolverine, Gulo gulo.Crossref | GoogleScholarGoogle Scholar |
Yadav, S, Pickford, R, Zammit, RA, and Ballard, JWO (2021). Metabolomics shows the Australian dingo has a unique plasma profile. Scientific Reports 11, 5245.
| Metabolomics shows the Australian dingo has a unique plasma profile.Crossref | GoogleScholarGoogle Scholar |
Zhang, S-J, Wang, G-D, Ma, P, Zhang, L-L, Yin, T-T, Liu, Y-H, Otecko, NO, Wang, M, Ma, Y-P, Wang, L, Mao, B, Savolainen, P, and Zhang, Y-P (2020). Genomic regions under selection in the feralization of the dingoes. Nature Communications 11, 671.
| Genomic regions under selection in the feralization of the dingoes.Crossref | GoogleScholarGoogle Scholar |