Putting rakali in the spotlight: innovative methods for detecting an elusive semi-aquatic mammal
Emmalie Sanders


A
B
C
Abstract
Freshwater ecosystems rank among the most threatened environments on Earth. Monitoring aquatic and semi-aquatic species is vital to informing conservation of freshwater ecosystems. However, many semi-aquatic mammals can be difficult to detect with conventional survey methods.
We aimed to identify the most effective survey method for detecting an Australian semi-aquatic mammal, the rakali (Hydromys chrysogaster).
We compared rakali detection rates among camera-trapping, live-trapping and visual surveys, and tested the influence of camera angle, trap proximity to water and time of survey, across the Yanco Creek system in southern New South Wales.
Nocturnal spotlight surveys were the most effective method for detecting rakali, with most observations occurring while individuals were foraging or swimming in the water. Camera traps facing a floating platform and cage traps mounted on floating platforms performed better than those deployed on land. Downward-facing camera traps detected rakali three times more often than did forward-facing cameras. Trapping rakali was unreliable, with the species detected at fewer than half of the sites where presence was confirmed via visual observation and camera traps. For species absence to be determined with 95% confidence, 2–4 weeks of nightly trapping is required, compared with six nights of visual surveys or 12 nights for a platform-facing camera. Morning visual surveys were largely ineffective because of predominantly nocturnal rakali activity and difficulty in detecting signs in creek environments.
The likelihood of detecting rakali can be maximised through the use of nightly spotlighting and deployment of baited camera traps focussed on platforms or natural resting areas within a water body.
Understanding the effectiveness of each method is essential for developing species-appropriate protocols for population monitoring. Our findings present suitable options to be further explored among the 100-plus small (<1 kg) semi-aquatic mammals worldwide that share similar behaviours and characteristics to the rakali, many of which are threatened or data deficient.
Keywords: Australia, camera trap, conservation, detection, Hydromys, monitoring, semi-aquatic, water, water rat.
Introduction
Species detection is a key factor contributing to the accuracy of survey results and any subsequent research, monitoring, and conservation efforts (O’Connell et al. 2006; Garden et al. 2007; Peres et al. 2021). Inadequate survey methods can misinform management decisions (Suárez-Tangil and Rodríguez 2021). For instance, poorly suited survey methods may have a lower detection rate, and indicate that a population is smaller than it actually is or is completely absent (Thompson 2004; De Bondi et al. 2010). This is particularly problematic if threatened species remain undetected. As the effectiveness of different survey methods also varies among species, it is important to identify what methods are effective for individual species (Thomas et al. 2020).
Live-trapping is a common approach for monitoring small to medium-sized mammals, and presents the opportunity to collect data regarding age, sex and body condition (Torre et al. 2016; Harkins et al. 2019). However, trapping can be extremely labour intensive, and hence costly, especially when monitoring species with low capture rates (Thomas et al. 2020). Consequently, less intrusive methods, such as camera trapping, are increasingly being deployed to monitor small to medium-sized mammals across the world (Meek et al. 2014; Molyneux et al. 2017; Wearn and Glover-Kapfer 2019). The decision of which monitoring approach to deploy for an individual species relies on an accurate understanding of how any chosen device functions and their capacity to detect the species of interest (Welbourne et al. 2016; Moore et al. 2023).
Semi-aquatic mammals can be particularly difficult to monitor. Many semi-aquatic mammals are cryptic and spend the majority of their active time foraging in the water (Hood 2020). The aquatic medium presents challenges, with the success of passive infrared camera traps around water being highly varied. Some cameras are unable to detect species in or in close proximity to the water because of similar body-surface and water temperatures preventing the wet animal from triggering the sensor (Herrin 2010; Lerone et al. 2015; Findlay et al. 2020). However, camera traps have successfully detected semi-aquatic species when positioned at a distance from the water, at haul-out areas, and latrine sites (Day et al. 2016; Findlay et al. 2020; Gil-Sánchez and Antorán-Pilar 2020). Other specialised methods are often adopted to detect semi-aquatic mammals, such as pressure-plate activated camera traps, or cage traps adapted to be deployed in the water (Lerone et al. 2015; Baker et al. 2018). The use of environmental DNA sequencing has also been demonstrated as a powerful tool in detecting semi-aquatic mammals, particularly in comparison with alternate methods (Sales et al. 2020; Brunt et al. 2021). Similarly, detection dogs trained for specific species are able to effectively locate cryptic mammals even in areas where previous methods have failed (Shields and Austin 2018; Thomas et al. 2020).
The rakali (Hydromys chrysogaster) is a semi-aquatic rodent native to Australia. It is highly adapted to the aquatic environment with partially webbed hind feet and a strong tail to aid swimming, dense vibrissae that can sense prey moving in the water and water-repellent double-layered fur for insulation (Watts and Aslin 1981; Hanke et al. 2020). It is predominantly nocturnal and carnivorous, with a diet consisting mainly of fish, crustaceans, invertebrates, and molluscs (Harris 1978; Woollard et al. 1978). It forages in water but consumes prey on land or exposed logs and vegetation, often within 10 cm of water (Harris 1978). Food remains left at a feeding site (midden) are one sign of rakali presence (Triggs 2004). The rakali shares morphological and behavioural tendencies similar to those seen in numerous other small semi-aquatic mammals (Hood 2020; Sanders et al. 2024). Further research on the rakali therefore has the potential to offer greater insight into other semi-aquatic mammals.
Rakali is elusive and trap shy, making the species difficult to detect (Williams, 2019). Live-trapping results vary significantly depending on location and season (Harris 1978; Gardner and Serena 1995; Smart et al. 2011; Leigh and Breed 2020). Live-trapping has failed to detect rakali, or did so at a very low rate, even in areas where the species is known to be abundant (Williams 2019). Community-based visual surveys have been successful in monitoring rakali presence (Trocini et al. 2015; Williams and Serena 2018), particularly where other passive methods such as hair funnels have failed (Gourmas 2011). Rakali individuals have been captured in fyke nets deployed for platypus (Ornithorhynchus anatinus), but can chew through netting and become entangled (Williams and Serena 2018). The use of camera traps in detecting rakali remains largely untested, with large variation in the few instances they have been used (Gourmas 2011; McIlduff et al. 2014; Rendall et al. 2014).
The rakali has been seldom studied or included in ecosystem assessments (Williams 2019). It is unknown how potential threats to rakali, including climate change, water management, increasing aridity and drought, affect it (Lee 1995; Scott and Grant 1997). A greater understanding of the best techniques to detect and monitor rakali is needed to inform such research. Here, we aimed to fill that gap by comparing the capacity to detect rakali using a range of survey methods.
Materials and methods
Study area
The study was conducted throughout the Yanco Creek System in southern New South Wales, Australia. The system begins at the confluence of the Yanco Creek and Murrumbidgee River near Narrandera and follows the Yanco, Colombo, Forest and Billabong creeks until the Billabong Creek connects to the Edward River at Moulamein. Combined, the system has over 800 km of highly regulated creek frontage. The predominant vegetation in the system consists of river red gum (Eucalyptus camaldulensis) forest or black box (Eucalyptus largiflorens) woodland with an understorey being dominated by sedges (Carex sp.), wallaby grass (Austrodanthonia sp.) or Warrego grass (Paspalidium jubiflorum) (State Government of NSW and Department of Planning and Environment 2022). The Yanco Creek System supports a diverse range of freshwater birds and fish, but the presence of rakali has been largely unreported (Atlas of Living Australia 2023). Fourteen sites spread across the system were selected from a long-term study as part of the Murrumbidgee Monitoring Evaluation and Research program (Turner et al. 2022). Twelve sites were located along the various creeks and backwaters within the system and two sites were located along billabongs (Fig. 1). The distance between sites ranged from 2.5 km to 32.5 km.
Camera trapping
Camera traps were set at each site for a minimum of 30 days between March and July 2022. We used Swift solar-powered passive infrared (PIR) cameras (Swift Enduro, Outdoor Cameras Australia) set to record five images per triggering event to increase the likelihood of obtaining clear, identifiable images. Three cameras were set at each site. Two cameras were paired on the bank within 5 m of the water, mounted to trees or 180-cm-long star pickets and orientated to capture movement between the water and land. One camera was positioned facing downward at a height of approximately 150 cm (as per De Bondi et al. 2010), and the other facing forward at a height of approximately 50 cm. Paired cameras were mounted 2–3 m apart and arranged to observe a punctured tin of sardines secured beneath the downward-facing camera to act as a scent lure. The third camera was positioned overlooking a floating platform deployed in the water. The platform was created from a water-treated plywood base with a tube of PVC pipe lined with pool noodles mounted underneath for buoyancy (Fig. 2a). Ribbed marine carpet glued to the PVC pipe provided added grip for the rakali to climb onto the platform. Holes in the plywood allowed for three star pickets to secure the platform, while allowing it to move with fluctuating water levels. Another punctured tin of sardines was mounted on the middle star picket. The platform provided a uniform surface across sites to identify whether rakali is more readily detected when it is in or surrounded by water as opposed to on land. Images were processed and stored in the Wildlife Insights Database (Sanders 2021). Although this database uses machine-learning to identify the presence of an animal and identify its species, it was often unable to recognise the presence of rakali and other small mammals, and hence could not assign a correct taxonomic classification, perhaps owing to a lack of species-specific training data (Ahumada et al. 2020). Thus, all images were checked manually. Data were cleaned to represent single rakali events in which a rakali was detected with at least 15 min between the previous detection or a different individual was detected distinguished by body size and proportion of white on the tail tip.
Live-trapping
Live trapping was conducted from 30 April to 9 May and from 13 June to 5 July 2022, within a week of camera-trap collection. Six terrestrial wire-cage traps (20 × 20 × 57.5 cm, medium cage trap, Get Trapped) and 10 type-B Elliott traps (15.5 × 15 × 46 cm; Elliott Scientific Company, Upwey, Vic., Australia) were spaced evenly along a 500 m transect bordering the waterway at each site. Four additional cage traps were mounted to the platforms mentioned above and set in the water parallel to the transect (Fig. 2b). Platforms were set at a depth of 30–90 cm, depending on the total water depth of the wetland and safe wading depth. All traps were lined with coconut fibre and baited with whole pilchards, with a plastic covering over the rear half of each cage trap for added shelter. Trapping took place over three nights per site, with traps set 1 h before sunset and cleared at sunrise.
Captured rakali individuals were transferred to a handling bag modified after Koprowski (2002) for more efficient and safe processing of the animal. The bag was cone shaped with the largest end able to fit over the opening of the cage traps that was sealed with an adjustable buckle once the rakali was contained. A double-sided zip that ran the length of the bag enabled access to specific body parts that required observation or measuring (Fig. 3). Each rakali was tagged with a Trovan ID100B (1.4 mm) FDX-A Midichip, weighed, sexed, and body length, tail length, tail albinism length, head length and width and hind-foot length were measured. All procedures were approved by the Charles Sturt University Animal Care and Ethics Committee (A21212) and New South Wales Department of Planning, Industry and Environment (SL102560).
Visual surveys
During each trapping period, 30-min visual surveys were conducted each morning and night, for a total of six surveys per site. Night surveys were aided with hand-held spotlights. Any signs of rakali observed while walking the edge of the bank were recorded, including physical sightings of rakali, fresh footprints, scat or middens.
Statistical analysis
Rakali detections were organised into a binomial array of each site and night surveyed for each of the methods. The unmarked package ver. 1.2.5 (Fiske and Chandler 2011) and AICmodavg package ver. 2.3 (R package ver. 2.3.2; https://cran.r-project.org/package=AICcmodavg) were used to fit occupancy-detection models in R ver. 4.2.2 (R Core Team 2022). Additional packages used included magrittr ver. 2.0.3 (R package ver. 2.0.3; https://CRAN.R-project.org/package=magrittr) for basic formatting, and dplyr ver. 1.1.2 (R package ver. 1.1.2; https://CRAN.R-project.org/package=dplyr) for modifying variable orders for plots created with ggplot2 ver. 3.4.2 (Wickham 2016). A basic occupancy-detection model was fitted with survey method the only predictor to identify any differences between methods. The ‘predict’ function provided the nightly detection probability, standard error and 95% confidence interval of each method. The number of nights required at each site was calculated as
where P is the nightly detection probability and N is the minimum number of nights required to determine species absence at the specified level of confidence (α) (Kéry 2002; De Bondi et al. 2010). The number of nights required to be 80%, 90% and 95% confident of rakali detection was calculated. The cumulative probability of detection of combined methods was determined via the equation
where P is the overall cumulative probability of detection and ∏ denotes the product over all survey approaches. The total number of different survey approaches is given by n. The probability of not detecting the species in ni attempts for the ith survey approach is given by (1 − Pi)ni, where Pi is the probability of detection for the ith approach and ni is the number of attempts for the ith approach.
Results
Species detection
Rakali presence was confirmed at 13 of the 14 sites across a combination of all methods. Nightly visual surveys and platform-facing cameras both detected species at nine sites, while all other methods detected rakali only at six or fewer sites (Table 1). Additional species observed via the camera traps included the brushtail possum (Trichosurus vulpecula), European red fox (Vulpes vulpes), feral cat (Felis catus), livestock (domestic cattle and sheep) and numerous waterbirds (Supplementary Table S1).
Method | Trap nights | Rakali detections | Sites detected | |
---|---|---|---|---|
Downward-facing camera | 565 | 183 | 6 | |
Forward-facing camera | 492 | 57 | 6 | |
Platform-facing camera | 483 | 145 | 9 | |
Cage trap | 252 | 7 | 4 | |
Elliott trap | 420 | 5 | 2 | |
Platform-mounted cage trap | 168 | 12 | 4 | |
Night visual survey | 42 | 29 | 9 | |
Morning visual survey | 42 | 3 | 3 |
Sampling effort
In all, 42 cameras were deployed for 1727 days in total. However, owing to camera malfunctions, false triggers causing the memory to fill, or platforms rising outside of the field of view only 1540 days were active (Table 1). Combined trapping effort resulted in 24 captures of 18 individual rakali. There was a large male skew in the sample of rakali captured (n = 13) and numerous juveniles (n = 10). The average weight was 615 ± 195 g (300–1060 g) and average body length 237 ± 25 mm (203–283 mm).
Detection probability
Night-time visual surveys showed the highest nightly detection probability of 0.41 ± 0.08 (s.e.), followed by platform-facing camera traps (0.22 ± 0.02), platform-mounted cage traps (0.18 ± 0.06) and cage traps (0.15 ± 0.06) (Fig. 4). Elliott traps and downward-facing cameras had a probability of 0.10 (±0.05; ±0.01), and morning visual surveys had a probability of detection of 0.08 ± 0.04 and forward-facing cameras only 0.07 ± 0.01. Consequently, these methods also required the least number of nights to be confident in species detection, with nightly surveys requiring only six nights and platform-facing cameras 12 nights to achieve 95% confidence (Table 2, Fig. 5). Nightly visual surveys performed significantly better than did the other methods (b = 1.342, z = 2.438, P = 0.015), whereas forward-facing camera traps performed the worst (b = −0.954, z = −1.972, P = 0.049). There was no significant difference in rakali detection among the remaining survey methods.
Average nightly detection probability of each survey method targeting rakali, with grey bars indicating the standard error.
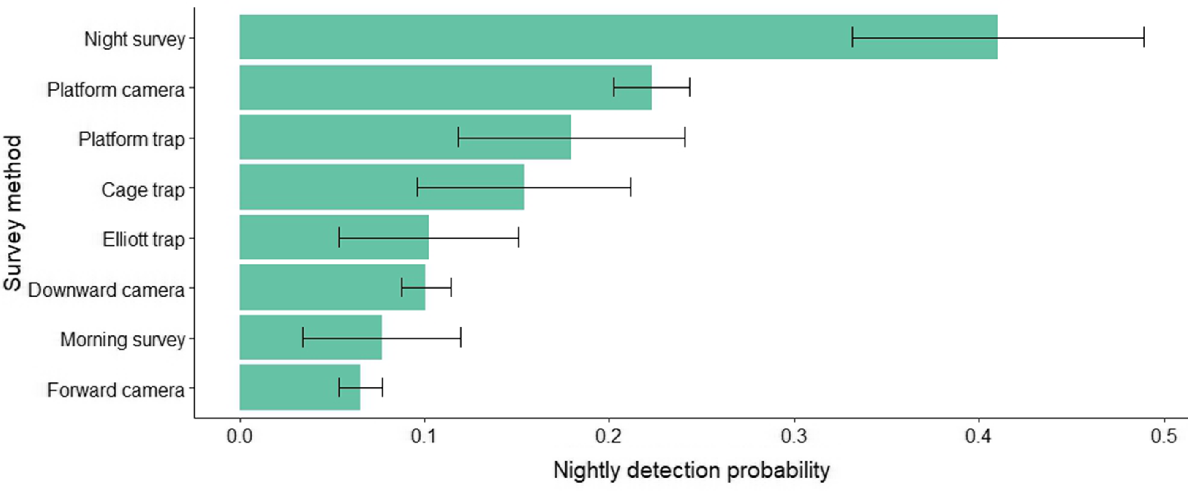
Survey method | Number of nights required | |||
---|---|---|---|---|
80% confidence | 90% confidence | 95% confidence | ||
Nightly searches | 4 (1.92–5.14) | 5 (2.74–7.36) | 6 (3.57–9.57) | |
Platform-facing camera | 7 (5.22–7.84) | 10 (7.46–11.21) | 12 (9.71–14.59) | |
Platform-mounted trap | 9 (3.99–17.46) | 12 (5.72–24.98) | 16 (7.44–32.50) | |
Cage trap | 10 (4.47–21.92) | 14 (6.39–31.36) | 18 (8.31–40.81) | |
Elliott trap | 15 (5.77–40.42) | 22 (8.26–57.83) | 28 (10.74–75.24) | |
Downward-facing camera | 16 (11.51–19.93) | 22 (16.47–28.51) | 29 (21.43–37.10) | |
Morning searches | 21 (6.72–63.52) | 29 (9.61–90.88) | 38 (12.51–118.24) | |
Forward-facing camera | 24 (16.56–34.28) | 35 (23.69–49.04) | 45 (30.82–63.81) |
The respective upper and lower 95% confidence intervals are given in parentheses.
Cumulative probability of detecting rakali over time (survey nights) for eight survey methods.
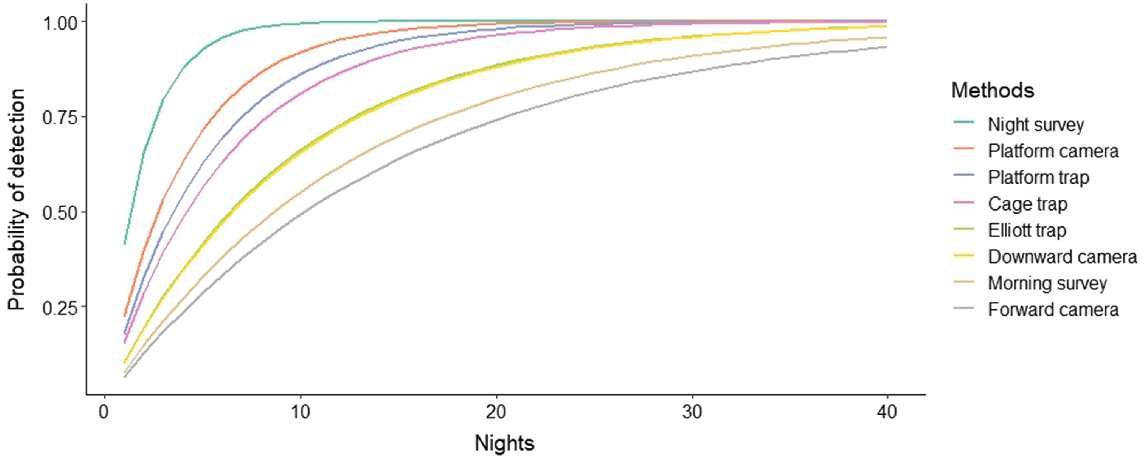
Combining methods and increasing the number of traps set further reduced the number of nights required to achieve certainty of rakali absence above 90% (Table 3). Various combinations of the top methods showed similar detection probabilities, with as little as two to nine nights required. The number of traps set was also increased to reduce the number of nights required, such that four nights with three times as many platform-mounted traps (12 traps) or 24 terrestrial cage traps still provides >90% certainty.
Survey method | Nights | cP | |
---|---|---|---|
Extended night survey (1 h) | 3 | 0.95 | |
Platform-facing camera + two downward-facing cameras | 7 | 0.96 | |
5 | 0.90 | ||
Platform-facing camera + downward-facing camera | 9 | 0.96 | |
7 | 0.92 | ||
Night survey + platform-facing camera | 4 | 0.96 | |
3 | 0.90 | ||
Night survey + three platform-facing cameras | 2 | 0.92 | |
Night survey + four platform-facing cameras | 2 | 0.95 | |
12 platform-mounted traps | 5 | 0.95 | |
4 | 0.91 | ||
Four platform-mounted traps + 12 cage traps | 5 | 0.93 | |
18 cage traps | 6 | 0.95 | |
24 cage traps | 4 | 0.93 | |
30 cage traps | 3 | 0.92 |
Discussion
Freshwater ecosystems are one of the most threatened globally, and their occupants, including semi-aquatic mammals, have an elevated risk of extinction (Dudgeon et al. 2006; Sanders et al. 2024). Monitoring of these at-risk species is paramount to ensure any population declines do not go unnoticed and species can be targeted for conservation interventions. Even though rakali is considered least concern nationally, it has a short lifespan and low fecundity and can easily become extinct locally after prolonged drought or habitat alteration (Lee 1995; Scott and Grant 1997; Sanders 2021). The rakali is already listed in Western Australia as a rare Priority species in need of monitoring due to such events (Lee 1995). Here, rakali were most readily detected when in the water, rather than on land. The top three methods were nocturnal surveys, where the vast majority of rakali individuals were observed foraging or swimming in the water, and on platforms deployed in the water for both camera and cage traps. For a semi-aquatic mammal, focusing on methods within their main foraging medium appears highly beneficial.
Traps mounted to floating platforms have been successful in trapping water voles (Arvicola amphibius), muskrats (Ondatra zibethicus and Neofiber alleni), South American water rats (Nectomys squamipes), water opossums (Chironectes minimus), mink (Neovision vison) and spot-legged turtles (Rhinoclemmys punctularia) (Schooley and Branch 2005; Ahlers et al. 2010; Harrington et al. 2012; Leite et al. 2016; Lima et al. 2016; Baker et al. 2018; Garcia-Passos et al. 2018). However, the use of cameras overlooking such platforms has not been previously reported and provides a successful novel method. Camera traps have been used at a distance from the water along runways or haul-out areas, particularly for larger semi-aquatic mammals such as otters (Lutra lutra) and coypu (Myocastor coypus) (Gil-Sánchez and Antorán-Pilar 2020; Mori et al. 2020). Camera traps are used even less frequently for smaller semi-aquatic mammals, although detections of the Japanese water shrew (Chimarrogale platycephala) (Yonezawa et al. 2020) and now the rakali suggest that this is a promising method for such species. Further, platforms provide a clear slate for dietary sample collection from scat and food remains, although for longer deployments, care must be taken to monitor water levels or deploy only in stable water bodies.
Despite some methods outperforming others, the best methods for estimating species presence are not always the most efficient regarding cost or time (Moore et al. 2023). Although spotlighting at night yielded the quickest confirmation of rakali presence, this method still required six nights to confidently determine whether the species was absent without extending survey times. This would be very labour intensive for larger surveys across multiple sites. The deployment of scent-baited cameras observing platforms in the water, or similar natural resting areas, presents a potential option for projects requiring assessment across multiple sites. With twice as many nights required for camera deployment, this still required only two field trips for the initial set-up and camera retrieval. However, any time saved in the field may be lost during the processing of camera images, particularly with numerous false triggers and non-target species detections (Findlay et al. 2020). Most false triggers were caused by vegetation growing into frame, shadows and movement in the water from floating debris or waves. All cameras were set with the highest sensitivity level to prevent any missed captures of rakali; however, the use of a lower-sensitivity level would be worth investigating to minimise excessive triggers and image-processing times. Although cost analysis was not completed during this study, total costs of monitoring should be considered from the initial purchase of cameras traps or live traps to the salaries of staff for fieldwork and image processing (De Bondi et al. 2010; Molyneux et al. 2017; Moore et al. 2023).
As previously reported in other studies (Smart et al. 2011; Williams 2019), rakali were hesitant to enter traps in our study area, with a 10% probability of trapping rakali on a single night for terrestrial traps, or 20% probability for platform-mounted traps. Overall, the trapping success rate for platform cage traps was 7%, terrestrial cage traps 3% and Elliott traps 1%. This is congruent with previous trapping studies of the species, with an average 6% trap rate across multiple studies in areas of known rakali occurrence (Williams 2019). Trapping was extremely unpredictable, with seven individuals captured at a single site despite no rakali observations during visual surveys, yet another site with six individuals observed on a single night resulted in no captures. An additional trapping session conducted over 3 weeks in February 2023 also failed to capture a single rakali despite providing multiple bait options and fresh footprints surrounding the traps. The capture success rate of rakali is seasonal and is greatest in autumn and winter (Harris 1978). However, even when surveying during this time, fewer than half of the sites resulted in any captures despite numerous rakali observations.
Terrestrial-based camera traps were among the worst-performing methods to detect rakali. Because these are more often used in broad-scale monitoring, at greater distances to waterbodies or using plant-based lures or no bait, this may explain the scarcity of rakali identified in broadscale studies that include waterways (Homan 2022; Seidlitz et al. 2022; Kutt et al. 2023). Camera traps used to detect semi-aquatic mammals deployed near the water have previously failed to detect their target species, particularly in close proximity to the water. Wet animals have a lower capture success than do the dry ones because a wet animal’s surface temperature can be similar to its surroundings (Lerone et al. 2015; Findlay et al. 2020). In the rakali, peripheral vasoconstriction and insulating fur reduce heat loss when swimming (Dawson and Fanning 1981), which would reduce the amount of infrared radiation emitted from an animal, thereby decreasing the ability of a camera to distinguish it from the water (Herrin 2010; Lerone et al. 2015; Findlay et al. 2020). However, in our study numerous rakali were photographed swimming past the platforms or from the terrestrial cameras when water levels rose within the field of view. Recent surveys where rakali was included as a target species, with camera traps set within 1 m of the water or under structures such as bridges or jetties, successfully detected rakali presence (McIlduff et al. 2014; Parrott et al. 2020).
Camera angle also determined whether an individual triggered the camera, particularly when they were on land. Generally, forward-facing cameras with a larger field of view outperform downward-facing cameras for small to medium-sized mammals (Taylor et al. 2014; Nichols et al. 2017; Moore et al. 2020). Conversely, downward-facing cameras allow for clearer identification of small mammals and have a greater probability of detection for dorsoventrally flattened species such as varanids (De Bondi et al. 2010; Moore et al. 2020). In this study, forward-facing cameras recorded only a third of the events observed by the paired downward-facing camera, meaning the camera failed to recognise rakali despite individuals being within the camera’s field of view. These false negatives could be attributed to two aspects of PIR camera functioning. Passive infrared cameras are triggered when a change in infrared radiation is detected between a background surface and an object, such as moving vegetation or the body of an animal (Welbourne et al. 2016). For an animal to be successfully captured, it must pass through the sensor’s detection zone to first trigger the camera and the viewable area of the camera, an overlap known as the registration area (Findlay et al. 2020; Moeller et al. 2023). The amount of infrared radiation that is detectable by the camera trap is reduced at longer distances, and more so when wet (Findlay et al. 2020). Whereas rakali undetected by the forward-facing camera likely failed to enter the detection zone and registration area, trigger accuracy is also reduced with an increased distance, smaller body size, and the closer the body surface temperature is to the surroundings (Welbourne et al. 2016; Randler and Kalb 2018; Findlay et al. 2020). It is likely that a combination of factors affected the triggering accuracy of a forward-facing camera. Given this, greater rakali detections by forward-facing cameras may be achieved if set closer to the lure and at a lower height (e.g. 30 cm), although this is not always possible when set near fluctuating water levels. It is worth noting that such fluctuations are also likely to have affected the alignment of the registration area of the platform-facing camera on the platform.
Although rakali can be active in the early morning, most of the activity occurs at night (Harris 1978; Gardner and Serena 1995). This was reflected in the scarcity of rakali activity observed during our morning surveys. Footprints were visible only in soft mud and clay substrates, which were scarcely present, with most banks being too dry or covered in leaf litter. Because rakali often consumes its prey on exposed objects in the water (Harris 1978), many middens and scat were not visible from land. Although daytime visual surveys are often used for other semi-aquatic mammals such as muskrats with distinctive nests or latrine sites, this is not a wholly effective method for rakali (Schooley and Branch 2005; Day et al. 2016; Sales et al. 2020). Daytime surveys are still effective when rakali signs such as tracks, scat and middens are being sought (e.g. for dietary study). However, because most signs were not found readily, such surveys should be completed once rakali presence has been confirmed, and when water levels are decreasing, to increase the likelihood of detecting tracks along the water’s edge.
Management implications
Our findings have broad implications for the design of monitoring programs for rakali and other semi-aquatic mammals. Future monitoring programs around wetlands and other water bodies should aim to incorporate effective methods for determining rakali presence. We recommend the inclusion of spotlighting across water bodies during night-time observation-based projects, or the deployment of an additional camera trap overlooking a haul-out area in the water with an appropriate scent lure during any monitoring surrounding waterways. Rakali is a key component of aquatic ecosystems, but many areas lack records of species presence, let alone a baseline indication of the species’ health within an area. With increasing pressures facing freshwater systems, coupled with climate change, more care must be taken to monitor all water-dependent species (Dudgeon et al. 2006). Although rakali is currently listed as least concern, the species is short-lived and has a low reproductive output, making it vulnerable to changes within its environment (Scott and Grant 1997). Greater awareness of the species through citizen-science and long-term monitoring programs of aquatic environments could provide indicators of other threats should local rakali populations disappear.
Data availability
Camera-trap data are available online via the Wildlife Insights database: http://n2t.net/ark:/63614/w12004053.
Declaration of funding
Funding was provided through the Australian Government Research and Training Program and Murrumbidgee Monitoring Evaluation and Research project.
Acknowledgements
We acknowledge Simon McDonald of the Charles Sturt University Spatial Data Analysis Network (SPAN) for the creation of Fig. 1. Special thanks are given to field volunteers Amy Stephens, Alexandra Toth, Zac Rolfe, William Lawson, Simon Van Every, Christopher Matheson and Richard Sanders.
References
Ahlers AA, Heske EJ, Schooley RL, Mitchell MA (2010) Home ranges and space use of muskrats Ondatra zibethicus in restricted linear habitats. Wildlife Biology 16(4), 400-408.
| Crossref | Google Scholar |
Ahumada JA, Fegraus E, Birch T, Flores N, Kays R, O’Brien TG, Palmer J, Schuttler S, Zhao JY, Jetz W, Kinnaird M, Kulkarni S, Lyet A, Thau D, Duong M, Oliver R, Dancer A (2020) Wildlife insights: a platform to maximize the potential of camera trap and other passive sensor wildlife data for the planet. Environmental Conservation 47, 1-6.
| Crossref | Google Scholar |
Atlas of Living Australia (2023) Hydromys chrysogaster Geoffroy, 1804. (Atlas of Living Australia: Canberra) Available at https://www.ala.org.au/ [Accessed 10 October 2023]
Baker R, Scott DM, Keeling C, Dwight C (2018) Overwinter survival and post-release movements of translocated water voles: implications for current mitigation guidance. European Journal of Wildlife Research 64, 56.
| Crossref | Google Scholar |
Brunt T, Cecil M, Griffiths J, Adams-Hosking C, Murray P (2021) Where are the platypuses (Ornithorhynchus anatinus) now? A snapshot in time of their distribution in the Greater Brisbane region. Australian Mammalogy 43, 368-372.
| Crossref | Google Scholar |
Dawson TJ, Fanning FD (1981) Thermal and energetic problems of semiaquatic mammals: a study of the Australian water rat, including comparisons with the platypus. Physiological Zoology 54, 285-296.
| Crossref | Google Scholar |
Day CC, Westover MD, Hall LK, Larsen RT, McMillan BR (2016) Comparing direct and indirect methods to estimate detection rates and site use of a cryptic semi-aquatic carnivore. Ecological Indicators 66, 230-234.
| Crossref | Google Scholar |
De Bondi N, White JG, Stevens M, Cooke R (2010) A comparison of the effectiveness of camera trapping and live trapping for sampling terrestrial small-mammal communities. Wildlife Research 37, 456-465.
| Crossref | Google Scholar |
Dudgeon D, Arthington AH, Gessner MO, Kawabata Z-I, Knowler DJ, Lévêque C, Naiman RJ, Prieur-Richard A-H, Soto D, Stiassny MLJ, Sullivan CA (2006) Freshwater biodiversity: importance, threats, status and conservation challenges. Biological Reviews of the Cambridge Philosophical Society 81, 163-182.
| Crossref | Google Scholar | PubMed |
Findlay MA, Briers RA, White PJC (2020) Component processes of detection probability in camera-trap studies: understanding the occurrence of false-negatives. Mammal Research 65, 167-180.
| Crossref | Google Scholar |
Fiske I, Chandler R (2011) unmarked: an r package for fitting hierarchical models of wildlife occurrence and abundance. Journal of Statistical Software 43, 1-23.
| Crossref | Google Scholar |
Garcia-Passos GO, Soeiro MS, Lira-da-Silva RM (2018) Use of tomahawk traps in freshwater Testudines capture. Herpetology Notes 11, 285-289.
| Google Scholar |
Garden JG, McAlpine CA, Possingham HP, Jones DN (2007) Using multiple survey methods to detect terrestrial reptiles and mammals: what are the most successful and cost-efficient combinations? Wildlife Research 34, 218-227.
| Crossref | Google Scholar |
Gardner JL, Serena M (1995) Observations on activity patterns, population and den characteristics of the water rat Hydromys chrysogaster along Badger Creek, Victoria. Australian Mammalogy 18, 71-75.
| Crossref | Google Scholar |
Gil-Sánchez JM, Antorán-Pilar E (2020) Camera-trapping for abundance estimation of otters in seasonal rivers: a field evaluation. European Journal of Wildlife Research 66, 72.
| Crossref | Google Scholar |
Hanke W, Meyer S, Bleckmann H, Dehnhardt G (2020) Hydrodynamic reception in the Australian water rat, Hydromys chrysogaster. Journal of Comparative Physiology A 206, 517-526.
| Crossref | Google Scholar |
Harkins KM, Keinath D, Ben-David M (2019) It’s a trap: optimizing detection of rare small mammals. PLoS ONE 14, e0213201.
| Crossref | Google Scholar | PubMed |
Harrington LA, Hays GC, Fasola L, Harrington AL, Righton D, Macdonald DW (2012) Dive performance in a small-bodied, semi-aquatic mammal in the wild. Journal of Mammalogy 93(1), 198-210.
| Crossref | Google Scholar |
Homan P (2022) Surveys of vertebrate fauna on the northern outskirts of metropolitan Melbourne, 2006-2022: (1) mammals. The Victorian Naturalist 139, 173-183.
| Google Scholar |
Koprowski JL (2002) Handling tree squirrels with a safe and efficient restraint. Wildlife Society Bulletin 30, 101-103.
| Google Scholar |
Kutt AS, Waller NL, Colman NJ, Perry JJ, Starr CR (2023) Camera trapping ekes out some improvement for surveying sparse mammal populations in northern Queensland. Australian Mammalogy 45, 293-304.
| Crossref | Google Scholar |
Kéry M (2002) Inferring the absence of a species: a case study of snakes. The Journal of Wildlife Management 66, 330-338.
| Crossref | Google Scholar |
Leigh CM, Breed WG (2020) A demographic study of the water-rat (Hydromys chrysogaster) on the River Torrens in Adelaide, South Australia. Australian Mammalogy 42, 277-282.
| Crossref | Google Scholar |
Leite MdS, Galliez M, Queiroz TL, Fernandez FAS (2016) Spatial ecology of the water opossum Chironectes minimus in Atlantic forest streams. Mammalian Biology 81(5), 480-487.
| Crossref | Google Scholar |
Lerone L, Carpaneto GM, Loy A (2015) Why camera traps fail to detect a semi-aquatic mammal: activation devices as possible cause. Wildlife Society Bulletin 39, 193-196.
| Crossref | Google Scholar |
Lima DO, Pinho GM, Fernandez FAS (2016) Spatial patterns of the semi-aquatic rodent Nectomys squamipes in Atlantic forest streams. Journal of Natural History 50(7–8), 497-511.
| Crossref | Google Scholar |
McIlduff C, Koeller K, Wilson B, Bleby K (2014) Use of remote cameras to survey and monitor mammal fauna occurrence at Loch McNess and Lake Yonderup, Yanchep National Park, Perth, Western Australia (2011–2013). Report for Department of Parks and Wildlife. Government of Western Australia Department of Parks and Wildlife. Perth, Western Australia. Available at https://library.dbca.wa.gov.au/#record/135247 [Accessed 15 November 2023]
Meek PD, Ballard G-A, Fleming PJS, Schaefer M, Williams W, Falzon G (2014) Camera traps can be heard and seen by animals. PLoS ONE 9, e110832.
| Crossref | Google Scholar | PubMed |
Moeller AK, Waller SJ, DeCesare NJ, Chitwood MC, Lukacs PM (2023) Best practices to account for capture probability and viewable area in camera-based abundance estimation. Remote Sensing in Ecology and Conservation 9, 152-164.
| Crossref | Google Scholar |
Molyneux J, Pavey CR, James AI, Carthew SM (2017) The efficacy of monitoring techniques for detecting small mammals and reptiles in arid environments. Wildlife Research 44, 534-545.
| Crossref | Google Scholar |
Moore HA, Valentine LE, Dunlop JA, Nimmo DG (2020) The effect of camera orientation on the detectability of wildlife: a case study from north-western Australia. Remote Sensing in Ecology and Conservation 6, 546-556.
| Crossref | Google Scholar |
Moore HA, Dunlop JA, Geyle HM, Greenwood L, Nimmo DG (2023) First you get the money, then you get the power: comparing the cost and power of monitoring programs to detect changes in occupancy of a threatened marsupial predator. Conservation Science and Practice 5, e12881.
| Crossref | Google Scholar |
Mori E, Andreoni A, Cecere F, Magi M, Lazzeri L (2020) Patterns of activity rhythms of invasive coypus Myocastor coypus inferred through camera-trapping. Mammalian Biology 100, 591-599.
| Crossref | Google Scholar | PubMed |
Nichols M, Glen AS, Garvey P, Ross J (2017) A comparison of horizontal versus vertical camera placement to detect feral cats and mustelids. New Zealand Journal of Ecology 41, 145-150.
| Crossref | Google Scholar |
O’Connell AF, Jr, Talancy NW, Bailey LL, Sauer JR, Cook R, Gilbert AT (2006) Estimating site occupancy and detection probability parameters for meso- and large mammals in a coastal ecosystem. The Journal of Wildlife Management 70, 1625-1633.
| Crossref | Google Scholar |
Parrott ML, Doody JS, McHenry C, Clulow S (2020) Eat your heart out: choice and handling of novel toxic prey by predatory water rats. Australian Mammalogy 42, 235-229.
| Crossref | Google Scholar |
Peres PHdF, Grotta-Neto F, Luduvério DJ, Oliveira MLd, Duarte JMB (2021) Implications of unreliable species identification methods for neotropical deer conservation planning. Perspectives in Ecology and Conservation 19, 435-442.
| Crossref | Google Scholar |
Randler C, Kalb N (2018) Distance and size matters: a comparison of six wildlife camera traps and their usefulness for wild birds. Ecology and Evolution 8, 7151-7163.
| Crossref | Google Scholar | PubMed |
Rendall AR, Sutherland DR, Cooke R, White J (2014) Camera trapping: a contemporary approach to monitoring invasive rodents in high conservation priority ecosystems. PLoS ONE 9, e86592.
| Crossref | Google Scholar | PubMed |
Sales NG, McKenzie MB, Drake J, Harper LR, Browett SS, Coscia I, Wangensteen OS, Baillie C, Bryce E, Dawson DA, Ochu E, Hänfling B, Lawson Handley L, Mariani S, Lambin X, Sutherland C, McDevitt AD (2020) Fishing for mammals: landscape-level monitoring of terrestrial and semi-aquatic communities using eDNA from riverine systems. Journal of Applied Ecology 57, 707-716.
| Crossref | Google Scholar |
Sanders E (2021) Effectiveness of camera traps in detecting a cryptic semi-aquatic mammal. Wildlife Insights. Available at http://n2t.net/ark:/63614/w12004053 [15 November 2023]
Sanders E, Wassens S, Michael DR, Nimmo DG, Turner JM (2024) Extinction risk of the world’s freshwater mammals. Conservation Biology 38, e14168.
| Crossref | Google Scholar | PubMed |
Schooley RL, Branch LC (2005) Survey techniques for determining occupancy of isolated wetlands by round-tailed muskrats. Southeastern Naturalist 4, 745-756.
| Crossref | Google Scholar |
Seidlitz A, Bryant KA, Armstrong NJ, Wayne AF (2022) Animal detections increase by using a wide-angle camera trap model but not by periodically repositioning camera traps within study sites. Pacific Conservation Biology 28, 25-35.
| Crossref | Google Scholar |
Smart C, Speldewinde P, Mills H (2011) Influence of habitat characteristics on the distribution of the water-rat (Hydromys chrysogaster) in the greater Perth region, Western Australia. Journal of the Royal Society of Western Australia 94, 533-539.
| Google Scholar |
State Government of NSW and Department of Planning and Environment (2022) NSW state vegetation type map. The Sharing and Enabling Environmental Data Portal. Available at https://datasets.seed.nsw.gov.au/dataset/95437fbd-2ef7-44df-8579-d7a64402d42d [Accessed 15 November 2023]
Suárez-Tangil BD, Rodríguez A (2021) Estimates of species richness and composition depend on detection method in assemblages of terrestrial mammals. Animals 11, 186.
| Crossref | Google Scholar | PubMed |
Taylor BD, Goldingay RL, Lindsay JM (2014) Horizontal or vertical? Camera trap orientations and recording modes for detecting potoroos, bandicoots and pademelons. Australian Mammalogy 36, 60-66.
| Crossref | Google Scholar |
Thomas ML, Baker L, Beattie JR, Baker AM (2020) Determining the efficacy of camera traps, live capture traps, and detection dogs for locating cryptic small mammal species. Ecology and Evolution 10, 1054-1068.
| Crossref | Google Scholar | PubMed |
Torre I, Freixas L, Arrizabalaga A, Díaz M (2016) The efficiency of two widely used commercial live-traps to develop monitoring protocols for small mammal biodiversity. Ecological Indicators 66, 481-487.
| Crossref | Google Scholar |
Wearn OR, Glover-Kapfer P (2019) Snap happy: camera traps are an effective sampling tool when compared with alternative methods. Royal Society Open Science 6, 181748.
| Crossref | Google Scholar | PubMed |
Welbourne DJ, Claridge AW, Paull DJ, Lambert A, Rowcliffe M, Disney M (2016) How do passive infrared triggered camera traps operate and why does it matter? Breaking down common misconceptions. Remote Sensing in Ecology and Conservation 2, 77-83.
| Crossref | Google Scholar |
Williams G, Serena M (2018) Distribution of the Australian water-rat Hydromys chrysogaster in Victoria: findings from community-based sightings and live-trapping surveys. The Victorian Naturalist 135, 71-83.
| Google Scholar |
Woollard P, Vestjens WJM, Maclean L (1978) The ecology of the eastern water rat Hydromys chrysogaster at Griffith, N.S.W.: food and feeding habits. Wildlife Research 5, 59-73.
| Crossref | Google Scholar |
Yonezawa S, Ushio M, Yamanaka H, Miya M, Takayanagi A, Isagi Y (2020) Environmental DNA metabarcoding reveals the presence of a small, quick-moving, nocturnal water shrew in a forest stream. Conservation Genetics 21, 1079-1084.
| Crossref | Google Scholar |