Plant morphological traits and leaf nutrient concentration are associated with flammability and phylogenetic relationships in sub-alpine vegetation, New Zealand
Shanta Budha-Magar

A
B
C
D
E
F
Abstract
Understanding relationships among leaf nutrient concentrations, morphological traits and plant flammability aids predictions of plant flammability. Few studies have simultaneously examined these relationships in a phylogenetic context.
Quantify relationships among plant flammability, leaf nutrient concentrations, morphological traits and phylogenetic pattern.
We measured shoot and whole plant flammability (for small-sized species), shoot and leaf morphological traits, and leaf nutrient concentrations for 29 vascular plant taxa. Shared and unique plant flammability variation explained by nutrient traits, leaf morphology and shoot traits was estimated, incorporating phylogenetic relationships among species via variance partitioning.
Flammability had a substantial phylogenetic proportion; 28% of variation in flammability was explained either independently by phylogenetic relatedness or as shared variation with morphology and shoot traits (49%), or nutrient concentrations (20%). Twig dry matter content and retained dead material were positively and moisture content and most nutrient traits were negatively correlated with plant flammability.
Variation in leaf nutrient concentrations and plant morphology showed a strong phylogenetic pattern, suggesting that features of plants that determine their flammability are strongly underpinned by evolution.
The substantial shared variation between leaf nutrient concentrations, morphological traits and phylogenetic relationships suggests that morphological traits will be more useful than nutrient traits when predicting flammability.
Keywords: calcium, dead material, fire, flammability, leaf nutrients, magnesium, moisture content, morphological traits, phosphorus, phylogenetic relationships, potassium, sulfur, variance partitioning.
Introduction
Plant flammability refers to the ability of a plant to ignite and propagate fire (Anderson 1970; Santacruz-García et al. 2019; Tumino et al. 2019). Flammability can be quantified using standardised experimental burning of plants or plant parts as four components: (i) ignitability, how quickly a plant ignites; (ii) sustainability, how long a plant burns for; (iii) combustibility, which describes the amount of heat released; and (iv) consumability, which is the amount of biomass burnt, e.g. the proportion of fuel consumed (Anderson 1970; Martin et al. 1994; Gill and Zylstra 2005; White and Zipperer 2010). Variation in these four flammability components among plant species has been shown to be related to variation in plant functional traits (Pérez-Harguindeguy et al. 2013; Grootemaat et al. 2015; Cardoso et al. 2018; Alam et al. 2020).
Many different plant functional traits are correlated with flammability. Traits such as leaf and shoot dry matter content, retention of dead materials and volatile organic compounds are positively correlated whereas traits such as specific leaf area, leaf thickness and moisture content are negatively correlated with flammability (Grootemaat et al. 2015; Pausas et al. 2016; Alam et al. 2020; Ormeño et al. 2020; Popović et al. 2021). Among these traits, one of the strongest trait–flammability relationships is with foliar moisture content, the amount of water within plant leaves. Higher foliar moisture content decreases the likelihood of fire ignition and spread whereas low moisture content increases susceptibility to fire (Murray et al. 2013). However, one area of trait–flammability relationships that still needs attention is the link between flammability and leaf nutrient concentrations.
Much of our understanding of flammability–nutrient relationships comes from studies where several compounds of nutrients have been used as fire retardants, either in the natural environment to control wildfires (Scarff et al. 2012; Schafer and Mack 2018; Chukwunwike and Okafor 2019) or in wood/textile industries (Yan et al. 2019; Gebke et al. 2020; Fang et al. 2021; Guo et al. 2021). Higher concentrations of certain nutrients in leaf tissue have been shown to reduce flammability through several mechanisms. For instance, phosphorus compounds will release phosphorus-based volatiles into the flame zone, inhibiting combustion (Braun et al. 2006). Similarly, compounds with high nitrogen content yield non-flammable gases when combusted, and high phosphorus and sulfur contents can result in high dehydration of the fuel, producing char that is stable and heat-isolating (Horacek and Grabner 1996; Liodakis et al. 2002). Variation in phosphorus concentration indirectly influences fire behaviour (Scarff and Westoby 2008; Scarff et al. 2012, 2021). For instance, a study conducted using 32 evergreen tree and shrub species from eastern Australia observed a negative correlation between burning time and leaf phosphorus and nitrogen concentrations (Grootemaat et al. 2015). Other evidence of leaf nutrient concentrations influencing plant flammability can be speculated from their compounds used for other various purposes, such as sulfur-based flame retardants with low phosphate content that are used in the commercial wood industry (Gebke et al. 2020). Magnesium and potassium phosphate composites are used as fire-resistant compounds to coat substrates to protect against fire, such as birch plywood (Yan et al. 2019; Gebke et al. 2020; Fang et al. 2021), and calcium hydrogen phosphate dihydrate is a potential fire retardant for bonding wood-based materials (Ozyhar et al. 2022). These studies demonstrate that there are correlations between plant flammability and compounds of elements that also naturally occur at various concentrations within leaves, although leaf nutrient concentrations are not the only traits likely to influence flammability.
Multiple leaf and canopy traits influence the flammability of live fuels (Murray et al. 2013; Schwilk 2015; Alam et al. 2020) and many of these traits are consistently correlated with leaf nutrient concentrations (e.g. specific leaf area (SLA) and leaf N) (Domínguez et al. 2012; Wang et al. 2022). Leaf and canopy traits that influence flammability include shoot biomass, bulk density (BD), moisture content (MC), retained dead material (Dm), SLA, leaf size (area, LA; length, LL; and thickness, LT), and leaf dry matter content (dry mass/saturated mass) (Grootemaat et al. 2015; Alam et al. 2020). Leaf dry matter content (LDMC), twig dry matter content (TDMC) and the amount of Dm are positively related to plant flammability, whereas other traits, including SLA and MC, are often negatively related to plant flammability (Mason et al. 2016; Alam et al. 2020; Popović et al. 2021). Although leaf nutrient concentrations have a robust correlation with leaf productivity (Funk et al. 2021; Wang et al. 2022), the relative significance of leaf nutrient versus morphological traits in influencing plant flammability has rarely been investigated.
Understanding relationships between plant flammability and functional traits in the context of plant evolution is vital for understanding the evolution of plants in response to fire. Species’ traits, particularly leaf nutrient concentrations (Díaz et al. 2016; Yang et al. 2017) can vary over time with environmental conditions. The evolutionary process, despite generating biological diversity, also constrains trait evolution, resulting in conserved traits through lineages (Delsuc et al. 2005; Baum 2008). Taxa with a recent common ancestor are more likely to share a similar trait composition than taxa with a distant common ancestor (Delsuc et al. 2005). Therefore, phylogenetic distances between taxa may be used as a tool to examine trait variation, such as plant flammability. Several recent studies examined evolutionary patterns of plant flammability and morphological traits across species and suggested that both flammability and morphological traits are associated and phylogenetically conserved (Zhao et al. 2016; Cui et al. 2020a, 2020b, 2022). A study examined evolutionary patterns of litter flammability within the gymnosperm phylogenetic relatedness in relation to both morphological traits and leaf chemistry traits (secondary compound – terpene content; Cornwell et al. 2015), although the relationships between leaf nutrient concentrations (phosphorus (P), potassium (K), magnesium (Mg), sulfur (S) and calcium (Ca)) and flammability were not tested.
Several studies have examined the role of leaf nutrients (mainly phosphorus and nitrogen) in determining plant flammability; almost all these studies have focussed on flammability at the leaf scale (Scarff and Westoby 2008; Scarff et al. 2012; Grootemaat et al. 2015; Mason et al. 2016; Dehane et al. 2017), or as part of litter fuel mixes (Scarff and Westoby 2006). Leaf flammability is a poor predictor of shoot flammability (Alam et al. 2020). Therefore, shoot flammability should better approximate whole plant flammability because burning the entire shoot maintains the influence of plant architecture (Alam et al. 2020). Despite the importance of shoot flammability, and its recognised links to some leaf chemical concentrations, such as tannins, lignin and terpenes (Grootemaat et al. 2015; Ormeño et al. 2020), only one study has examined the association between leaf nutrient concentrations and shoot flammability (Alam et al. 2020). They showed that leaf phosphorus was not correlated with shoot flammability for 43 common indigenous perennial New Zealand plants (Alam et al. 2020). So far, no studies have estimated phylogenetic relationships in leaf nutrients and plant morphological traits, and how these traits relate to variation in shoot or whole plant flammability. Furthermore, shoot flammability is likely to be better than leaf flammability at predicting whole plant flammability (Alam et al. 2020). Although there has been considerable research into the relationships between leaf nutrients and leaf flammability (Etlinger and Beall 2004; Pickett et al. 2009; Alam et al. 2020), there has been little research into the relationship between leaf nutrients and shoot flammability. In this study, we specifically asked: is interspecific variation in shoot/whole plant flammability related to variation in leaf nutrient concentrations, morphological traits and phylogenetic conservatism, and what portion of variation in flammability is shared or independent among these (nutrients, morphology and phylogenetic relatedness) variable sets? We estimated phylogenetic patterns in leaf nutrient concentrations (P, K, Mg, S and Ca) and morphological traits (BD, TDMC, MC, Dm, LA, LL, LT, LDMC and SLA) and quantified their independent and shared contribution to variation with phylogenetic relatedness, in shoot/whole plant flammability, using 29 taxa from subalpine New Zealand. Our selection of nutrients in this study are based on the predicted relationships between them and flammability primarily from the fire safety literature in environment (Scarff and Westoby 2006; Scarff et al. 2012; Grootemaat et al. 2015) and industrial contexts (Liodakis et al. 2002; Yan et al. 2019; Gebke et al. 2020; Ozyhar et al. 2022). We predicted that flammability would be negatively correlated with leaf nutrient concentrations.
Methods
Study site
Plant samples were collected within a 2.4 km distance of each other in the summer of 2021 from Arthur’s Pass National Park, which lies across the Southern Alps, South Island, New Zealand (920 m above sea level (asl); 42°54′19.2″S, 171°33′56.0″E). Arthur’s Pass is a markedly wet zone (Leathwick et al. 2002); climate data from the last 49 years showed that Arthur’s Pass has a mean annual maximum temperature of 17.13 ± 0.60°C, a mean annual minimum temperature of 1.7 ± 0.45°C and mean annual rainfall of 4081 mm (NIWA 2021). East of the pass, treeline forests are dominated by Fuscospora cliffortioides (Nothofagaceae, mountain beech) but west of the pass, treeline forests are diverse admixtures of evergreen broadleaved angiosperms and conifers from which F. cliffortioides is absent (Cockayne 1898; Cockayne and Calder 1932). Subalpine ecosystems of shrublands and tussock grasses (Chionochloa spp.; Poaceae) occur between the two treeline forest types (Wardle 1964; Burge et al. 2020). Subalpine and forest ecosystems developed without regular exposure to fires (McGlone 2001; Guild and Dudfield 2010). Instances of fires, if any, were infrequent or sporadic, limiting the evolution of specific traits and adaptations in response to fire within these communities (Perry et al. 2014).
Sample collection
We collected both leaves and shoots to determine flammability and morphological traits, but nutrient concentration analysis was performed for leaves only. For 26 of the 29 taxa used in this study (Supplementary Table S1), we collected six samples (i.e. one sample from each of six individuals) of each taxon for flammability and morphological trait measurements (Supplementary Tables S2 and S3). We collected two to four samples of ≥15 g fresh weight of leaf material that could be readily collected for each taxon of 23 of the 26 taxa for leaf nutrient concentration analysis where possible. Only two replicates were collected for Lycopodium scariosum (Lycopodiaceae) and three replicates from Dracophyllum traversi (Ericaceae) owing to limited availability of material. Each replicate was collected from a different individual. In addition, four leaf samples were collected from each of six individuals per taxon to measure different foliar and morphological traits. We collected six samples from each taxon to measure a range of shoot morphology traits and shoot/whole plant flammability (Padullés Cubino et al. 2018; Alam et al. 2020; Cui et al. 2020a). Each shoot sample was collected from a different individual for each taxon. For trees and shrubs, 70 cm long terminal branches were cut from healthy and reproductively mature plants with sun-exposed shoots where possible. For grasses and forbs, whole tillers were collected including roots, then the roots were cut to a minimum length to preserve the aboveground plant architecture. In cases where the grasses and forbs were longer than 70 cm, the lower 70 cm was sampled and material above that length was trimmed (Padullés Cubino et al. 2018). For ferns, fronds of up to 70 cm were collected. Additionally, from those same individual plants, shoot sub-samples approximately 10 cm long from apical end of any branches were collected to measure moisture and shoot dry matter content. For the single-branched plants, each sample was collected from different individual plants that were close to each other in their habitat. Leaf samples were stored in plastic zip-lock bags; shoot samples were stored in black polythene bags and stored in a chilled environment (6°C) for a maximum of 5 days before trait measurements were taken. Few taxa were flowering at the time of sampling; material tested was mostly vegetative material from mature individuals.
Plant flammability measurements
We measured the flammability of taxa by burning shoot and whole above-ground plant samples using a device designed by Jaureguiberry et al. (2011) and modified by Wyse et al. (2016). Prior to burning, we air-dried samples at room temperature for 24 h, as per Wyse et al. (2018). We laid each sample horizontally on a grill for 2 min to preheat them, then ignited them using a blowtorch for 10 s. Time to ignition (if it occurred) and total burning time were recorded in seconds. Time to ignition was converted to an ignition score by subtracting the time to ignition from 10 (Padullés Cubino et al. 2018; i.e. a time to ignition of 3 s was converted to an ignition score of seven). Our ignition method is based on that developed by Jaureguiberry et al. (2011) who examined the effect of shorter or longer time of exposure to an ignition source (blowtorch) before settling on 10 s as an appropriate compromise between logistic feasibility and ecological realism. Having adopted this approach of turning on the blowtorch for 10 s, we contemplated how best to score the time to ignition of samples that did not ignite after 10 s of exposure to the blowtorch. We decided (following Padullés Cubino et al. 2018) that it was best to rescale the time to ignition as an ignition score as this would allow samples that did not ignite to be assigned a score of zero. Consequently, our procedure does not allow our assessments of time to ignition greater than 10 s. We consider this to be appropriate given the heat energy provided by our ignition source (16.7 kW/h; Wyse et al. 2016) relative to the size of our samples. We have found that a time to ignition range of 0.5–10 s allows us to discriminate between the ignition of a wide range of species, while still allowing the tests to be conducted within a logistically feasible short time frame. Samples that did not ignite were given an ignition score of zero. The maximum temperature attained during burning was recorded in degrees Celsius (°C) using an infrared thermometer (Fluke 572, Fluke Corp., Everett, WA, USA), which was pointed at the flames for the duration of the flaming combustion after the blowtorch had been turned off. Finally, burnt biomass was visually estimated as the percentage of biomass consumed in the fire. Taxa that did not ignite were assigned 0% burnt biomass and the maximum temperature was recorded as the initial temperature of the grill (i.e. 150°C) (Padullés Cubino et al. 2018; Alam et al. 2020) (Supplementary Table S2).
Leaf nutrient trait measurements
To measure leaf nutrient concentrations, we oven-dried leaf samples of ∼15 g for 72 h at 65°C, following previous methods (Pérez-Harguindeguy et al. 2013; Habte et al. 2016). A leaf sample of ∼5 g dry weight was then ground, and leaf nutrient concentrations (Ca, Mg, P, K and S) were analysed using an inductively coupled plasma – optical emission spectroscopy (Agilent 5110 ICP-OES) technique in a microwave digester (CEM MARS Xpress, CEM Corporation, NC, USA) at Lincoln University, New Zealand. A dried, ground and well-mixed 0.2 g dry weight sample was placed in a microwave vessel for element extraction. We added 2.0 mL trace element grade nitric acid (69%) and 2.0 mL of 30% hydrogen peroxide to the sample (modified EPA method 3050B; USEPA 1996), sealed the vessel and vortexed it. We digested plant samples following a heating program consisting of a heating ramp up to 90°C for 15 min and a heating plateau for 5 min (Liberato et al. 2017). This was followed by a heating ramp up to 180°C for 10 min and a heating plateau for 15 min. Finally, we recorded the amount of digested sample (mg kg−1) obtained after plant digestion.
Morphological trait measurements
To determine morphological trait variation among taxa, we measured a range of morphological traits: TDMC (g g−1), BD (g cm−3), LL (cm), LT (mm), LA (cm2), LDMC (g g−1) and SLA (cm2 g−1), and two other, non-morphological traits: MC (%) and Dm (%), following previous methods (Pérez-Harguindeguy et al. 2013; Alam et al. 2020). At the leaf level, we measured leaf dimensions, length and width using a ruler and thickness using a micrometer (model size 3202-25A of measuring range 0–25 mm). Then, we scanned leaf samples to create digital images from which we computed the LA using ImageJ (version 1.53E). In the case of compound leaves, individual leaf laminas or leaflets without petiole or rachis were measured. At the shoot level, we measured length, width and height of the sample that was collected for flammability measurement prior to burning. These measured shoot sample dimensions (length, width and height) were used to determine ellipsoidal volume (cm3) following Thorne et al. (2002) and then bulk density. We visually estimated the percentage retention of dead material (including leaves, branches and bark) present on each sample to determine the influence of dead material on flammability. For both leaves and shoots, we weighed the samples to obtain a fresh mass at the time of sampling and a wet mass (submerged in water for 7 h). We then oven-dried samples for 48 h at 65°C and reweighed them to obtain a dry mass. Then, to obtain LDMC (dry mass/wet mass) and SLA, we weighed the same sample that was used for the leaf dimension measurements at the leaf level. At the shoot level, we measured the weight of subsamples approximately 10 cm in length to obtain moisture and twig dry matter content.
DNA sequence data
To determine phylogenetic relationships in leaf nutrient traits, leaf morphology and plant flammability, we searched for published rbcl DNA sequences of the 29 taxa from GenBank (https://www.ncbi.nlm.nih.gov). For 15 taxa, DNA sequences were not available in GenBank; taxa within the same genus found in New Zealand were downloaded. Furthermore, our sequencing data are constrained by using only the rbcl marker because no other markers were available for all our species. Finally, we aligned DNA sequences online in Muscle (https://www.ebi.ac.uk/Tools/msa/muscle/) (Edgar 2004).
Data analysis
To supplement the field data, we obtained data from the literature and our own unpublished sources on flammability and morphological trait values for three taxa: Agrostis sp., Pimelea oreophila and Rytidosperma sp. (Curran TJ et al. unpubl. data; Padullés Cubino et al. 2018) and mean leaf nutrient concentration values for six taxa: Agrostis sp., Coprosma foetidissima, Myrsine nummularia, Pimelea oreophila, Poa colensoi and Rytidosperma sp. (Richardson SJ et al. unpubl. data). Similar protocols to the present study were used in these studies. Thus, in total, we had a complete dataset for 29 taxa (Supplementary Table S1). We estimated taxon-level values for flammability, leaf nutrient concentration, leaf morphology and shoot traits by taking the mean of individual samples within taxa; these were used in all analyses (Table 1 and Supplementary Fig. S1, Supplementary Tables S2 and S3).
Taxon name | Family | Calcium (Ca) (mg kg−1) | Potassium (K) (mg kg−1) | Magnesium (Mg) (mg kg−1) | Phosphorus (P) (mg kg−1) | Sulfur (S) (mg kg−1) | |
---|---|---|---|---|---|---|---|
Anisotome haastii | Apiaceae | 10,015.5 ± 513.39 | 24,062 ± 2343.76 | 2624 ± 164.81 | 1280.5 ± 49.42 | 1771.5 ± 49.61 | |
Astelia nervosa | Asteliaceae | 10,338.3 ± 741.55 | 14,622.5 ± 1079.77 | 1487.8 ± 114.24 | 477 ± 29.42 | 613 ± 41.64 | |
Blechnum minus | Blechnaceae | 5791 ± 617.66 | 21,867.5 ± 719.81 | 3770 ± 311.85 | 1107.5 ± 92.7 | 1130.3 ± 55.91 | |
Brachyglottis elaeagnifolia | Asteraceae | 10,010.5 ± 4078.26 | 22,506.5 ± 3358.61 | 2746.8 ± 492.18 | 1280.3 ± 177.47 | 2088.5 ± 437.43 | |
Celmisia armstrongii | Asteraceae | 3894.5 ± 768.85 | 9574.8 ± 1355.73 | 1066.5 ± 192.39 | 581.8 ± 38.16 | 640.8 ± 26.72 | |
Celmisia discolor | Asteraceae | 5713.3 ± 197.55 | 18,661.5 ± 976.81 | 1545.3 ± 102.46 | 1051.8 ± 70.07 | 1004.8 ± 73.94 | |
Chionochloa conspicua | Poaceae | 1370.3 ± 410.54 | 15,549 ± 1227 | 756.8 ± 77.93 | 657.5 ± 23.95 | 1112 ± 140.51 | |
Chionochloa rubra | Poaceae | 1107.5 ± 22.95 | 9805 ± 1158.92 | 675 ± 77.15 | 482.8 ± 43.66 | 1172.8 ± 42.01 | |
Coprosma serrulata | Rubiaceae | 15,660.8 ± 1108.91 | 15,597 ± 565.3 | 2619.3 ± 462.22 | 820.3 ± 38.07 | 1328.8 ± 156.44 | |
Dracophyllum longifolium | Ericaceae | 7283 ± 1104.79 | 4994.5 ± 130.43 | 949.5 ± 78.31 | 564 ± 13.26 | 713.8 ± 51.48 | |
Dracophyllum traversii | Ericaceae | 6179.3 ± 742.11 | 5983 ± 354.34 | 904.7 ± 155.03 | 555.7 ± 51.4 | 440.3 ± 36.16 | |
Dracophyllum uniflorum | Ericaceae | 8594.5 ± 818.26 | 5208.3 ± 472.21 | 1302.5 ± 120.22 | 551.5 ± 37.88 | 893.8 ± 38.75 | |
Fuscospora cliffortioides | Nothofagaceae | 8911.7 ± 1467.08 | 5367 ± 361.85 | 1249.3 ± 86.33 | 1065 ± 50.01 | 572.3 ± 13.32 | |
Gaultheria rupestris | Ericaceae | 11,385.3 ± 747.65 | 9168.5 ± 1230.94 | 2717.5 ± 202.32 | 692.8 ± 175.47 | 1014.5 ± 140.81 | |
Lycopodium scariosum | Lycopodiaceae | 933 ± 55 | 6942 ± 168 | 1785 ± 47 | 743 ± 13 | 742.5 ± 41.5 | |
Myrsine nummularia | Primulaceae | 10,800 | 6300 | 1800 | 1017.8 | 1800 | |
Pseudopanax colensoi | Araliaceae | 6936.5 ± 1641.55 | 13,669.5 ± 2068.75 | 2391.5 ± 109.62 | 906.3 ± 36.04 | 946 ± 20.81 | |
Ourisia macrophylla | Plantaginaceae | 7828.8 ± 302.84 | 17,463.3 ± 450.32 | 2596.5 ± 52.18 | 879.3 ± 30.91 | 1630.3 ± 122.6 | |
Phormium cookianum | Asphodelaceae | 3758 ± 630.77 | 10,532.3 ± 1125.27 | 843.8 ± 82.8 | 910 ± 100.67 | 558.3 ± 56.87 | |
Phyllocladus alpinus | Podocarpaceae | 6447.3 ± 1332.64 | 6804.3 ± 515.26 | 1274.5 ± 213.25 | 959.8 ± 113.25 | 730 ± 19.63 | |
Podocarpus nivalis | Podocarpaceae | 21,222.5 ± 3959.33 | 4499 ± 468.32 | 727.8 ± 112.33 | 687.5 ± 101.22 | 741.8 ± 47.41 | |
Polystichum vestitum | Dryopteridaceae | 4182.5 ± 817.71 | 15,904 ± 1139.94 | 2472.3 ± 318.46 | 1657 ± 208.53 | 2639.3 ± 201.72 | |
Veronica subalpina | Plantaginaceae | 7737.3 ± 942.63 | 13,031.3 ± 2581.92 | 1595.7 ± 295.97 | 1078.7 ± 18.84 | 1577.3 ± 294.27 |
A minimum of two samples for leaf nutrient traits for each taxon was used. Taxa for which we used pre-existing data (six taxa; Richardson SJ et al., unpubl. data) are not included in this table.
To determine the functional trait variations in flammability, morphology and leaf nutrient concentrations across the species, we performed separate principal component analyses (PCAs) for each of these three sets of variables. PCAs were implemented using the ‘pca’ function in the ‘FactoMineR’ package version 2.3 (Lê et al. 2008) on centred and standardised data. Further, to quantify individual trait–flammability relationships, we computed pairwise Pearson’s correlation coefficients between plant flammability, as measured by the species scores on the first flammability principal component and other traits (leaf nutrient concentrations, leaf morphology, shoot traits and the trait principal components).
We then employed variance partitioning to estimate the amount of variation in flammability (ignition score, maximum temperature, burning time and burnt biomass) explained by phylogenetic relatedness, leaf nutrient concentrations and morphological traits. This approach is based on its wide application in community ecology for understanding community assemblage in relation to different environmental variables (for example, Borcard et al. 2018), but it also has been applied in a phylogenetic context (Buckley et al. 2014). Our datasets included a leaf nutrient concentration dataset, a leaf and shoot morphology dataset and a phylogenetic distance set as explanatory variables with the flammability dataset as the response variable. First, we obtained matrices of plant flammability components, leaf nutrient concentrations, leaf morphology and shoot traits by standardising taxon level values of each of these traits using a scale function to ensure that trait values were on a comparable scale in ‘vegan’ version 2.6 (Oksanen et al. 2022). Second, we calculated the Euclidean distances among species on the standardised flammability matrix. Third, we used the phylogenetic distance matrix from DNA sequence data where phylogenetic distance was estimated using the maximum likelihood method implemented with the functions ‘dist.ml’ that estimates phylogenetics in ‘phangorn’ version 2.11 (Schliep et al. 2017) for all 29 taxa (Supplementary Fig. S2 and Supplementary Table S4). We then used these phylogenetic distances to generate a data frame of eigenvectors using the ‘pcnm’ function in ‘vegan’ by computing principal coordinates of neighbourhood map distances (pcnm) where each element represents the spatial distance between pair of taxa based on their phylogenetic relationships (Buckley et al. 2014). Forward selection within a distance-based redundancy analysis (db-RDA), based on Akaike’s Information Criterion (AIC) was used to identify an uncorrelated set of pcnm that were then included in the variance partitioning to represent phylogenetic variation among species; the top seven uncorrelated pcnms were selected (Borcard et al. 2018). We then performed variance partitioning to estimate the unique and shared variance in flammability explained by the three datasets: leaf nutrient concentrations, leaf and shoot morphology, and phylogenetic relatedness, following the procedure outlined in Borcard et al. (2018).
Data visualisations using the packages ‘ggplot2’ (Wickham 2016) and ‘ggreppel’ (Slowikowski et al. 2021) and all analyses (packages specified) were conducted in R version 4.3.0 (R Core Team 2023).
Results
Leaf nutrient concentrations, morphological traits and plant flammability varied widely among plant taxa (Table 1 and Supplementary Tables S2 and S3). When the four flammability variables (ignition score, maximum temperature, burning time and burnt biomass) were combined in a PCA, the first and second principal components (hereafter, flammability PC1 and PC2) explained 66.15% and 20.97% of the variation, respectively (Fig. 1 and Supplementary Table S5). The flammability PC1 represented higher ignition scores, burnt biomass and maximum temperature, and flammability PC2 represented higher burning time. Based on the first principal component PC1, Phyllocladus alpinus (Podocarpaceae), Chionochloa rubra (Poaceae), Pimelea oreophila (Thymelaeaceae), Celmisia discolor (Asteraceae), Lycopodium scariosum (Lycopodiaceae) and Phormium cookianum (Asphodelaceae) were the most flammable taxa (Fig. 1 and Supplementary Table S2) whereas Anisotome haastii (Apiaceae), Coprosma serrulata (Rubiaceae), Coprosma foetidissima (Rubiaceae), Brachyglottis elaeagnifolia (Asteraceae) and Ourisia macrophylla (Plantaginaceae) were the least flammable taxa.
Principal component analysis of relative taxon flammability for the 29 taxa from subalpine vegetation of South Island, New Zealand. Four flammability components (red arrows and text) are: ignition score (inverse ranking of time to ignition), maximum temperature, burning time and burnt biomass. Taxon codes are the first three letters of each of genus and species epithet (see full species list in Supplementary Table S1).
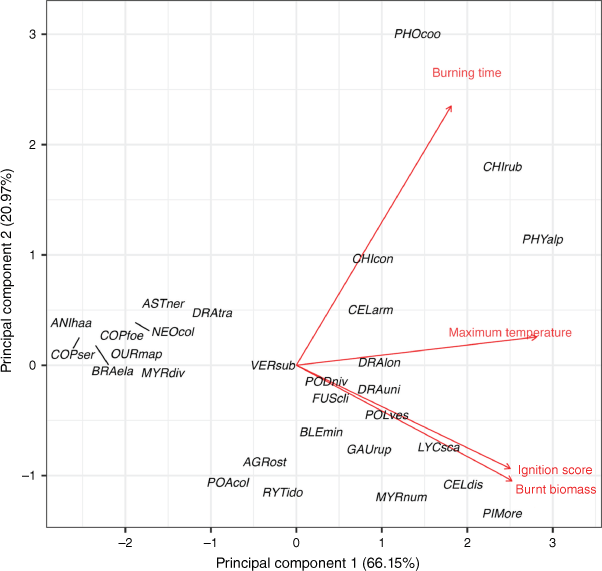
In the PCA of five leaf nutrient concentrations, PC1 and PC2 explained 52.56% and 26.45% of the total variation, respectively (Fig. 2). PC1 represented higher concentrations of magnesium, potassium, phosphorus and sulfur, and PC2 represented higher calcium concentration. When the range of leaf and shoot morphological traits were combined, the first component of the PCA on leaf morphology and shoot traits explained 31.54% of the total variation and represented TDMC, MC, LT, LL, LA, dead material and BD (Fig. 3; Supplementary Fig. S3). The second component explained 22.91% of the total variation, representing leaf morphology (LDMC), shoot traits (TDMC) and Dm. The third component explained 14.88% of the total variation and represented SLA and MC.
Principal component analysis of the mean scores for each taxon based on measurement of leaf nutrient concentrations (red) for 29 taxa from subalpine vegetation of South Island, New Zealand. Taxon codes are the first three letters of each of genus and species epithet as code (see full species list Supplementary Table S1). Nutrient concentration abbreviations are calcium (Ca), magnesium (Mg), phosphorus (P), potassium (K), and sulfur (S).
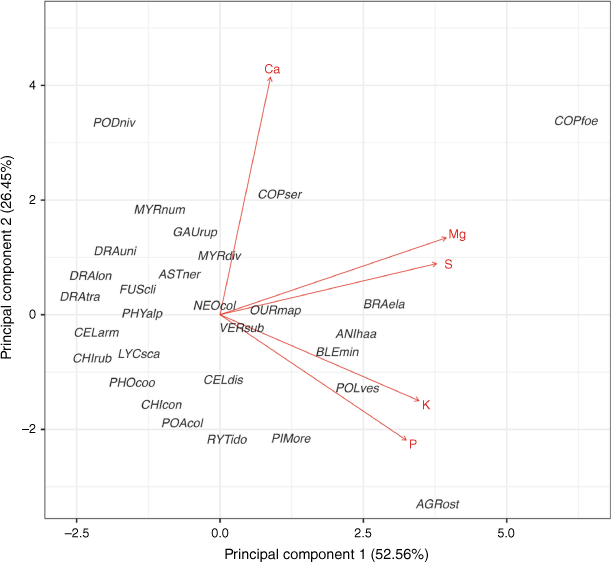
Principal component analysis of the mean scores for each taxon based on leaf (purple) and shoot (green) morphological trait measurements for all 29 taxa from subalpine vegetation of South Island, New Zealand. Taxon codes are the first three letters of each of genus and species epithet as code (see full species list, Supplementary Table S1). Trait abbreviations are leaf length, LL; leaf thickness, LT; leaf area, LA; leaf dry matter content, LDMC; specific leaf area, SLA; bulk density, BD; % dead material, Dm; % moisture content, MC; and twig dry matter content, TDMC.
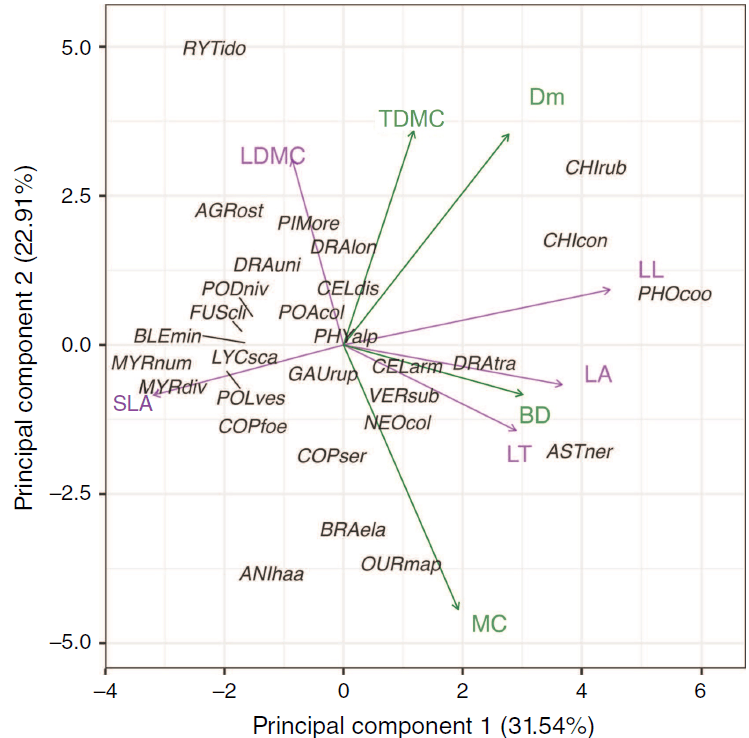
Pearson correlation coefficients showed that plant flammability was negatively correlated with leaf nutrient concentrations (except phosphorus) and positively correlated with most morphological traits of the leaves and shoots (Fig. 4; Supplementary Fig. S4). Moisture content was strongly negatively correlated with ignition score, maximum temperature reached, burnt biomass and flammability (PC1).
Pearson’s correlation coefficients between plant flammability variables and leaf nutrient concentrations, leaf morphology and shoot traits for all 29 plant taxa from subalpine vegetation of South Island, New Zealand, in addition to their correlations with principal components from the flammability PCA. Abbreviated variables are: concentration of calcium Ca; magnesium, Mg; phosphorus, P; potassium, K; sulfur, S; leaf length, LL; leaf thickness, LT; leaf area, LA; leaf dry matter content, LDMC; specific leaf area, SLA; bulk density, BD; % dead material, Dm; % moisture content, MC; twig dry matter content, TDMC; flammability PC1, PC1_flam; and flammability PC2, PC2_flam. The colour ramp represents positive (blue) to negative (red) correlations. Critical value of correlation (r) for 29 taxa is 0.34.
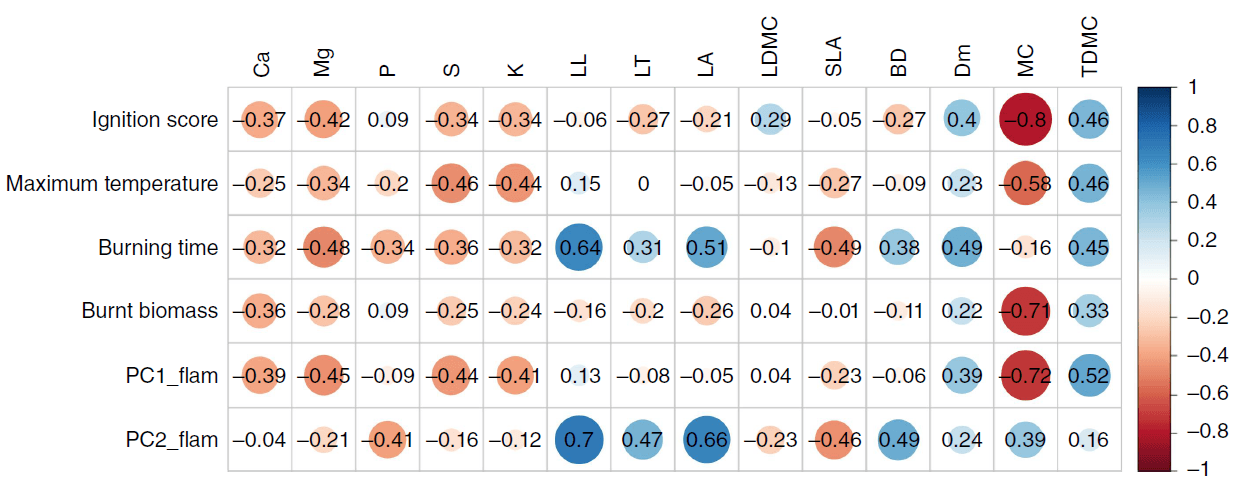
Variance partitioning showed that more than 50% of variation across taxa in shoot/whole plant flammability was explained by morphological traits, phylogenetic distances and leaf nutrient concentrations (Fig. 5). The largest portion of plant flammability was explained by leaf morphology and shoot traits (49.6%); however, all but 20% of this variation was shared with phylogenetic relatedness (12.7%), leaf nutrient concentration (15.5%), or both (7.3%). There was no unique plant flammability variation explained by leaf nutrient concentrations alone; rather, 3.8% of the variation was shared with phylogenetic relatedness and 7.3% was shared with both phylogenetic relatedness and morphology. Of the total variation, 47.7% was unexplained by three sets of plant trait variables.
Venn diagram showing the variation in plant flammability (ignition score, maximum temperature, burning time and burnt biomass) partitioned by three explanatory variable sets: Euclidean distances in leaf nutrient concentrations and leaf and shoot morphological traits, and phylogenetic distance PCNM scores using 29 taxa from subalpine vegetation of South Island, New Zealand. Leaf nutrient concentrations were of phosphorus (P), potassium (K), magnesium (Mg), calcium (Ca), and sulfur (S). Leaf and shoot morphological traits were leaf length (LL), leaf thickness (LT), leaf area (LA), leaf dry matter content (LDMC), specific leaf area (SLA), bulk density (BD), % dead material (Dm), % moisture content (MC) and twig dry matter content (TDMC). Variation in phylogenetic distances among species was quantified as seven Principal Coordinates of Neighbourhood Matrix (PCNM) scores. The negative variation value for the leaf nutrient concentrations was due to complete shared variation caused by the high intercorrelations among leaf nutrient concentrations and morphological traits and should be interpreted to be zero.
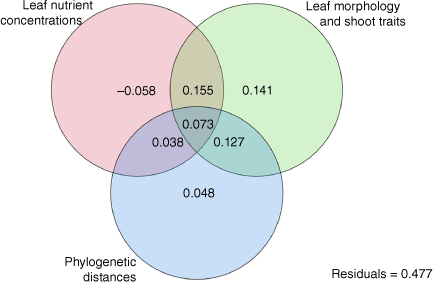
Discussion
Our study is one of the few to examine correlations between leaf nutrient traits and plant flammability (Scarff et al. 2012; Cornwell et al. 2015; Grootemaat et al. 2015; Mason et al. 2016; Alam et al. 2020) and, therefore, contributes more broadly to ecological research on the variation and evolution of plant flammability. We observed a substantial phylogenetic pattern in leaf nutrient concentrations, leaf morphology and shoot traits, and shoot/whole plant flammability. This is evidenced by the significant proportion of trait variation shared by both morphological and foliar nutrient traits and phylogeny when considering the variation of shoot/whole plant flammability. This result indicates that closely related plant taxa tend to have similar flammability characteristics inherited from their common ancestors, as has been demonstrated elsewhere (Murray et al. 2013; Cui et al. 2020a, 2020b). Thus, causal relationships between traits and flammability should be examined within a phylogenetic context, and simple bivariate relationships, e.g. between leaf nutrient concentrations and flammability, need to be treated with caution owing to a persistent phylogenetic contribution and collinearity of traits. This further demonstrates that it is the suites of traits, rather than single traits that influence flammability (Alam et al. 2020), reinforcing the need to consider morphological, physiological, architectural and nutrient concentration traits when examining drivers of flammability.
Our comparisons among three variable sets (leaf nutrients, leaf and shoot morphology, and phylogenetic relatedness) indicate that leaf and shoot morphological traits explained higher variance in plant flammability than leaf nutrients. There was also a substantial phylogenetic pattern in the flammability of the studied plant taxa. However, there was a lack of unique variation in shoot flammability explained by leaf nutrients. This result might be from including only five leaf chemistry traits whereas other studies have incorporated measurements of terpenes, which themselves are phylogenetically conserved (Zhang et al. 2021), and other flammable compounds occurring in leaves (Cornwell et al. 2015; Ormeño et al. 2020; Ganteaume et al. 2021; Guerrero et al. 2024). Flammable secondary compounds (e.g. terpenes) are carbon-rich compounds that increase flammability by having low flash points (Pausas et al. 2016; Ganteaume et al. 2021). However, nutrient-rich compounds exposed to high temperatures tend to form charcoal (and not release flammable gases) and are therefore not very flammable (Scarff and Westoby 2008). Furthermore, the weak effect of leaf nutrients traits on flammability when phylogenetic relatedness is considered is similar to previous research evaluating patterns between plant flammability in relation to leaf chemistry (terpenes) and a range of other traits across species; leaf nutrient variables were observed to have second-order effects on flammability but did play a role in determining burning time (Cornwell et al. 2015; Zhao et al. 2016). Additionally, the phylogenetic pattern of our result could be influenced by the inclusion of sequencing data using only the rbcl marker (Bell et al. 2017; Pere et al. 2023). Therefore, we suggest that future studies should include a wider range of leaf chemistry (those tested here, and nitrogen and carbon) and secondary compounds (e.g. terpenes), alongside morphological and shoot traits such as plant architecture, all examined within a phylogenetic context (i.e. taking phylogeny into account). Additionally, we acknowledge the potential importance of nitrogen in shoot and whole plant flammability as suggested by its influence on the flammability of materials (e.g. Horacek and Grabner 1996). We did not measure nitrogen in our study owing to cost constraints. It is possible that nitrogen could contribute to some of the unexplained variation in shoot and whole plant flammability in our species, although it is usually tightly correlated with P (Reich and Oleksyn 2004).
Individual correlations between flammability and leaf nutrient concentrations and leaf and shoot morphological variables were consistent with previous research (Grootemaat et al. 2015; Wyse et al. 2018; Alam et al. 2020). Our result of a negative correlation between plant flammability and the leaf concentrations of magnesium, potassium, calcium and sulfur in this plant community implies that leaf nutrient traits may help to inhibit ignition (Scarff et al. 2012; Grootemaat et al. 2015). High concentrations of these nutrients could result in cooler fires, with shorter burning time and less biomass consumption (Grootemaat et al. 2015), though our results suggest that other traits (moisture content, twig dry matter content and percentage of dead material retained) have greater influence on flammability. Our finding of a lack of correlation between phosphorus and shoot/whole plant flammability is consistent with a previous study examining the direct relationship between the two, i.e. phosphorus content and shoot/whole plant flammability (Alam et al. 2020), but contrasted with studies of leaf-level flammability (Grootemaat et al. 2015).
An interesting outcome of our study was finding species that have high flammability despite evolving in a landscape that has been quite fire-free over evolutionary time. New Zealand ecosystems generally had very low fire frequency prior to human arrival, ∼750 years BP, with exceptions including ecosystems such as restiad bogs (Perry et al. 2014). However, studies from around the world have found species with high flammability in largely fire-free ecosystems, such as rainforests (Bowman et al. 2014; Calitz et al. 2015; Wyse et al. 2016; Potts et al. 2022). Indeed, Cui et al. (2020a) noted the high prevalence of high flammability among small-leaved species from the genus Dracophyllum in New Zealand despite lack of fire as a selective force. This high flammability was considered an incidental or emergent property of these species as they evolved traits, including serotiny, to survive cold, dry conditions during Pleistocene glacial oscillations (Bond et al. 2004; Cui et al. 2020a).
Conclusion
We quantified relationships among plant traits, specifically leaf nutrient concentrations, leaf morphological traits, whole-shoot traits, and shoot or whole-plant flammability across 29 taxa in sub-alpine vegetation, New Zealand. We found that plant flammability is correlated with many traits, and that traits are strongly conserved within lineages. We expected that leaf nutrient concentrations would be correlated with shoot flammability, based on their presence in compounds used as fire retardants in the textile or wood industry and to control wildfires (Gebke et al. 2020; Fang et al. 2021; Guo et al. 2021; Ozyhar et al. 2022). Although we found significant correlations between plant flammability and leaf nutrient concentrations (Mg, K, S, Ca, but not P), these were weaker than correlations between morphological traits and flammability. Furthermore, nutrient concentrations did not explain any unique variation in flammability. Hence, our study emphasises the importance of considering suites of traits, rather than individual traits, when studying flammability, highlighting the need for a comprehensive approach that includes morphological as well as nutrient traits. Our study adds to a growing body of evidence that plant flammability is evolutionarily conserved within lineages (Zhao et al. 2016; Cui et al. 2020a, 2020b), and we suggest that this is because flammability is strongly associated with other plant traits that are also conserved within lineages (Pérez-Harguindeguy et al. 2013). These relationships are complex, and we recommend further exploration, particularly in order to understand the phylogenetic relationships of leaf nutrient traits within the context of plant flammability research.
Data availability
Datafiles are available as supplementary information in the New Zealand Vegetation Survey (NVS) database held by Manaaki Whenua Landcare Research (https://nvs.landcareresearch.co.nz/data/upload?prompt=Upload%20details).
Declaration of funding
This project was funded by The Miss E L Hellaby Indigenous Grasslands Research Trust in the form of a PhD scholarship and operating costs for SB-M. Auckland University of Technology and the Royal Society Te Aparangi provided funding for additional operating and nutrient analysis costs.
Acknowledgements
We are grateful to The Miss E L Hellaby Indigenous Grasslands Research Trust, Auckland University of Technology (AUT) and Royal Society Te Aparangi for financial support. We acknowledge Roger Cresswell and Emily Huang from Lincoln University (LU) for conducting nutrient testing, Adrian Paterson from (LU) for evolutionary discussions, and Kevin Lee from AUT for assisting with the analysis of the DNA sequence in Muscle. We extend our sincere thanks to the Department of Conservation (DOC) for permission to sample under Manaaki Whenua’s Global Concession Permit no. CA-31615-OTH at Arthur’s Pass National Park. We are incredibly thankful to our reviewers whose insightful comments helped us to improve this manuscript.
References
Alam MA, Wyse SV, Buckley HL, Perry GLW, Sullivan JJ, Mason NWH, Buxton R, Richardson SJ, Curran TJ (2020) Shoot flammability is decoupled from leaf flammability but controlled by leaf functional traits. Journal of Ecology 108(2), 641-653.
| Crossref | Google Scholar |
Anderson HE (1970) Forest fuel ignitibility. Fire Technology 6, 312-319.
| Crossref | Google Scholar |
Baum D (2008) Trait evolution on a phylogenetic tree: relatedness, similarity, and the myth of evolutionary advancement. Nature Education 1, 191 . Available at http://www.stevenpoe.net/uploads/3/7/3/4/37343605/baumcharactersontrees.pdf.
| Google Scholar |
Bell KL, Loeffler VM, Brosi BJ (2017) An rbcL reference library to aid in the identification of plant species mixtures by DNA metabarcoding. Applications in Plant Sciences 5(3), 1600110.
| Crossref | Google Scholar | PubMed |
Bond WJ, Dickinson KJM, Mark AF (2004) What limits the spread of fire-dependent vegetation? Evidence from geographic variation of serotiny in a New Zealand shrub. Global Ecology and Biogeography 13, 115-127.
| Crossref | Google Scholar |
Borcard D, Gillet F, Legendre P (2018) Canonical ordination. In ‘Numerical ecology with R’. (Eds D Borcard, F Gillet, P Legendre) pp. 203–297. (Springer International Publishing AG). 10.1007/978-3-319-71404-2_6
Bowman DM, French BJ, Prior LD (2014) Have plants evolved to self-immolate? Frontiers in Plant Science 5, 590.
| Crossref | Google Scholar | PubMed |
Braun U, Balabanovich AI, Schartel B, Knoll U, Artner J, Ciesielski M, Döring M, Perez R, Sandler JKW, Altstädt V, Hoffmann T, Pospiech D (2006) Influence of the oxidation state of phosphorus on the decomposition and fire behaviour of flame-retarded epoxy resin composites. Polymer 47(26), 8495-8508.
| Crossref | Google Scholar |
Buckley HL, Rafat A, Ridden JD, Cruickshank RH, Ridgway HJ, Paterson AM (2014) Phylogenetic congruence of lichenised fungi and algae is affected by spatial scale and taxonomic diversity. PeerJ 2, e573.
| Crossref | Google Scholar | PubMed |
Burge OR, Bellingham PJ, Arnst EA, Bonner KI, Burrows LE, Richardson SJ, Wiser SK, Wood JR, Wilmshurst JM (2020) Integrating permanent plot and palaeoecological data to determine subalpine post-fire succession, recovery and convergence over 128 years. Journal of Vegetation Science 31(5), 755-767.
| Crossref | Google Scholar |
Calitz W, Potts AJ, Cowling RM (2015) Investigating species-level flammability across five biomes in the Eastern Cape, South Africa. South African Journal of Botany 101, 32-39.
| Crossref | Google Scholar |
Cardoso AW, Oliveras I, Abernethy KA, Jeffery KJ, Lehmann D, Edzang NJ, McGregor I, Belcher CM, Bond WJ, Malhi YS (2018) Grass species flammability, not biomass, drives changes in fire behavior at tropical forest-savanna transitions. Frontiers in Forests and Global Change 1, 6.
| Crossref | Google Scholar |
Chukwunwike SA, Okafor KJ (2019) A review on some selected bio-based (green) flame retardants. Research & Reviews: Journal of Engineering and Technology 8(1), 38-43.
| Crossref | Google Scholar |
Cockayne L (1898) On the burning and reproduction of subalpine scrub and its associated plants; with special reference to Arthur’s Pass District. Transactions and Proceedings of the Royal Society of New Zealand 31, 398 . Available at https://paperspast.natlib.govt.nz/periodicals/TPRSNZ1898-31.2.6.1.33.
| Google Scholar |
Cockayne L, Calder JW (1932) The present vegetation of Arthur’s Pass (New Zealand) as compared with that of thirty-four years ago. Journal of Ecology 20(2), 270-283.
| Crossref | Google Scholar |
Cornwell WK, Elvira A, van Kempen L, van Logtestijn RS, Aptroot A, Cornelissen JH (2015) Flammability across the gymnosperm phylogeny: The importance of litter particle size. New Phytologist 206(2), 672-681.
| Crossref | Google Scholar | PubMed |
Cui X, Paterson AM, Alam MA, Wyse SV, Marshall K, Perry GLW, Curran TJ (2020a) Shoot-level flammability across the Dracophyllum (Ericaceae) phylogeny: evidence for flammability being an emergent property in a land with little fire. New Phytologist 228(1), 95-105.
| Crossref | Google Scholar |
Cui X, Paterson AM, Wyse SV, Alam MA, Maurin KJL, Pieper R, Padullés Cubino J, O’Connell DM, Donkers D, Bréda J, Buckley HL, Perry GLW, Curran TJ (2020b) Shoot flammability of vascular plants is phylogenetically conserved and related to habitat fire-proneness and growth form. Nature Plants 6(4), 355-359.
| Crossref | Google Scholar |
Cui X, Paterson AM, Perry GLW, Wyse SV, Alam MA, Huang C, Zhou S, Xiao L, Lai C, He F, Cao D, Marshall K, Curran TJ (2022) Intraspecific variation in shoot flammability in Dracophyllum rosmarinifolium is not predicted by habitat environmental conditions. Forest Ecosystems 9, 100017.
| Crossref | Google Scholar |
Dehane B, Hernando C, Guijarro M, Madrigal J (2017) Flammability of some companion species in cork oak (Quercus suber L.) forests. Annals of Forest Science 74(3), 60.
| Crossref | Google Scholar |
Delsuc F, Brinkmann H, Philippe H (2005) Phylogenomics and the reconstruction of the tree of life. Nature Reviews Genetics 6(5), 361-375.
| Crossref | Google Scholar | PubMed |
Díaz S, Kattge J, Cornelissen JHC, Wright IJ, Lavorel S, Dray S, Reu B, Kleyer M, Wirth C, Colin PI, Garnier E, Bönisch G, Westoby M, Poorter H, Reich PB, Moles AT, Dickie J, Gillison AN, Zanne AE, et al. (2016) The global spectrum of plant form and function. Nature 529(7585), 167-171.
| Crossref | Google Scholar | PubMed |
Domínguez MT, Aponte C, Pérez-Ramos IM, García LV, Villar R, Marañón T (2012) Relationships between leaf morphological traits, nutrient concentrations and isotopic signatures for Mediterranean woody plant species and communities. Plant and Soil 357(1), 407-424.
| Crossref | Google Scholar |
Edgar RC (2004) MUSCLE: Multiple sequence alignment with high accuracy and high throughput. Nucleic Acids Research 32(5), 1792-1797.
| Crossref | Google Scholar | PubMed |
Etlinger MG, Beall FC (2004) Development of a laboratory protocol for fire performance of landscape plants. International Journal of Wildland Fire 13(4), 479.
| Crossref | Google Scholar |
Fang Y, Yin X, Cui P, Wang X, Zhuang K, Ding Z, Xing F (2021) Properties of magnesium potassium phosphate cement-expanded perlite composites applied as fire resistance coating. Construction and Building Materials 293, 123513.
| Crossref | Google Scholar |
Funk JL, Larson JE, Vose G (2021) Leaf traits and performance vary with plant age and water availability in Artemisia californica. Annals of Botany 127(4), 495-503.
| Crossref | Google Scholar | PubMed |
Ganteaume A, Romero B, Fernandez C, Ormeño E, Lecareux C (2021) Volatile and semi-volatile terpenes impact leaf flammability: Differences according to the level of terpene identification. Chemoecology 31(4), 259-275.
| Crossref | Google Scholar |
Gebke S, Thümmler K, Sonnier R, Tech S, Wagenführ A, Fischer S (2020) Flame retardancy of wood fiber materials using phosphorus modified wheat starch. Molecules 25(2), 335.
| Crossref | Google Scholar | PubMed |
Gill AM, Zylstra P (2005) Flammability of Australian forests. Australian Forestry 68(2), 87-93.
| Crossref | Google Scholar |
Grootemaat S, Wright IJ, Bodegom PM, Cornelissen JHC, Cornwell WK, Schweitzer J (2015) Burn or rot: leaf traits explain why flammability and decomposability are decoupled across species. Functional Ecology 29(11), 1486-1497.
| Crossref | Google Scholar |
Guerrero F, Espinoza L, Carmona C, Blackhall M, Quintero C, Ocampo-Zuleta K, Paula S, Madrigal J, Guijarro M, Carrasco Y, Bustamante-Sánchez MA, Miranda A, Yáñez K, Bergmann J, Taborga L, Toledo M (2024) Unravelling the chemistry of plant flammability: exploring the role of volatile secondary metabolites beyond terpenes. Forest Ecology and Management 572, 122269.
| Crossref | Google Scholar |
Guild D, Dudfield M (2010) A history of fire in the forest and rural land- scape in New Zealand – Part 2, post-1830 influences, and implications for future fire management. Professional Paper 54, 31-38 Available at https://www.semanticscholar.org/paper/A-history-of-fire-in-the-forest-and-rural-landscape-Guild-Dudfield/6a3fbf63c2d2a7642f4c61452b5668982b32488f.
| Google Scholar |
Guo J, Zhou Z, Ming Q, Sun D, Li F, Xi J, Wu Q, Yang J, Xia Q, Zhao X (2021) Recovering chemical sludge from the zero liquid discharge system of flue gas desulfurization wastewater as flame retardants by a stepwise precipitation process. Journal of Hazardous Materials 417, 126054.
| Crossref | Google Scholar | PubMed |
Habte G, Hwang IM, Kim JS, Hong JH, Hong YS, Choi JY, Nho EY, Jamila N, Khan N, Kim KS (2016) Elemental profiling and geographical differentiation of Ethiopian coffee samples through inductively coupled plasma-optical emission spectroscopy (ICP-OES), ICP-mass spectrometry (ICP-MS) and direct mercury analyzer (DMA). Food Chemistry 212, 512-520.
| Crossref | Google Scholar | PubMed |
Horacek H, Grabner R (1996) Advantages of flame retardants based on nitrogen compounds. Polymer Degradation and Stability 54(2), 205-215.
| Crossref | Google Scholar |
Jaureguiberry P, Bertone G, Díaz S (2011) Device for the standard measurement of shoot flammability in the field. Austral Ecology 36(7), 821-829.
| Crossref | Google Scholar |
Lê S, Josse J, Husson F (2008) FactoMineR: an R package for multivariate analysis. Journal of Statistical Software 25, 1-18.
| Crossref | Google Scholar |
Leathwick J, Morgan F, Wilson G, Rutledge D, Mcleod M, Johnston K 2002 ‘Land environments of New Zealand: a technical guide.’ (Landcare Research New Zealand Limited) Available at https://www.landcareresearch.co.nz/uploads/public/Tools-And-Resources/Maps/LENZ/LENZ_Technical_Guide.pdf
Liberato CG, Barros JAVA, Virgilio A, Machado RC, Nogueira A, Nóbrega JA, Schiavo D 2017 ‘Determination of macro and micronutrients in plants using the Agilent 4200 MP AES’. pp. 1–5. (Agilent Technologies) Available at https://www.agilent.com/cs/library/applications/4200_MP-AES-5991-7856EN-plant-nutrients.pdf
Liodakis S, Bakirtzis D, Lois E, Gakis D (2002) The effect of (NH4)2HPO4 and (NH4)2SO4 on the spontaneous ignition properties of Pinus halepensis pine needles. Fire Safety Journal 37(5), 481-494.
| Crossref | Google Scholar |
Martin RR, Gordon DA, Gutierrez ME, Lee DM, Molina TD, Schroeder RA, Sapsis DB, Stephens SL, Chambers M (1994) Assessing the flammability of domestic and wildland vegetation. In ‘Proceedings of the 12th Conference on fire and forest meteorology’, 26–28 October 1993. (Society of American Foresters: Jekyll Island GA (USA)) 10.13140/RG.2.1.3999.3680
Mason NWH, Frazao C, Buxton RP, Richardson SJ (2016) Fire form and function: Evidence for exaptive flammability in the New Zealand flora. Plant Ecology 217, 645-659.
| Crossref | Google Scholar |
McGlone MS (2001) The origin of the indigenous grasslands of southeastern South Island in relation to pre-human woody ecosystems. New Zealand Journal of Ecology 25(1), 15 . Available at https://www.researchgate.net/profile/Matt-Mcglone/publication/253762174_The_origin_of_native_grasslands_of_southeastern_South_Island_in_relation_to_pre-human_woody_ecosystems/links/00b7d5329fd1c3b11c000000/The-origin-of-native-grasslands-of-southeastern-South-Island-in-relation-to-pre-human-woody-ecosystems.pdf.
| Google Scholar |
Murray BR, Hardstaff LK, Phillips ML (2013) Differences in leaf flammability, leaf traits and flammability-trait relationships between native and exotic plant species of dry sclerophyll forest. PLoS One 8(11), e79205.
| Crossref | Google Scholar | PubMed |
National Institute of Water and Atmospheric Research (NIWA) (2021) The national climate database, national institute of water and atmospheric research. CliFlo: NIWA’s National Climate Database on the Web. Available at https://cliflo.niwa.co.nz
Oksanen J, Simpson GL, Blanchet FG, Kindt R, Legendre P, Minchin PR, O’Hara RB, Solymos P, Stevens MHH, Szoecs E, Wagner H, Barbour M, Bedward M, Bolker B, Borcard D, Carvalho G, Chirico M, Caceres MD, Durand S et al. (2022) vegan: Community ecology package (2.6-2) [Computer software]. Available at https://CRAN.R-project.org/package=vegan
Ormeño E, Ruffault J, Gutigny C, Madrigal J, Guijarro M, Hernando C, Ballini C (2020) Increasing cuticular wax concentrations in a drier climate promote litter flammability. Forest Ecology and Management 473, 118242.
| Crossref | Google Scholar |
Ozyhar T, Tschannen C, Thoemen H, Zoppe JO (2022) Evaluating the use of calcium hydrogen phosphate dihydrate as a mineral-based fire retardant for application in melamine-urea-formaldehyde (MUF)-bonded wood-based composite materials. Fire and Materials 46(3), 595-604.
| Crossref | Google Scholar |
Padullés Cubino J, Buckley HL, Day NJ, Pieper R, Curran TJ (2018) Community-level flammability declines over 25 years of plant invasion in grasslands. Journal of Ecology 106(4), 1582-1594.
| Crossref | Google Scholar |
Pausas JG, Alessio GA, Moreira B, Segarra-Moragues JG (2016) Secondary compounds enhance flammability in a Mediterranean plant. Oecologia 180(1), 103-110.
| Crossref | Google Scholar | PubMed |
Pere K, Mburu K, Muge EK, Wagacha JM, Nyaboga EN (2023) Molecular discrimination and phylogenetic relationships of Physalis species based on ITS2 and rbcL DNA barcode sequence. Crops 3(4), 302-319.
| Crossref | Google Scholar |
Pérez-Harguindeguy N, Díaz S, Garnier E, Lavorel S, Poorter H, Jaureguiberry P, Bret-Harte MS, Cornwell WK, Craine JM, Gurvich DE, Urcelay C, Veneklaas EJ, Reich PB, Poorter L, Wright IJ, Ray P, Enrico L, Pausas JG, de Vos AC, et al. (2013) New handbook for standardised measurement of plant functional traits worldwide. Australian Journal of Botany 61(3), 167-234.
| Crossref | Google Scholar |
Perry GLW, Wilmshurst JM, McGlone MS (2014) Ecology and long term history of fire in New Zealand. New Zealand Journal of Ecology 38(2), 157-176 Available at https://www.jstor.org/stable/24060795.
| Google Scholar |
Pickett BM, Isackson C, Wunder R, Fletcher TH, Butler BW, Weise DR (2009) Flame interactions and burning characteristics of two live leaf samples. International Journal of Wildland Fire 18(7), 865-874.
| Crossref | Google Scholar |
Popović Z, Bojović S, Marković M, Cerdà A (2021) Tree species flammability based on plant traits: a synthesis. Science of The Total Environment 800, 149625.
| Crossref | Google Scholar | PubMed |
Potts E, Tng D, Apgaua D, Curran TJ, Engert J, Laurance SGW (2022) Growth form and functional traits influence the shoot flammability of tropical rainforest species. Forest Ecology and Management 522, 120485.
| Crossref | Google Scholar |
R Core Team (2023) ‘A language and environment for statistical computing.’ (R foundation for statistical computing: Vienna, Austria) Available at https://www.R-project.org/
Reich PB, Oleksyn J (2004) Global patterns of plant leaf N and P in relation to temperature and latitude. Proceedings of the National Academy of Sciences of the United States of America 101(30), 11001-11006.
| Crossref | Google Scholar | PubMed |
Santacruz-García AC, Bravo S, del Corro F, Ojeda F (2019) A comparative assessment of plant flammability through a functional approach: the case of woody species from Argentine Chaco region. Austral Ecology 44(8), 1416-1429.
| Crossref | Google Scholar |
Scarff FR, Westoby M (2006) Leaf litter flammability in some semi-arid Australian woodlands. Functional Ecology 20(5), 745-752.
| Crossref | Google Scholar |
Scarff FR, Westoby M (2008) The influence of tissue phosphate on plant flammability: a kinetic study. Polymer Degradation and Stability 93(10), 1930-1934.
| Crossref | Google Scholar |
Scarff FR, Gray BF, Westoby M (2012) Exploring phosphate effects on leaf flammability using a physical chemistry model. International Journal of Wildland Fire 21(8), 1042-1051.
| Crossref | Google Scholar |
Scarff FR, Lenz T, Richards AE, Zanne AE, Wright IJ, Westoby M (2021) Effects of plant hydraulic traits on the flammability of live fine canopy fuels. Functional Ecology 35(4), 835-846.
| Crossref | Google Scholar |
Schafer JL, Mack MC (2018) Nutrient limitation of plant productivity in scrubby flatwoods: does fire shift nitrogen versus phosphorus limitation? Plant Ecology 219(9), 1063-1079.
| Crossref | Google Scholar |
Schliep K, Potts AJ, Morrison DA, Grimm GW (2017) Intertwining phylogenetic trees and networks. Methods in Ecology and Evolution 8(10), 1212-1220.
| Crossref | Google Scholar |
Schwilk DW (2015) Dimensions of plant flammability. New Phytologist 206(2), 486-488.
| Crossref | Google Scholar | PubMed |
Slowikowski K, Schep A, Hughes S, Dang TK, Lukauskas S, Irisson JO, Kamvar ZN, Ryan T, Christophe D, Hiroaki Y, Gramme P, Abdol AM, Barrett M, Cannoodt R, Krassowski M, Chirico M, Aphalo P (2021) ggrepel: automatically position non-overlapping text labels with ‘ggplot2’ (0.9.1) [Computer software]. Available at https://CRAN.R-project.org/package=ggrepel
Thorne MS, Skinner QD, Smith M, Rodgers JD, Laycock WA, Cerekci SA (2002) Evaluation of a technique for measuring canopy volume of shrubs. Journal of Range Management 55(3), 235.
| Crossref | Google Scholar |
Tumino BJ, Duff TJ, Goodger JQD, Cawson JG (2019) Plant traits linked to field-scale flammability metrics in prescribed burns in Eucalyptus forest. PLoS One 14(9), e0223401.
| Crossref | Google Scholar |
USEPA (1996) ‘Method 3050B: acid digestion of sediments, sludges, soils (Revision 2).’ (USEPA) Available at http://www.greenrivertech.com.tw/stand-method-pdf/rohs/EPA3050b.pdf
Wang Z, Huang H, Wang H, Peñuelas J, Sardans J, Niinemets Ü, Niklas KJ, Li Y, Xie J, Wright IJ (2022) Leaf water content contributes to global leaf trait relationships. Nature Communications 13(1), 5525.
| Crossref | Google Scholar | PubMed |
Wardle P (1964) Facets of the distribution of forest vegetation in New Zealand. New Zealand Journal of Botany 2(4), 352-366.
| Crossref | Google Scholar |
White RH, Zipperer WC (2010) Testing and classification of individual plants for fire behaviour: plant selection for the wildland–urban interface. International Journal of Wildland Fire 19(2), 213-227.
| Crossref | Google Scholar |
Wickham H (2016) ‘Ggplot2: elegant graphics for data analysis.’ (Springer-Verlag New York) Available at https://ggplot2.tidyverse.org/
Wyse SV, Perry GLW, O’Connell DM, Holland PS, Wright MJ, Hosted CL, Whitelock SL, Geary IJ, Maurin KJL, Curran TJ (2016) A quantitative assessment of shoot flammability for 60 tree and shrub species supports rankings based on expert opinion. International Journal of Wildland Fire 25(4), 466-477.
| Crossref | Google Scholar |
Wyse SV, Perry GLW, Curran TJ (2018) Shoot-level flammability of species mixtures is driven by the most flammable species: implications for vegetation-fire feedbacks favouring invasive species. Ecosystems 21(5), 886-900.
| Crossref | Google Scholar |
Yan L, Xu Z, Liu D (2019) Synthesis and application of novel magnesium phosphate ester flame retardants for transparent intumescent fire-retardant coatings applied on wood substrates. Progress in Organic Coatings 129, 327-337.
| Crossref | Google Scholar |
Yang X, Huang Z, Zhang K, Cornelissen JHC (2017) Taxonomic effect on plant base concentrations and stoichiometry at the tips of the phylogeny prevails over environmental effect along a large scale gradient. Oikos 126(9), 1241-1249.
| Crossref | Google Scholar |
Zhang Y, Deng T, Sun L, Landis JB, Moore MJ, Wang H, Wang Y, Hao X, Chen J, Li S, Xu M, Puno PT, Raven PH, Sun H (2021) Phylogenetic patterns suggest frequent multiple origins of secondary metabolites across the seed-plant ‘tree of life’. National Science Review 8(4), nwaa105.
| Crossref | Google Scholar | PubMed |
Zhao W, Cornwell WK, van Pomeren M, van Logtestijn RS, Cornelissen JH (2016) Species mixture effects on flammability across plant phylogeny: the importance of litter particle size and the special role for non-Pinus Pinaceae. Ecology and Evolution 6(22), 8223-8234.
| Crossref | Google Scholar | PubMed |