Exploring the impact of airtanker drops on in-stand temperature and relative humidity
Melanie Wheatley A B # * , Anne Cotton-Gagnon C # , Jonathan Boucher C , B. Mike Wotton B D , Colin B. McFayden A D , Natasha Jurko D and Jason Robinson AA Ontario Ministry of Natural Resources and Forestry, Aviation, Forest Fire and Emergency Services, Dryden Fire Management Centre, 95 Ghost Lake Road, P.O. Box 850, Dryden, ON P8N 2Z5, Canada.
B Institute of Forestry and Conservation, John H. Daniels Faculty of Architecture, Landscape and Design, University of Toronto, 33 Willcocks Street, Toronto, ON M5S 3B3, Canada.
C Laurentian Forestry Centre, Canadian Forest Service, Natural Resources Canada, 1055 rue du P.E.P.S., P.O. Box 10380, Québec City, QC G1V 4C7, Canada.
D Great Lakes Forestry Centre, Canadian Forest Service, Natural Resources Canada, 1219 Queen Street E., Sault Ste. Marie, ON P6A 2E5, Canada.
International Journal of Wildland Fire 32(8) 1269-1276 https://doi.org/10.1071/WF22218
Submitted: 18 November 2022 Accepted: 17 May 2023 Published: 2 June 2023
© 2023 The Author(s) (or their employer(s)). Published by CSIRO Publishing on behalf of IAWF. This is an open access article distributed under the Creative Commons Attribution 4.0 International License (CC BY).
Abstract
Background: There has been little quantification of the extent and duration of micrometeorological changes within a forest after airtanker drops of water-based suppressant. It has been speculated that a period of prolonged relative humidity – referred to as a ‘relative humidity (RH) bubble’ – temporarily exists in the canopy understorey post-drop.
Aims: We quantify the RH bubble from the drops of five airtankers commonly used by wildland fire management organisations in Canada.
Methods: We measured airtankers dropping water, foam concentrates, and gel enhancers in a mature jack pine stand. We examined the duration of change in RH and temperature using Generalised Additive Models, and the consequence of these changes on fine fuel moisture.
Key results: The average maximum RH increased and temperature decreased, indicating that the effects of the ‘RH bubble’ in-stand lasted from 25 to 76 min, depending upon the airtanker type and load configuration.
Conclusion: Airtanker drops cause an in-stand increase in RH and decrease in temperature, but this ambient change has a limited effect on potential fire behaviour.
Implications: The direct effect of water wetting the fuel is the most impactful effect of an airtanker drop. The ‘RH bubble’ created, though observable, does not change fine fuel moisture enough to impact fire behaviour.
Keywords: aerial suppression, enhancer, fine fuel moisture, fire management, general additive model, humidity dome, mature jack pine, RH bubble.
Introduction
The objective of airtanker suppression on a wildland fire is to facilitate ground crew action by reducing fire intensity, or to help slow the rate of fire spread or steer the fire away from an area. These objectives are met by direct attack, where suppression drops are applied directly onto the combustion zone, or through indirect attack, where airtanker drops are positioned ahead of the flaming perimeter in unburned fuels. Airtankers increase the fuel moisture ahead of the fire with either water, foam, or gel, creating a temporary barrier to resist fire spread. When airtankers use indirect suppression tactics, the suppressant falls onto the forest canopy, wetting unburned surface fuels below. The amount of canopy infiltration with suppressant is dependent upon the forest structure and density of the stand.
It has been suggested that another effect of an airtanker drop, aside from directly reducing the energy in the combustion zone, is the creation of a micrometeorological condition within an unburned forested area commonly described as a ‘humidity dome’, or ‘relative humidity (RH) bubble’, as referred to herein. This ‘RH bubble’ is thought to be a volume of cooler and wetter air within the forest that raises the moisture of fuels within it, thereby reducing their combustion rate. The concept of a micro-environment caused by an increased RH due to water vapour has been explored using sprinkler systems, where Gibos and Hvenegaard (2009) measured the change of moisture content in an air mass surrounded by a sprinkler system in an open field or closed canopy forest. However, their findings suggested that an air mass is not receptive for retaining additional water vapour, and that winds were the most influential factor in the movement of airborne moisture. The direct influence of sprinkler-watering on fuel moisture has also been investigated (Barnes 2017; Barnes et al. 2017; Miller and Barnes 2018), specifically the change in fuel moisture represented by the Fine Fuel Moisture Code (FFMC) and Duff Moisture Code (DMC) in the Canadian Forest Fire Weather Index (FWI) System (Van Wagner 1987). Barnes (2017) found that the influence of direct water application from sprinklers was responsible for an increase in fuel moisture; however, they did not examine how sprinklers influenced RH and temperature and their respective/subsequent effects on fine fuel moisture.
In this study we explore the creation of an RH bubble from the drops of four different fixed-wing skimmer airtankers and one rotary-wing airtanker commonly used for fire suppression throughout the boreal forest. Specifically, we investigate the following: (1) whether an airtanker drop creates a distinguishable RH bubble (i.e. by reducing the in-stand ambient temperature and raising the in-stand RH); (2) how long this RH bubble lasts; and (3) how the altered environment within the RH bubble affects the surface fuel moisture as represented by the FFMC. In doing so, we explore the differences in the first two listed metrics between water, foam concentrates, and gel enhancers that are commonly used for airtanker suppression in Canada.
Methods
Field experiment
The field experiments were conducted during the summers of 2018 and 2019 as a collaboration between the Ontario Ministry of Natural Resources and Forestry (OMNRF) and the Canadian Forest Service (CFS).1 A mature homogeneous jack pine (pinus banksiana Lamb.) stand with moderate tree density (classified as a C-3 stand by the Canadian Forest Fire Behaviour Prediction (FBP) System; Forestry Canada Fire Danger Group (1992)) (Fig. 1a) was selected outside of Dryden, Ontario, Canada. We installed 44 ‘Measurement Computing’ USB-502 sensors, affixed to a wooden field stake at a height of approximately 1 m above the forest floor (Fig. 1b), sheltered by a Styrofoam cup to mitigate the chance of water from airtanker drops hitting the sensors directly. These sensors recorded RH and temperature at 1-min intervals in a grid configuration spaced relatively evenly, with sensors covering approximately 180 m by 45 m in the C-3 stand (Fig. 1c). In 2018, the grid included 22 sensors, covering the same surface area, but sensors were further apart. Three other sensors were installed in the same stand away from the drop zone by 50–100 m to establish a control baseline measure of RH and temperature. These control sensors were deployed to measure local untreated air mass conditions before, during, and after each drop to compare with the treated drop zone air mass conditions. The airtanker drops were conducted under average weather conditions for the months of June and July in Dryden, Ontario, with the average temperature throughout the campaign being 25°C and average wind speed 9 km/h.2
(a) Photograph of mature jack pine stand (C-3) where experiment was conducted. Stand was moderate stocked mature jack pine in the boreal forest of Canada. Overall height was 19.5 m with an estimated crown closure of 90%. (b) Example sensor set-up with the USB-502 sensor. (c) Layout of grid covering an area of 180 m by 45 m with 44 sensors monitoring RH and temperature. This drop example is from a CL-215 heavy airtanker using foam additives. The coverage levels were determined from an interpolation of water cup data points collected in a larger experiment on airtanker drop effectiveness.
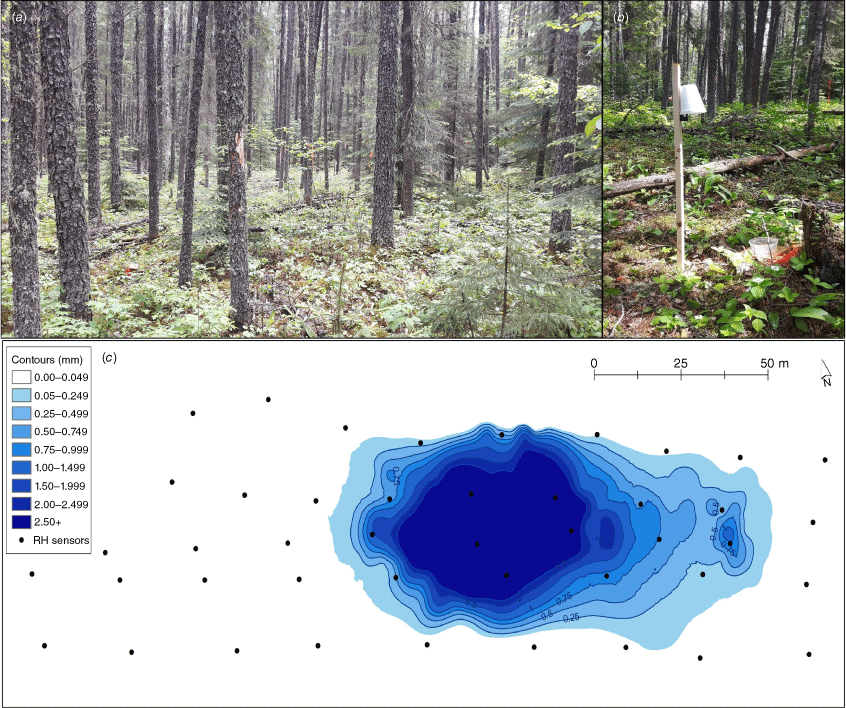
The four different fixed-wing aircraft tested were the CL-415 (four door bomb system, water capacity 6100 L), CL-215 (two door bomb system, water capacity 5400 L), AT-802F Fire Boss (water capacity 3000 L), and the DHC-6 Twin Otter (water capacity 2091 L) equipped with Wipaire 13000 Series Floats with OMNRF bombing conversion. A rotary-wing suppression aircraft, the Bell 412 (B412), was also tested and equipped with a Simplex Model 304 Fire Attack system (water capacity 1400 L). We classified the five airtankers, based on suppressant litres and/or physical size of the airtanker. For the purposes of this study, a light airtanker includes the B412 helicopter, an intermediate airtanker includes the DH-6 Twin Otter and AT-802 Fire Boss, and a heavy airtanker includes the CL-215 and CL-415. All aircraft were given standard flight parameters, which was drop height typically 46 m (150 ft) Above Ground Level (AGL) for fixed-wing airtankers and approximately 32 m (105 ft) AGL for the B412. Drop speed was approximately 100 knots for the fixed wing airtankers and 20–40 knots for the B412. All airtankers dropped in the same direction across the experiment plot, with full tanks, and a full salvo drop (i.e. when all doors are released at the same time, particularly for CL-415 and CL-215 airtankers). We examined the use of water, Class A foam additives commonly used by Canadian fire management agencies, and polymer-based and polyacrylate copolymer water additives (i.e. gel). For the Class A foam, different concentration percentages can be used (i.e. 0.1% ‘wet water’, 0.3% ‘standard ratio’, 0.5% ‘heavy foam’), which change the viscosity of the load. For this experiment the airtankers used both 0.3 and 0.5% concentrations. Not all five airtanker types were tested for each enhancer type because some aircraft could not equip certain additives as per the OMNRF standard operating procedures.
Data analysis
Not all sensor data or airtanker drops could be used in the data analysis based on several criteria that may have influenced the drop effect on RH and temperature. First, some drops were eliminated because the RH within the site was already elevated from prior airtanker drops, resulting in the drop having little impact on it. Second, drops that fell outside the study area or where too few sensors were directly in the drop footprint were eliminated. Third, some individual sensors were eliminated because they were malfunctioning in a way that affected normalisation of the data.
We examined both the magnitude of change and its duration for RH and temperature using time series data for 30 min prior to the drop time and up to 90 min after the drop. The size of the drop footprints for each aircraft differed, and drops were not necessarily centred on the grid. In-stand sensors that were within the airtanker drop footprint, as defined by any sensor within an interpolated contour level with a depth of liquid greater than 0.05 mm (Fig. 1c), were selected to compare with the three control sensors. These interpolated contour depths were derived from data collection from a larger experiment, which involved collecting water/enhancer drops in cups pre-positioned within a measured grid along the forest floor. There were 480 cups placed throughout the same area as the sensors, with approximately six cups between each sensor. Within each drop there was considerable variability in the individual time series observed from the sensors (Fig. 2a). We created an overall single time series of RH and temperature for each drop, using a Generalised Additive Model (GAM). The data were normalised between control and treatment using the 30 min prior to a suppressant drop.
(a) Example of the raw time series data for both RH and temperature from a CL-215 airtanker water drop. (b) GAM model results of RH and temperature fluctuations for the duration of time analysed for the same drop. Ribbons represent the ±2 standard error.
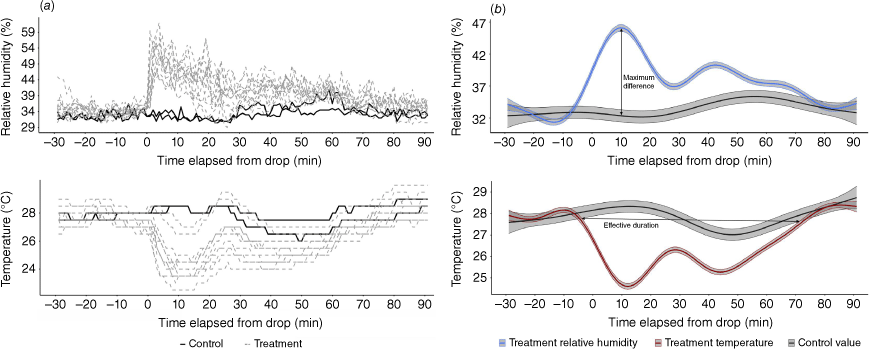
To estimate the magnitude of RH and temperature effects, the maximum difference between control sensors and treatment sensors as predicted by the GAM were extracted (Fig. 2b). The duration of the RH bubble effect (i.e. the temporal effect of the airtanker drop) for RH was determined to end when the difference between the lower bound of the treatment and upper bound of the control was smaller than the mean standard error of the control sensors, or less than the sensors precision of 0.5% RH. Similarly for temperature, the effect was determined to end when the difference between the lower bound of the control and upper bound of the treatment was smaller than the mean standard error of the control sensors, or less than the sensors precision of 0.5°C temperature (Fig. 2b). If this threshold was never met, we determined the RH bubble duration ended after 90 min.
Logistical constraints of the overall airtanker drop experiment prevented us from directly sampling change in moisture of forest floor litter before and after drops. Airtanker drops were often carried out when feathermoss was still wet from previous drops, thus the intensive fuel moisture sampling required for precise, paired comparisons was not possible. Therefore, we explored the potential impact of a drop, and the RH bubble created by it, on a dry closed canopy conifer forest floor bed using the Hourly Fine Fuel Moisture Code (HFFMC) calculation (Van Wagner 1977) from the Canadian Forest Fire Danger Rating System (CFFDRS). This involved exploring the impacts on fuel litter moisture, represented by the FFMC, of two scenarios: (1) elevated RH and lowered temperature (consistent with the maximum effects observed in the RH bubble), with no wetting effect; and (2) this same RH bubble in scenario 1, along with the wetting fuels impact (i.e. 0.5 mm of water). A scenario in which the pre-drop conditions supported vigorous burning was used: air temperature was 25°C, RH was 25%, and wind speed was 10 km/h. These conditions correspond to a head fire intensity of about 3000 kW/m, equivalent to the mid-range of Intensity Class 4, in the FBP System’s mature jack pine fuel type (C-3) (Forestry Canada Fire Danger Group 1992). Observed maximum RH and temperature change from the RH bubble was then applied to create scenarios of moisture content change in the HFFMC of a jack pine forest.
Results and discussion
Post-drop RH and temperature
The airtanker drop data show the creation of a micrometeorological condition with both RH and temperature, and this was evident in most of the analysed drops. The average maximum RH increased, and temperature decreased for the different airtanker types and enhancers used. The in-stand RH bubble effects seemed to last for up to about an hour (Table 1), and on average, heavy airtankers maximum temperature decrease was about 3°C and was associated with a RH increase of approximately 16%. This drop in temperature is due to the cooler lake water of 10–15°C being dropped into a warmer forest. Without any change in absolute amount of moisture in the air, in an air mass that started at a temperature of 25°C and an RH of 40%, a drop in air temperature of 3°C would correspond to a rise in RH of approximately 8%. Therefore, the change in RH within the stand is likely associated with both the observed temperature drop and the addition of moisture into the local air in the stand. These airtanker drop effects eventually stabilise back to baseline within 90 min of delivery.
Enhancer | Aircraft | Number of observations | Relative humidity (%) | Temperature (°C) | ||
---|---|---|---|---|---|---|
Mean maximum difference | Mean duration of effect (min) | Mean maximum difference | Mean duration of effect (min) | |||
None (water) | CL-415 | 2 | 16 ± 2.7 | 62 ± 28 | 3.0 ± 0.3 | 59 ± 31 |
CL-215 | 2 | 15 ± 1.2 | 79 ± 9 | 3.3 ± 0.4 | 76 ± 15 | |
Total heavy airtanker | 4 | 16 ± 1.2 | 71 ± 13 | 3.2 ± 0.2 | 67 ± 15 | |
AT 802 Fire Boss | 4 | 9 ± 1.0 | 51 ± 3 | 1.6 ± 0.4 | 25 ± 9 | |
DH6 Twin Otter | 6 | 12 ± 1.8 | 62 ± 10 | 1.0 ± 0.4 | 45 ± 10 | |
Total intermediate airtanker | 10 | 11 ± 1.2 | 58 ± 6 | 1.8 ± 0.3 | 37 ± 7 | |
Foam | CL-415 | 1 | 13 | 90 | 1.6 | 32 |
CL-215 | 4 | 10 ± 3.0 | 60 ± 11 | 1.5 ± 0.5 | 32 ± 21 | |
Total heavy airtanker | 5 | 11 ± 2.4 | 66 ± 11 | 1.5 ± 0.4 | 32 ± 16 | |
B412 (light airtanker) | 4 | 12 ± 2.8 | 62 ± 16 | 2.7 ± 0.2 | 65 ± 11 | |
Gel | AT 802 Fire Boss (intermediate airtanker) | 4 | 9 ± 2.2 | 65 ± 15 | 2.0 ± 0.3 | 51 ± 14 |
The maximum difference and duration of effect for RH and temperature were compared among different airtanker load capacities (Fig. 3). Intuitively, we would expect airtankers with a higher load capacity to result in a greater maximum difference of effect and a longer duration of hold, but our results do not consistently show this. Generally, our results show that foam has less of an effect than water for both the maximum effect and duration of hold. Although for intermediate airtankers, gel resulted in an increase effect for almost all metrics, except for maximum effect of temperature. For water, we see that the heavy airtankers had a greater effect than intermediate airtankers for both RH and temperature. However, for foam we see the opposite pattern: the light airtanker (i.e. B412) has a greater effect for both RH and temperature than the heavy skimmer airtanker grouping. We completed four different Analysis of Variance (ANOVA) comparisons for each of our four outcome metrics (i.e. maximum effect of RH, duration of effect of RH, maximum effect of temperature, and duration of effect of temperature) with airtanker category (i.e. light, intermediate, and heavy) and enhancer type (i.e. water, foam, and gel). We found strong evidence of an association between maximum effect of temperature with airtanker category (P = 0.024) and enhancer (P = 0.015). Tukey post hoc tests revealed that heavy airtankers had a greater maximum effect of temperature than intermediate (P < 0.01), but light airtankers had a greater effect than both heavy (P = 0.04) and intermediate airtankers (P < 0.01). These findings are consistent with Fig. 3b and can be due to limited replicates of drops; however, we also note that aircraft speed and drop height plays an important role in the ability of an airtanker drop to penetrate the forest canopy; therefore, drops from the slower moving, lower altitude rotary-wing aircraft would be expected to be more concentrated into a smaller footprint. For the enhancer type contrasts, both gel (P = 0.02) and water (P < 0.01) had a greater maximum effect of temperature than foam. There was no evidence of differences between water and gel.
Mean maximum difference between control and in-stand sensors compared with the mean duration of effect for both (a) RH and (b) temperature, with error bars representing the standard error. The airtankers are grouped into three categories based on load capacity: light (B412), intermediate (Twin Otter, 802 Fire Boss), and heavy (CL-215, CL-415).
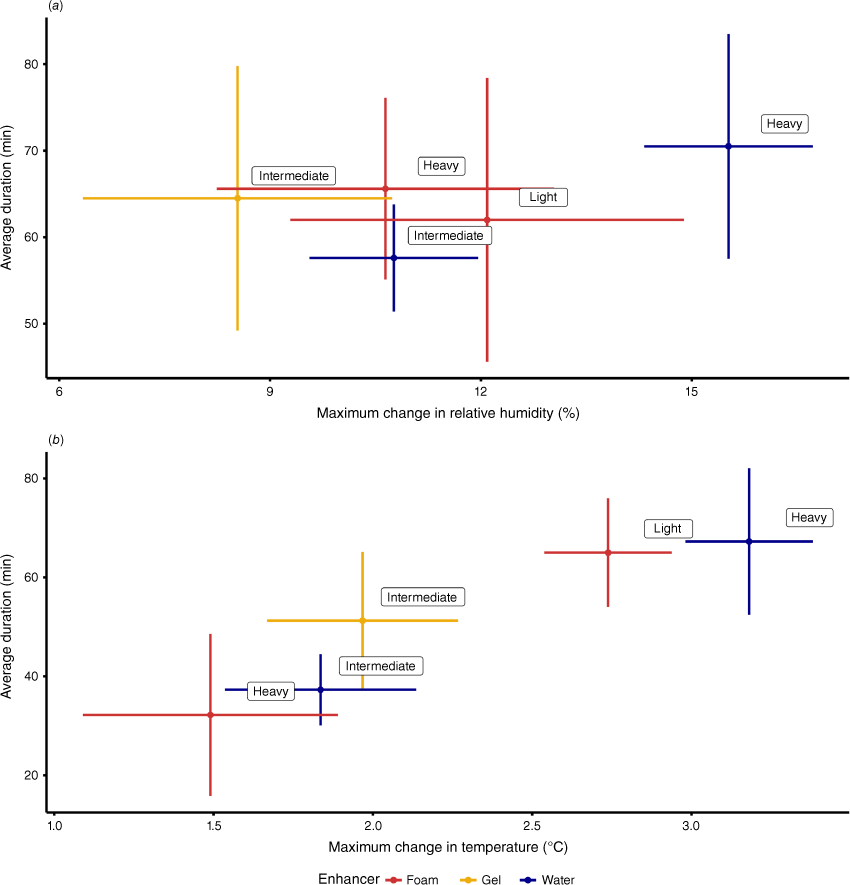
Although there is considerable variability in these observations, our findings suggest that water seems to have the most effective ability to create this RH and temperature micrometeorological condition. However, there were limitations in the data collection that may have impacted the ability to find meaningful differences in the enhancers’ ability to create this RH bubble. Specifically for the foam data collection, our standard water cup measuring method (Thomasson 2012) was not applicable for the physical composition of foam, so we measured the depth of foam in each cup using a ruler instead. This calibration method identified sensors that should be incorporated into our estimated drop extent, which determined the RH sensors included in the analysis; we do not believe the result was sensitive to this outcome. In addition, wind influenced placement of the airtanker drop and the amount of drift associated with it, which may have affected the strength of the RH bubble. We also recognise that skimmer airtankers often work in circuits where multiple airtankers will drop simultaneously after one another. We did not analyse the influence of subsequent drops on the compound effects of in-stand RH and temperature.
Influence of post-drop RH and temperature on fuel moisture
To simulate the effects of the RH bubble on litter moisture, we created a scenario similar to the effect of a heavy airtanker water drop in Fig. 3, where at 1500 hours, a drop increased humidity instantly by 15% and decreased the air temperature by 3°C for the hour after the drop. For the next 2 h, we followed the recovery of moisture content in needle litter using the HFFMC model (Fig. 4), which started prior to the drop at an equilibrium moisture content of about 8% (FFMC = 93). At 1600 hours, the in-stand atmosphere returned to pre-drop conditions and the RH bubble effects were gone (middle path). We added direct wetting effect of water from the drop into the moisture calculation (Fig. 4, rightmost path). We assumed the equivalent of 0.5 mm of rain penetrated the canopy and fell onto surface fuels at 1500 hours and followed drying of the HFFMC for 2 h. The atmospheric effects of the RH bubble on their own for 1 h do not change fuel moisture appreciably (Fig. 4); the small reduction in litter moisture shown in this scenario would not influence fire behaviour significantly. In the second scenario (i.e. direct water influence), even though the HFFMC model assumes only a small fraction (~7%) of that direct water amount (i.e. 0.5 mm) is absorbed, moisture content changes significantly enough to cause a substantial change in fire behaviour. Under these ambient conditions represented in Fig. 4 (i.e. temperature = 25°C, RH = 25%, wind speed = 10 km/h), the time lag in drying of the litter layer modelled in the HFFMC is about 5 h. A fuel with a faster recovery rate, like the 1-h time lag fuel moisture model used in the US NFDRS (Deeming et al. 1977), would recover from the added 0.5 mm of water faster; however, it would still only reach a moisture content of 10.3% by 1800 hours, so would still be exhibiting some reduction in spread rate and fire intensity compared with the control conditions (Fig. 4, leftmost path), where moisture content was 8%.
The change in fuel moisture as represented by the hourly FFMC for three scenarios: no airtanker drop (control conditions; left); a change in temperature and RH conditions without wetting effect (centre); and the change due in ambient conditions along with a wetting effect equivalent to 0.5 mm of precipitation (right). Moisture content percentages are below the FFMC values in parentheses. Before-drop weather conditions were: temperature, 25°C; RH, 25%; wind speed, 10 km/h.
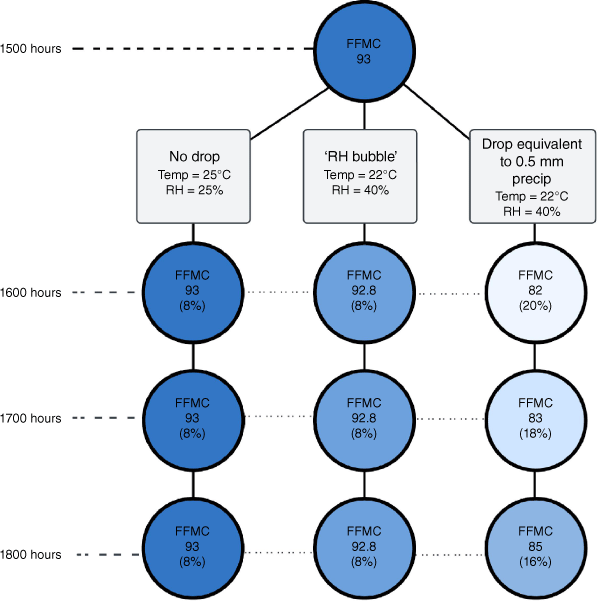
Conclusion and implications
We showed that there is indeed an observable RH bubble (i.e. an in-stand increase in RH and decrease in temperature) after a water-based suppressant drop from airtankers; however, these relatively small, short-lived (<1 h) changes in ambient conditions are not likely, by themselves, to have much of an effect on reducing fire behaviour. We found little consistent difference in the average micrometeorological changes for in-stand air temperature and RH with drops from different airtanker types and when comparing foam versus gel water enhancers. Fire response staff should be aware that although a micrometeorological environment is created after an indirect suppression airtanker drop, these effects may only last up to an hour, and situational awareness should be maintained. The primary impact on fire behaviour, reducing fire spread, fire intensity, and spot fire potential, is not due to the RH bubble itself but rather from the direct absorption of water into fuels.
Acknowledgements
The OMNRF provided support and coordination of the drop trials. A special thank you to the Aviation, Forest Fire and Emergency Services (AFFES) Management Team, the Provincial Aerial Fire Operations Unit, Aviation Services and the Integrated Services Section. We recognise the support of the following groups: the Dryden Fire Management Headquarters and FireRanger crews, the AFFES Summer Experience Program students who participated in the preparation and carrying out drop trials, and specifically to Benito Russo who helped plan, coordinate, and execute the drop trials. Additionally, we recognise support and advice from R. Hsieh and R. Refai of FPInnovations. Without the support of the City of Dryden and the Dryden Regional Airport Authority this project would not have occurred. Lastly, thank you to the Minnesota Department of Natural Resources, Manitoba Sustainable Development Wildfire Program and the Société de protection des forêts contre le feu (SOPFEU).
References
Barnes DE (2017) Fuel moisture changes from Sprinkler-watering treatments in interior Alaska boreal forests. Master of Science in Protected Areas and Wildlands Management thesis, Department of Renewable Resources, University of Alberta, Edmonton, AB, Canada. 10.7939/R3QR4P322 [verified 23 September 2022]
Barnes DE, Baxter G, Miller E (2017) Moisture changes from sprinkler watering. Information note. (FP Innovations: Hinton, AB, Canada) Available at https://library.fpinnovations.ca/en/permalink/fpipub7309 [verified 26 April 2023]
Forestry Canada Fire Danger Group (1992) Development of the Canadian forest fire behavior prediction system. Information Report ST-X-3. (Forestry Canada) Available at https://cfs.nrcan.gc.ca/publications?id=10068
Gibos K, Hvenegaard S (2009) Exploratory research into the existence of humidity domes created by wildfire sprinklers. Fire Report. (FP Innovations: Hinton, AB, Canada) Available at https://library.fpinnovations.ca/en/permalink/fpipub9370 [verified 25 April 2023]
Miller E, Barnes D (2018) Quantification of sprinkler effects in Alaskan feathermoss fuel beds. (Alaska Fire Science Consortium) Available at https://www.frames.gov/catalog/56053 [verified 26 April 2023]
Thomasson J (2012) Improving the visual assessment method of measuring cup amounts in airtanker drop tests. Project Note. (FP Innovations: Hinton, AB, Canada) Available at https://library.fpinnovations.ca/en/permalink/fpipub9447 [verified 25 April 2023]
Van Wagner CE (1977) Method of Computing Fine Fuel Moisture Content Throughout the Diurnal Cycle. Information Report PS-X-69. (Canadian Forest Service: Ottawa, Canada). Available at https://cfs.nrcan.gc.ca/publications?id=25591 [verified 29 April 2023]