Short-term impacts of operational fuel treatments on modelled fire behaviour and effects in seasonally dry forests of British Columbia, Canada
Kea H. Rutherford
A
B
C
Abstract
In response to increasing risk of extreme wildfire across western North America, forest managers are proactively implementing fuel treatments.
We assessed the efficacy of alternative combinations of thinning, pruning and residue fuel management to mitigate potential fire behaviour and effects in seasonally dry forests of interior British Columbia, Canada.
Across five community forests, we measured stand attributes before and after fuel treatments in 2021 and 2022, then modelled fire behaviour and effects using the Fire and Fuels Extension to the Forest Vegetation Simulator.
For our study area, field measurements combined with fire behaviour modelling indicated: (1) low-intensity thinning from below reduced potential of passive crown fire, whereas high-intensity thinning reduced potential of passive and active crown fire; (2) pruning after thinning from below did not further reduce potential of passive crown fire; and (3) chipping or pile burning of residue fuel mitigated potential of passive crown fire, but fire effects associated with chipping remain a concern.
There is limited prior research on the impacts of fuel treatments in western Canada. This research contributes to better understanding the potential impacts of fuel treatments in the fire-prone forests of interior British Columbia.
Keywords: ecosystems: temperate, Fire and Fuels Extension, fire behaviour: modelling, Forest Vegetation Simulator, fuel: chipping, FVS-FFE, mitigation, pile burning, pruning, thinning, treatments, wildfire management, wildland–urban interface.
Introduction
Extreme fire seasons have become a central challenge to forest management in western North America (Hessburg et al. 2021; Jain et al. 2024). In many seasonally dry montane forest ecosystems, a century of land-use change and fire exclusion has resulted in widespread changes in forest structure and composition, including accumulation of surface fuels, ingrowth of shade-tolerant understorey trees and reduction in canopy gaps (Hessburg et al. 2005, 2019; Coogan et al. 2021; Hagmann et al. 2021). The increased abundance and continuity of fuels, in conjunction with warmer, drier and longer fire seasons, have left seasonally dry forest ecosystems susceptible to uncharacteristically large, high-severity wildfires (Hessburg et al. 2005, 2019; Parks and Abatzoglou 2020; Parisien et al. 2023). Exemplifying this emerging wildfire crisis, 2017, 2018, 2021, 2023 and 2024 were the five most impactful fire seasons in a century in British Columbia (BC), Canada (Parisien et al. 2023, Daniels et al. 2024). In 2023, 2,840,545 ha of forests burned, more than twice the area-burned records set in 2017 (1,215,685 ha) and 2018 (1,355,271 ha) and 10 times the 20-year average (284,001 ha; British Columbia Wildfire Service 2023a). These fire seasons caused prolonged evacuations, hazardous air quality and destruction of property (Forest Practices Board 2023), demonstrating the increasing vulnerability of rural communities in BC and the resulting need for proactive management strategies such as fuel reduction treatments (Dickson-Hoyle et al. 2023; Daniels et al. 2024).
In BC, community forest managers are at the forefront of wildfire risk reduction through proactive fuel treatments to reduce wildfire impacts on ecosystems and damage to human communities and critical infrastructure (Dickson-Hoyle et al. 2023). A Community Forest Agreement is a 25-year, area-based (360 to >160,000 ha) licence operating on public land that grants the exclusive right to harvest timber within the tenure (Devisscher et al. 2021). Community forests are managed by local governments, First Nations, or community-based organisations to reflect the values of the local community, including wildfire risk reduction (Copes-Gerbitz et al. 2020; Dickson-Hoyle et al. 2023). Community forest managers often have multiple objectives for wildfire risk reduction projects, including decreasing the potential of crown fire, lowering tree mortality in the advent of fire, aiding fire suppression in the wildland–urban interface to protect homes and infrastructure, and supporting forest restoration efforts (British Columbia Wildfire Service 2020; British Columbia Community Forest Association 2024).
Fuel treatments commonly include thinning, pruning and surface fuel management to reduce the abundance and continuity of fuels in order to mitigate fire behaviour and effects (Agee and Skinner 2005; Stephens et al. 2009). In this context, fire behaviour can be represented by passive and active crown fire, while fire effects can be represented by tree mortality. ‘Passive crown fire’ refers to the ignition of individual or small groups of trees, whereas ‘active crown fire’ describes tree-to-tree crown fire spread (Scott and Reinhardt 2001). In multi-layered stands, ‘thinning from below’ starts with the removal of smaller-diameter trees, then extends to successively larger-diameter trees to reach a specific retention criterion (e.g. remaining stems per hectare). Low-intensity thinning from below can mitigate the potential of passive crown fire by reducing ladder fuels that facilitate vertical fire propagation from the surface into the tree crowns (Van Wagner 1977; Agee and Skinner 2005; Scott and Reinhardt 2007; Keane 2015). Alternatively, high-intensity thinning from below that removes larger trees can mitigate the potential of passive and active crown fire by limiting the vertical and horizontal continuity of crown fuels (Van Wagner 1977; Agee and Skinner 2005; Harrod et al. 2009; Johnson et al. 2011). As well as thinning, remaining trees are sometimes pruned to further reduce ladder fuels (Scott and Reinhardt 2007). Thinning and pruning activities lead to elevated surface fuel loads as residue fuel (including small trees, treetops and branches) is scattered across the site, which is commonly ameliorated through pile burning or chipping (Safford et al. 2009; Kreye et al. 2014). Pile burning decreases fuel loading, whereas chipping rearranges fuels into a more compact structure (Kreye et al. 2014).
In this study, we differentiate treatment ‘efficacy’ and ‘effectiveness’ using terminology consistent with medical research (Compher 2010). Treatment ‘efficacy’ is assessed by comparing modelled fire behaviour and effects under specified weather conditions. In contrast, treatment ‘effectiveness’ assesses whether a fuel treatment changed fire behaviour and effects as intended when intercepted by an actual wildfire. There is an extensive legacy of research on the efficacy (Stephens et al. 2009) and effectiveness (Martinson and Omi 2013; Kalies and Yocom Kent 2016; Davis et al. 2024) of fuel reduction treatments in the western United States (US). In contrast, few published studies have assessed fuel treatment efficacy or effectiveness in western Canada (Gray and Blackwell 2008 in seasonally dry forests; Beverly et al. 2020 and Thompson et al. 2020 in boreal forests), although government-funded programs have supported fuel treatments in wildland–urban interface forests for two decades (Beverly et al. 2020; Daniels et al. 2020, 2024).
This study was a collaboration with five community forests in the seasonally dry montane forests of southeastern BC. Fuel treatments conducted as part of forest management operations (hereafter, ‘operational fuel treatments’) provided the framework for our in situ research examining the impacts of five alternative fuel treatment types (i.e. combinations of thinning, pruning and residue fuel management) on modelled fire behaviour and effects. We addressed two questions: (1) how do key stand attributes vary within operational fuel treatment types? (2) How do alternative fuel treatment types impact potential fire behaviour, represented by modelled potential of passive and active crown fire, and potential fire effects, represented by modelled tree mortality? We measured components of the fuelbed in forest stands before and in the first year after treatments. Because operational implementation varied depending on pre-treatment stand conditions and specific management prescriptions, we examined summary statistics of key stand attributes within fuel treatment types before and after treatment. We then used the field data to model stand-level fire behaviour and effects using the Fire and Fuels Extension to the Forest Vegetation Simulator (FVS-FFE; Reinhardt and Crookston 2003). Using the fire behaviour and effects metrics derived from FVS-FFE, we fitted meta-models and used these to assess the efficacy of the five types of fuel treatments.
Methods
Study sites
We partnered with five community forests in the Kootenay–Boundary Natural Resource Region of BC: Creston, Harrop–Procter, Kaslo and District, Nakusp and Area Community Forests, and Slocan Integral Forestry Cooperative (Fig. 1). These forest tenures range from ~9000 to 33,000 ha, each forming part of the wildland–urban interface of a rural community. These community forests primarily occur in the low-to-mid elevation (i.e. 400–1500 m above sea level) Interior Cedar–Hemlock (ICH) biogeoclimatic ecosystem classification zone, which is characterised by snowy winters and hot dry summers (Ketcheson et al. 1991; Braumandle et al. 1992). Mean temperatures are below 0°C for the coolest winter months and above 15°C for the warmest summer months. Mean annual precipitation ranges from 500 to 1200 mm, with 25–50% as snow (Ketcheson et al. 1991). The forests in the ICH zone have diverse tree species, including western red cedar (Thuja plicata Donn ex D. Don), western hemlock (Tsuga heterophylla (Raf.) Sarg.), western larch (Larix occidentalis Nutt.), interior Douglas-fir (Pseudotsuga menziesii (Mirb.) Franco var. glauca (Beissn.) Franco), ponderosa pine (Pinus ponderosa Douglas ex Lawson), lodgepole pine (Pinus contorta Douglas ex Loudon var. latifolia Engelm.) and paper birch (Betula papyrifera Marshall) (Ketcheson et al. 1991). This seasonally dry mixed-conifer forest type historically experienced mixed-severity fire regimes that have been disrupted by fire exclusion for the past century (Nesbitt 2010; Marcoux et al. 2013, 2015; Greene and Daniels 2017; Chavardès et al. 2022). Contemporary stands in the community forests are dense and continuous. For all five forests, the provincial wildfire threat ratings are high to extreme (British Columbia Wildfire Service 2021), making them a priority for proactive fuel mitigation treatments (British Columbia Community Forest Association 2024).
Study site locations throughout the Kootenay–Boundary Natural Resource Region of British Columbia, Canada. The five community forests are Creston Community Forest (CCF), Harrop–Procter Community Forest (HPCF), Kaslo and District Community Forest (KDCF), Nakusp and Area Community Forest (NACFOR), and Slocan Integral Forestry Cooperative (SIFCo). Basemap attribution: map tiles by Stamen Design, under Creative Commons (CC) BY 3.0 (available at https://creativecommons.org/licenses/by/3.0/ [accessed 29 December 2021]). Data by OpenStreetMap, under ODbL (available at https://www.openstreetmap.org/copyright [accessed 29 December 2021]). Natural Resource Region boundaries attribution: contains information licensed under the Open Government License – British Columbia (available at https://www2.gov.bc.ca/gov/content/data/open-data/open-government-licence-bc [accessed 27 December 2021]). Road attribution: contains information licensed under the Open Government License – Canada (available at https://open.canada.ca/en/open-government-licence-canada [accessed 28 December 2021]). Provincial boundaries attribution: Boundary Files, 2011 Census. Statistics Canada Catalogue no. 92-160-X.
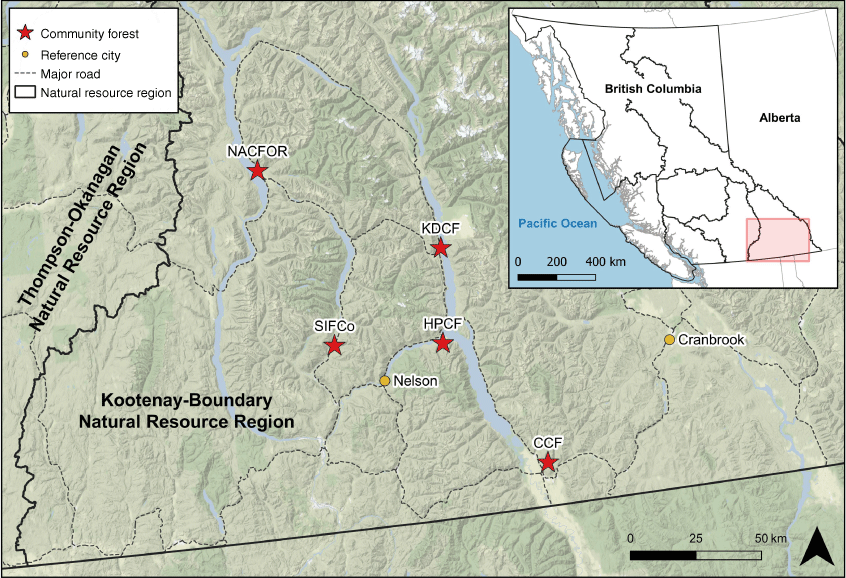
Development of fuel treatment prescriptions in BC
In BC, the guidelines and criteria for fuel treatments set by the BC Wildfire Service are rapidly evolving. When the fuel treatment prescriptions were developed in 2020 and 2021, the community forest managers primarily relied on broad guidance documents from the BC Wildfire Service (British Columbia Wildfire Service 2018, 2020). These guidance documents outlined common principles for fuel reduction treatments, such as reduce surface fuel loads and limit the vertical and horizontal continuity of crown fuels. The BC Wildfire Service provided an optional online worksheet for managers to calculate wildfire intensity (Byram 1959) and critical surface fire intensity for initial crown combustion (Van Wagner 1977; British Columbia Wildfire Service 2024). Outside this provided worksheet, the community forests we collaborated with did not utilise any stand-level fire behaviour models in the development of their fuel treatment prescriptions.
Treatment units and fuel treatment types
Eleven treatment units across the five community forests were used in this study (Table 1). Some treatment units included multiple stands based on tree species composition and planned fuel treatment type, for a total of 26 stands. The fuel treatment prescriptions for all stands were developed by community forest managers using guidance documents provided by BC Wildfire Service (British Columbia Wildfire Service 2018, 2020) and implemented between fall (autumn) 2021 and spring 2022.
Community forest | Treatment unit | Area (ha) | No. stands | No. sampling points per stand | No. sampling points per treatment unit | |
---|---|---|---|---|---|---|
CCF | 1 | 24 | 2 | 2:2 | 4 | |
2 | 24 | 1 | 2 | 2 | ||
3 | 20 | 3 | 1:1:1 | 3 | ||
HPCF | 1 | 26 | 2 | 1:4 | 5 | |
2 | 5 | 2 | 1:2 | 3 | ||
KDCF | 1 | 9 | 2 | 1:2 | 3 | |
2 | 3 | 1 | 2 | 2 | ||
NACFOR | 1 | 41 | 3 | 1:1:3 | 5 | |
2 | 10 | 3 | 1:1:1 | 3 | ||
SIFCo | 1 | 37 | 2 | 3:4 | 7 | |
2 | 18 | 5 | 1:1:1:1:2 | 6 | ||
Totals | 11 | 26 | 43 |
Community forests are Creston (CCF), Harrop–Procter (HPCF), Kaslo and District (KDCF), Nakusp and Area (NACFOR), and Slocan Integral Forestry Cooperative (SIFCo).
We categorised the fuel treatments implemented on the community forests into five different types based on thinning, pruning and residue fuel management (Table 2). Thinning included two levels of thinning from below: low or high intensity (Fig. 2). The post-treatment target tree density varied depending on the starting stand condition and the specific management prescription, but was generally 400–1400 stems ha−1 of well-spaced trees for low-intensity thinning versus 50–350 stems ha−1 for high-intensity thinning. Operational implementation of these thinning levels was achieved via operator execution of guidelines stated in treatment prescriptions rather than via pre-marking of trees for removal or retention. In these prescriptions, trees of any size or species were permitted to be removed for the safety of the workers or the efficiency of the operations. Live and healthy deciduous trees of all sizes were generally retained, as deciduous trees tend to be less flammable than coniferous trees (Pausas et al. 2017), and did not contribute to the target densities. Among coniferous trees, mature, fire-resistant interior Douglas-fir, western larch and ponderosa pine were preferentially retained. For the low-intensity thinning prescriptions, trees were removed by hand falling until a target retained density was achieved. Generally, only non-merchantable coniferous trees with diameter outside bark at breast height (1.3 m above ground; DBH) <17.5 cm considered not suitable for sawn wood products in BC were removed. For the high-intensity thinning prescriptions, tree removals via harvesting machines began with non-merchantable trees and then extended to merchantable trees to reach a target density.
Fuel treatment type | Thinning intensity | Prune | Residue fuel management | No. stands | No. sampling points | |
---|---|---|---|---|---|---|
1 | High | No | Pile burn | 4 | 12 | |
2 | High | Yes | Pile burn | 2 | 4 | |
3 | Low | No | Pile burn | 2 | 3 | |
4 | Low | Yes | Pile burn | 15 | 20 | |
5 | Low | Yes | Chip | 3 | 4 |
Examples of low- and high-intensity thinning from below. Low-intensity thinning example (top row) is from Harrop–Procter Community Forest and high-intensity thinning example (bottom row) is from Slocan Integral Forestry Cooperative. Orange stars represent anchor points for the paired pre- (left column) and post-treatment (right column) photos. All photos in the figure were taken by Kea Rutherford.
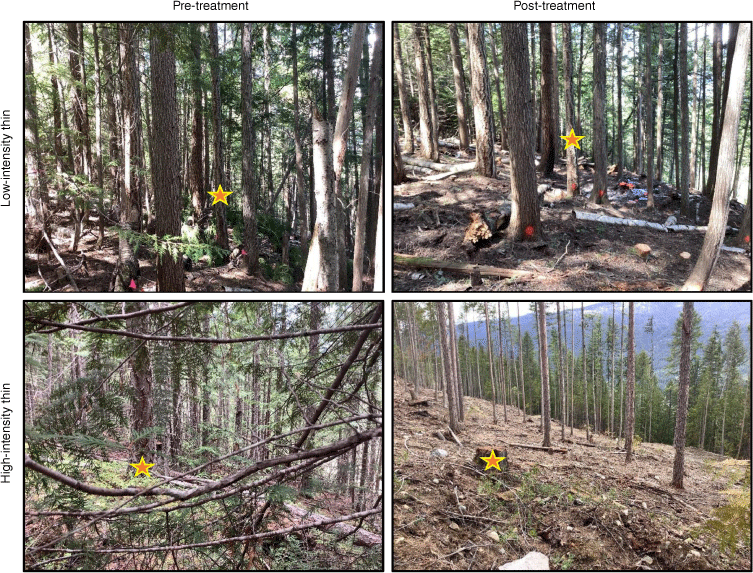
For prescriptions that included pruning, low branches of retained coniferous trees were removed to achieve a live crown base height of 2.5 or 3 m. To avoid compromising small coniferous trees, 40% live crown was retained for these trees rather than pruning to a specific height above ground.
Lastly, residue fuel management included two categories: pile burning or chipping. In the stands where residue fuel was chipped, the wood chips were evenly distributed across the stand to a target depth of 5–10 cm.
Field measurements
We established 43 sampling points across the 26 stands (Table 1). Where community forest personnel had previously established a systematic grid of sampling points, a few of these were randomly selected for measurement. In the other stands, sampling points were selected via simple random sampling.
Field measures were taken before and after treatment in the summers of 2021 and 2022, respectively. At each sampling point, we measured slope, aspect, elevation and key components of the fuelbed. Canopy trees with a DBH ≥12.5 cm were measured within a circular fixed-area plot (Gillis et al. 2005). If tree density was <1000 stems ha−1, we used a 0.04-ha (11.28 m radius) plot; if tree density was ≥1000 stems ha−1, we used a 0.02-ha (7.98 m radius) plot. Similarly, subcanopy trees with a DBH ≥5 cm and <12.5 cm were measured within a smaller subplot. If tree density was <4000 stems ha−1, we used a 0.01-ha (5.64 m radius) plot; if tree density was ≥4000 stems ha−1, we used a 0.005-ha (3.99 m radius) plot. For all standing trees, the species, DBH, total height, live crown base height, dead crown base height, crown position (i.e. progressing downward from dominant trees in the upper canopy through codominant and intermediate trees to suppressed trees in the lower canopy) and status (i.e. live, dead with decay class; USDA Forest Service 2019) were recorded. Surface fuels were measured using the line-intercept method (Brown 1974) along two perpendicular 30-m transects crossed at the mid-point. We tallied 1-h surface fuels (i.e. diameter ≤0.635 cm). For all 10-, 100- and 1000-h surface fuels (i.e. diameter >0.635 cm), we measured the diameter at the point of intersection and recorded decay class and, when possible, species. Surface fuel loads were calculated using published equations (Brown 1974; Beaufait et al. 1975; Ryan and Pickford 1978; US Forest Products Laboratory 1980; Woodall and Monleon 2008). For all 1-h surface fuels and for 10-, 100- and 1000-h surface fuels of unknown species, we weighted the coefficients required to calculate loadings by percentage basal area by species (Stephens 2001). Canopy cover was measured along the two line transects using a tube densitometer (Jennings et al. 1999). We measured the distance along each transect where shrubs intersected the line, which we used to calculate percentage shrub cover (Canfield 1941). A 0.01-ha circular subplot was established at the end of one of the line transects, in which regenerating trees (DBH <5 cm and height >1.3 m) were counted by species and status (i.e. live, dead) and percentage covers of grass and moss were ocularly estimated. The same field measurements were taken at the same sampling points pre- and post-treatment, except the DBH and total height of each retained tree, which we assumed did not change pre- to post-treatment (i.e. only 1 year of growth).
Stand attributes
The fuel treatments prescribed by the community forest managers aimed to reduce or rearrange surface, ladder and crown fuels. For each sampling point, key stand attributes were compiled from the pre- and post-treatment field data (Table 3). Surface fuels were described by fine fuel load. Ladder fuels were described by the density of small live trees, average live crown base height and average dead crown base height. Crown fuels were described by the density of medium plus large live trees and the relative composition of fire-resistant species.
Variable | Description | |
---|---|---|
Stand attributes representing surface, ladder, and crown fuels | ||
Large tree density (stems ha−1) | Live conifer trees with DBH ≥17.5 cm | |
Medium tree density (stems ha−1) | Live conifer trees with DBH 7.5–17.5 cm | |
Small tree density (stems ha−1) | Live conifer trees with DBH <7.5 cm and height >1.3 m | |
Fire-resistant species composition (%) | ((Basal area of live Douglas-fir + western larch + ponderosa pine with DBH ≥7.5 cm)/(total basal area of all live conifer trees with DBH ≥7.5 cm)) × 100 | |
Average live crown base height (m) | For all live conifer trees with DBH ≥7.5 cm | |
Average dead crown base height (m) | For all live conifer trees with DBH ≥7.5 cm | |
Fine fuel load (t ha−1) | 1-h A + 10-h B + 100-h C surface fuels | |
Fire behaviour and effects | ||
Probability of torching (%) | P-Torch; probability of passive crown fire occurrence | |
Crowning index (km h−1) | Wind speed (measured 6.1 m above ground) at which active crown fire would be expected, with higher values indicating lower probability of active crown fire | |
Tree mortality (%) | Probability of mortality at an individual tree level, compiled for each plot |
Modelling stand-level fire behaviour and effects
To assess potential stand-level fire behaviour and effects before and after treatment, we used the BC variant of FVS-FFE (Rebain et al. 2010; ESSA Technologies Ltd. 2013). The FFE for the BC variant of FVS uses the FFE originally developed for the Inland Empire variant, which has a recommended geographic range of northern Idaho, western Montana and eastern Washington, an area directly south of the US–Canada border with very similar forest types to our study sites (Ketcheson et al. 1991). To model potential fire behaviour, FVS-FFE links Rothermel (1972, 1991) surface and crown fire rate of spread models with Van Wagner (1977, 1993) crown fire transition and propagation models (Supplementary Material A: Table S1 summarises component models in FVS-FFE). A limitation of this modelling approach is that it generally underpredicts fire behaviour and effects (Fulé et al. 2001; Hall and Burke 2006; Cruz and Alexander 2010), with underprediction more pronounced for the pre-treatment stands (Foster et al. 2020; Stephens et al. 2023). Nevertheless, it has proved to be an effective approach for evaluating relative differences among fuel treatments in seasonally dry forests of the western US (e.g. Low et al. 2023; Stephens et al. 2023; Radcliffe et al. 2024). A strength of FVS-FFE is that it uses 53 standardised surface fuel models that include a range of fire-carrying fuel types (i.e. grass, grass–shrub, shrub, timber litter, timber–understorey and slash; Anderson 1982, Scott and Burgan 2005). These surface fuel models can represent important changes in the type, loading and compactness of surface fuels resulting from treatments. Therefore, we determined that FVS-FFE was a suitable stand-level model to evaluate the relative impacts of different types of fuel treatments on potential fire behaviour and effects in our study system.
Inputs to FVS-FFE included a tree list (i.e. species, status, DBH, total height, live crown ratio and stems per hectare for each tree), slope, aspect, elevation and weather conditions for each plot. For weather conditions, we matched the closest fire weather station within a 300-m elevation range to each community forest. Using archived weather data from 2007 to 2021 (15 years; British Columbia Wildfire Service 2022), we calculated the 90th percentile of temperature to be consistent with standards for fuel treatments set by the BC Wildfire Service (British Columbia Wildfire Service 2023b). We averaged the wind speeds concurrent with the 90th percentile temperature (Table 4). We set fuel moisture to the pre-defined, variant-specific ‘very dry’ group in FVS-FFE (out of four groups: very dry, dry, moist and wet), which best aligned with high fire risk conditions in our study area.
Community forest | Weather station | Station elevation (m) | Temperature (°C) | Probable maximum 1-min wind speed (km h−1) A | |
---|---|---|---|---|---|
CCF | Goatfell | 1098 | 31 | 10 | |
HPCF and KDCF | Akokli Creek | 821 | 28 | 15 | |
NACFOR | Falls Creek | 790 | 29 | 13 | |
SIFCo | Smallwood | 997 | 29 | 13 |
Community forests are Creston (CCF), Harrop–Procter (HPCF), Kaslo and District (KDCF), Nakusp and Area (NACFOR), and Slocan Integral Forestry Cooperative (SIFCo).
From the 53 standardised surface fuel models built into FVS-FFE, pre-treatment surface fuel models were assigned based on measured shrub, grass and moss cover, plus fine surface fuel loads (Table 5; Vaillant et al. 2009). Post-treatment fuel models depended on treatment type. For high-intensity thinning combined with pile burning (fuel treatment Types 1 and 2), timber litter and slash models were assigned to represent disruption of the understorey and measured generation of residue fuel. For low-intensity thinning combined with pile burning (fuel treatment Types 3 and 4), the post-treatment surface fuel conditions were the same as the pre-treatment conditions. For low-intensity thinning combined with chipping (fuel treatment Type 5), timber litter models were assigned to emulate the compact structure of chipped fuelbeds. To account for inherent uncertainty in assigning discrete surface fuel models, we assigned a ‘low’ and a ‘high’ surface fuel model to each plot, both pre- and post-treatment. The low surface fuel model has a lower relative spread rate and flame length than the paired high surface fuel model (Chiono et al. 2017; Low et al. 2023). The low and high surface fuel models were given equal weights in the FVS-FFE simulations to yield one pre- and one post-treatment outcome per plot.
Fuel treatment type | Plot conditions | Low surface fuel model | High surface fuel model | |
---|---|---|---|---|
Pre-treatment (all types) and Post-treatment Types 3 and 4 | Shrub cover ≥ 50% | TU5–165 B | SH4–144 B | |
Shrub cover < 50% and grass/moss cover ≥ 30% | 2 A | GR4–104 B | ||
Shrub cover < 50% and grass/moss cover < 30% | TU2–162 B | TU4–164 B | ||
Post-treatment Types 1 and 2 | NA | TL5–185 B | SB1–201 B | |
Post-treatment Type 5 | NA | TL2–182 B | TL3–183 B |
NA, not applicable.
Outputs of FVS-FFE include modelled fire behaviour (i.e. probability of torching (P-Torch) and crowning index) and fire effects (i.e. tree mortality) for each plot pre- and post-treatment (Table 3). P-Torch has been shown to reflect changes in the potential of passive crown fire more realistically than the alternative torching index metric because it does not require a calculation of stand-level canopy base height, which can be difficult to accurately represent (Rebain et al. 2010; Stephens et al. 2023). FVS-FFE is a powerful tool for making relative comparisons among fuel treatments (e.g. Low et al. 2023; Stephens et al. 2023); however, absolute values of the FVS-FFE outputs must be interpreted with caution. In particular, values of the crowning index can exceed realistic maximum wind speeds, indicating that the stand is highly resistant to active crown fire (Stephens et al. 2009). Based on historical weather data from the Kootenay region (Government of Canada 2022), we selected a threshold wind speed of 60 km h−1. FVS-FFE outputs above this threshold were reassigned a value of 60 km h−1.
Meta-models of fire behaviour and effects metrics
To examine the impacts of the five fuel treatment types on P-Torch, crowning index and tree mortality metrics derived from FVS-FFE, we fitted linear mixed-effects meta-models (Pinheiro and Bates 2000) using the ‘nlme’ package (Pinheiro et al. 2023) in R (R Core Team 2023). This is a meta-modelling approach because FVS-FFE outputs were used as the response variables (e.g. Fulé et al. 2012; Ahmed et al. 2020). For all three models, the response variable was the change in the fire metric from pre-treatment to post-treatment. Fuel treatment type was included as an explanatory variable in all models. Other explanatory variables included represented starting stand conditions calculated using pre-treatment field measurements, namely: average tree height for P-Torch, density of live trees for crowning index and Curtis Relative Density (RD; diameter and stems per hectare stand density metric; Curtis 1982) for tree mortality. Given the modest number of measured plots resulting in 43 records for fitting the linear mixed-effects models (Table 2), only one starting stand condition variable was chosen for each response variable, selected based on our knowledge of the fire metric. A two-way interaction between the starting stand condition variable (i.e. continuous variable) and fuel treatment type (i.e. class variable) was tested for inclusion in each model. Random intercepts for treatment unit and forest were included in the models. These random treatment and forest effects account for possible spatial correlation among plots from the same treatment unit and community forest.
For each response variable, we selected the best random-effects structure by performing likelihood ratio tests (α = 0.05) using the restricted likelihood and the ‘lmtest’ R package version 0.9.40 (Zeileis and Hothorn 2002). Proceeding with the selected random-effects structure, we fitted a model with fuel treatment type, the starting stand condition variable and the possible interaction. We selected the best fixed-effects structure using manual backward elimination (Kutner et al. 2005), starting with testing whether the interaction term could be dropped. To decide whether a variable was dropped from the model, we performed a likelihood ratio test (α = 0.05) using the full likelihood fit of the model. For each model, we checked that the assumptions of linear models were met by examining residual, normality and fitted line plots.
For models where the interaction with fuel treatment type was not significant, multiple comparisons among treatment means adjusted to the average of the starting stand condition variable were obtained using the ‘emmeans’ package in R (Lenth 2023) for: (1) high- versus low-intensity thinning treatments; (2) presence or absence of pruning within high-intensity thinning treatments; (3) presence or absence of pruning within low-intensity thinning treatments; and (4) pile burning and chipping residue fuel within low-intensity thinning treatments. Significant differences were determined by an α-level of 0.05, adjusted for multiple comparisons using the common Bonferroni P-value adjustment method. Only comparisons relevant for the specific fire metric were evaluated.
Results
Summary statistics of stand attributes within fuel treatment types
As expected, the densities of large-diameter trees substantially decreased following the high-intensity thinning treatments (i.e. fuel treatment Types 1 and 2; Fig. 3a). After treatment, the densities of large trees ranged from 0 to 325 stems ha–1, depending on the pre-treatment stand condition and the specific management prescription. For the low-intensity thinning treatments (i.e. fuel treatment Types 3, 4, and 5), the densities of large trees remained mostly unchanged, as prescribed. The densities of medium- and small-sized trees substantially decreased following treatment for all five treatment types (Fig. 3b, c). For the low-intensity thinning treatments, the density of medium-sized trees ranged from 0 to 700 stems ha–1 post treatment. Variation among sampling points reflected prescriptions that stipulated removals of trees below 12.5, 15 or 17.5 cm DBH, depending on pre-treatment stand conditions.
Measured stand densities stratified by tree size (rows) and fuel treatment type (columns). Tree sizes are (a) large (DBH ≥17.5 cm); (b) medium (DBH 7.5–17.5 cm); and (c) small (DBH <7.5 cm and height >1.3 m). Blue represents pre-treatment and green represents post-treatment values. Within box plots, lines represent median values and stars represent mean values. For each treatment type, n is the number of sampling points.
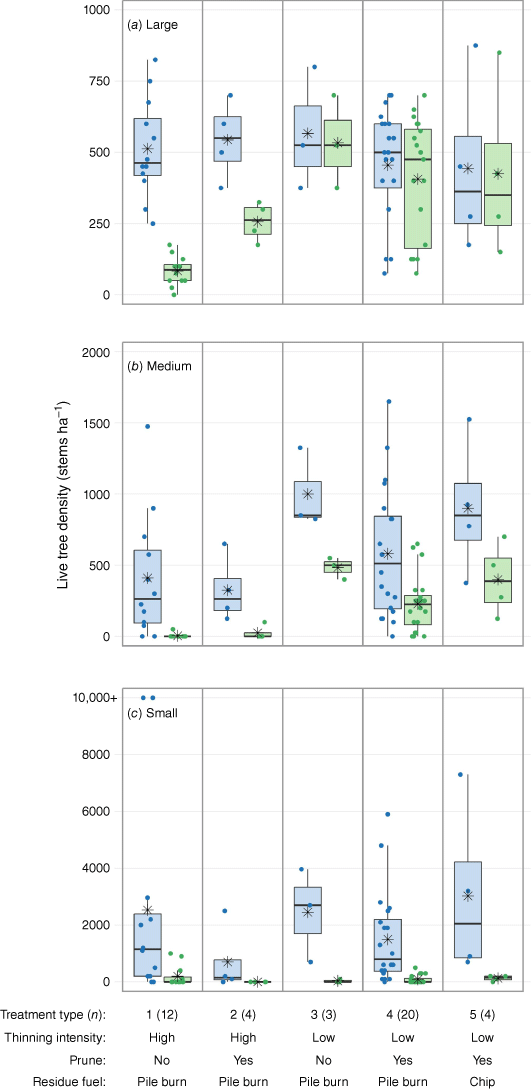
Changes in the relative composition of fire-resistant species reflected pre-treatment stand composition and the type of fuel treatment applied (Fig. 4a). For fuel treatment Type 1 (i.e. high-intensity thinning treatment), operators had a greater opportunity to preferentially select fire-resistant species for retention. Thus, the relative composition of fire-resistant species generally increased following treatment, with much higher variability pre- versus post-treatment. Stands that received fuel treatment Types 2 and 3 had low proportions of fire-resistant species before treatment, providing minimal opportunity to prioritise the retention of fire-resistant species so that the relative composition remained low after treatment. Stands that received fuel treatment Types 4 and 5 (i.e. low-intensity thinning treatments) had high variability in the relative composition of fire-resistant species before and after treatment. For these treatments, trees were selected for removal primarily based on size limits rather than species.
Measured stand attributes (panels a–d), summarised by fuel treatment type (columns within each panel). Blue represents pre-treatment and green represents post-treatment values. Within box plots, lines represent median values and stars represent mean values. For each treatment type, n is the number of sampling points.
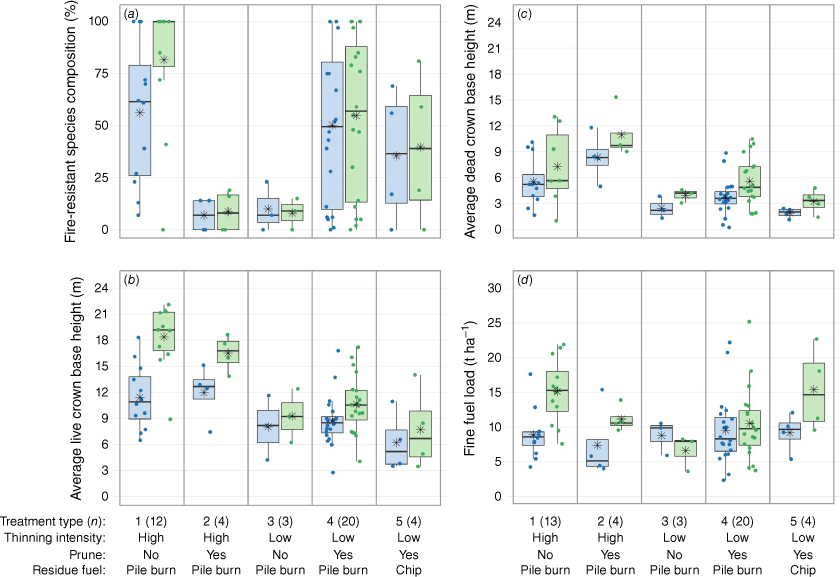
Average live crown base height was highly variable before treatment in all fuel treatment types (Fig. 4b). In stands that received high-intensity thinning treatments, all sampling points had a starting average live crown base height >6 m, well above the 2.5 or 3 m pruning target. Similarly, in stands that received low-intensity thinning treatments, most sampling points had a starting average live crown base height >3 m. Regardless of the presence or absence of pruning, average live crown base height generally increased following treatment owing to the retention of larger trees, which tend to have higher live crown base heights.
Pruning also removed dead branches below the 2.5 or 3 m pruning target. Stands that received high-intensity thinning treatments had high variability in average dead crown base height before treatment (Fig. 4c). For fuel treatment Type 1, variability was greater after treatment. For fuel treatment Type 2, average dead crown base height generally increased but variability was similar after treatment. In stands that received low-intensity thinning treatments, average dead crown base height generally increased following removal of most small trees, but variability remained moderate before and after treatment.
Fine fuel load substantially increased with the high-intensity thinning treatments that removed a greater number of trees using harvesting machines, which generated a large amount of residue fuel as small trees, treetops and branches were scattered on the forest floor (Fig. 4d). The increase of fine residue fuel was reduced through pile burning with varying degrees of success, as demonstrated by the high variability in fine fuel load after treatment, especially within fuel treatment Type 1. For low-intensity thinning treatments combined with pile burning (i.e. fuel treatment Types 3 and 4), the ranges in fine fuel load were similar pre- and post-treatment owing to the removal of residue fuel through pile burning. Fine fuel load generally increased with the low-intensity thinning treatment combined with chipping (i.e. fuel treatment Type 5), as expected.
Differences in modelled fire behaviour and effects among fuel treatment types
For P-Torch, the final meta-model had fuel treatment type and average tree height as fixed effects (Supplementary Material B: Tables S1 and S2). The final meta-model for crowning index had a random treatment unit effect as well as fuel treatment type and density of live trees as fixed effects (Supplementary Material B: Tables S3 and S4). The final meta-model for tree mortality had fuel treatment type and Curtis RD as fixed effects (Supplementary Material B: Tables S5 and S6). The interaction between treatment and the specific starting stand condition variable was not significant in any of the meta-models. Fit statistics for all final meta-models are provided in Supplementary Material C.
P-Torch generally decreased following treatment across all fuel treatment types, indicating lower relative potential for passive crown fire (Fig. 5a). Using the meta-model, the change in P-Torch from pre- to post-treatment was not significantly different between low- and high-intensity thinning treatments (Table 6). Within high-intensity thinning treatments, there was no significant difference in the change of P-Torch between pruning and not pruning. Within low-intensity thinning treatments, neither pruning nor residual fuel management approach had a significant effect on the change in P-Torch.
Fire behaviour and fire effects metrics output from FVS-FFE (panels a–c), summarised by fuel treatment type (columns within each panel). Blue represents pre-treatment and green represents post-treatment values. Within box plots, lines represent median values and stars represent mean values. For each treatment type, n is the number of sampling points.
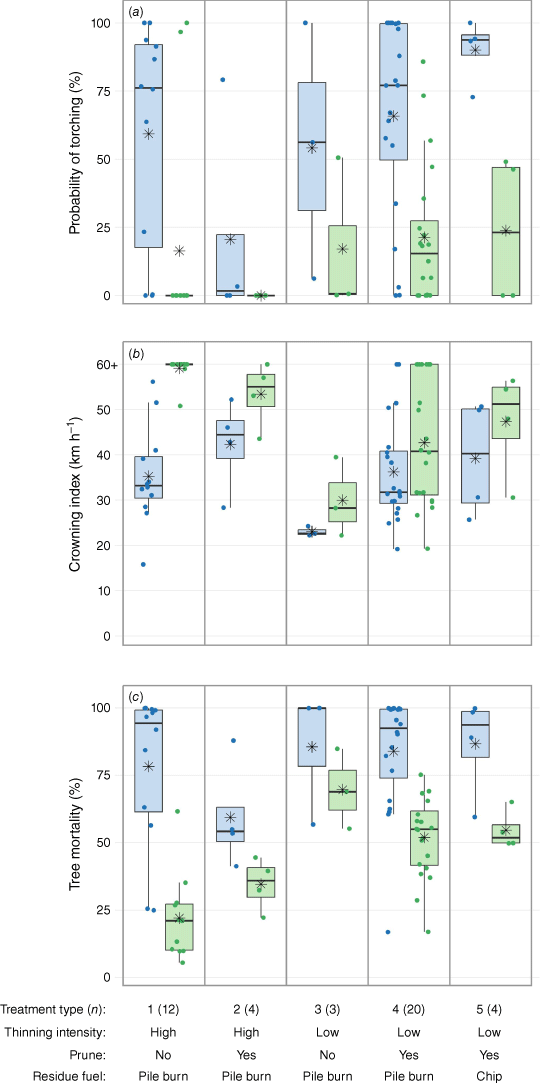
Comparison | Probability of torching (%) | Crowning index (km h−1) | Tree mortality (%) | |
---|---|---|---|---|
(1) Thinning intensity (high – low) | 3 (15)ns | 12 (3)** | −12 (8)* | |
(2) Pruning within high-intensity thinning (no – yes) | −12 (21)ns | – | – | |
(3) Pruning within low-intensity thinning (no – yes) | 20 (22)ns | – | – | |
(4) Residue fuel within low-intensity thinning (pile burn – chip) | 20 (21)ns | – | 10 (12)ns |
Means (s.e.) are for select comparisons: (1) low- versus high-intensity thinning treatments; (2) presence or absence of pruning within high-intensity thinning treatments; (3) presence or absence of pruning within low-intensity thinning treatments; and (4) pile burning and chipping residue fuel within low-intensity thinning treatments. Estimated marginal means were calculated at the average of the starting stand condition variable. Differences in the change of the fire behaviour or effects metric are significant at: **P < 0.01; *P < 0.05; ns, not significant at P = 0.05.
The crowning index generally increased with treatment across all fuel treatment types, representing lower potential of active crown fire (Fig. 5b). The change in the crowning index from pre- to post-treatment was significantly different between low- and high-intensity thinning treatments. On average, the crowning index increased by 12 km h−1 for high-intensity relative to low-intensity thinning (Table 6).
Modelled tree mortality generally decreased following treatment across all fuel treatment types (Fig. 5c). The change in modelled tree mortality from pre- to post-treatment was significantly different between low- and high-intensity thinning treatments. On average, modelled tree mortality decreased by 12% for high-intensity relative to low-intensity thinning (Table 6). Within low-intensity thinning treatments, there was no significant difference in the change of tree mortality between chipping and pile burning.
Discussion
Many of the ICH forests in the Kootenay region of BC have abundant and continuous fuels that pose high wildfire risk. Fuel treatments implemented by community forest managers aim to mitigate this risk through the reduction and rearrangement of forest fuels, with the ultimate goal of reducing the impacts of wildfire on communities and the forests that surround them. In this study, we measured the structure of forest stands before and after fuel treatments, modelled stand-level fire behaviour and effects, and then used meta-models to compare the efficacy (as in Compher 2010) of five operational fuel treatment types.
Modelling stand-level fire behaviour and effects in seasonally dry montane forests
Modelling stand-level fire behaviour and effects proved challenging owing to inherent limitations of existing models. Research conducted in montane, mixed-conifer forests of the western US commonly employs FVS-FFE, which links Rothermel (1972, 1991) surface and crown fire rate of spread models with Van Wagner (1977, 1993) crown fire transition and propagation models. A limitation of this modelling approach is that it generally underpredicts fire behaviour and effects, which Cruz and Alexander (2010) attribute, in part, to inherent underprediction in the Rothermel (1972, 1991) rate of spread models and incompatible linkages between Rothermel’s (1972) surface fire and Van Wagner’s (1977) crown fire initiation equations.
Given these limitations, we considered using the Crown Fire Initiation and Spread (CFIS) system developed by Alexander et al. (2006a, 2006b). However, we identified incongruencies that limited its application for assessing treated montane forests in the ICH zone of southeastern BC. Specifically, CFIS does not include a built-in surface fire rate of spread model, which prevents the assessment of surface fire effects (e.g. mortality). Additionally, CFIS represents surface fuels as three categorical consumption classes (<1, 1–2 and >2 kg m–2; Alexander et al. 2006b). For many forests in BC, the Canadian Forest Fire Behaviour Prediction System (FBP) can be used to estimate surface fuel consumption and select an appropriate class (Beverly et al. 2020; Perrakis et al. 2023). However, the FBP fuel types poorly represent the ICH forests in our study area (Parisien et al. 2013), particularly managed stands in the seasonally dry montane forests of interior BC (Baron et al. 2024). In its current form, CFIS does not represent the nuanced changes in type, loading and compactness when surface fuels are managed through reduction (e.g. pile burning, broadcast burning) and rearrangement (e.g. chipping).
Ultimately, we determined that FVS-FFE was the best currently available stand-level model to evaluate the relative impacts of alternative types of fuel treatments on potential fire behaviour and effects in our study system. We moderated underprediction bias by FVS-FFE to the extent possible through careful surface fuel model selection based on detailed quantitative data on vegetation cover and fine surface fuel loads, an approach that substantially improves fire behaviour predictions relative to those derived using the default surface fuel model selection in FVS-FFE (Collins et al. 2013). We recognise that fire behaviour and effects metrics derived from FVS-FFE are not precise predictions. To avoid overinterpretations, we summarised our field-based measurements of stand attributes and coupled them with meta-models of fire behaviour and effects metrics derived from FVS-FFE to provide insights into the potential relative impacts of the five alternative fuel treatments implemented by community forest managers in our study sites. Below, we discuss key interpretations from the meta-modelling results and identify priorities for future management and research.
Thinning treatments may reduce potential of crown fire and tree mortality
Low- and high-intensity thinning from below are common fuel treatments in the community forests of BC (British Columbia Community Forest Association 2024). In this study, both levels of thinning intensities generally decreased modelled potential of passive crown fire by mitigating dense understories of shade-tolerant western red cedar, western hemlock and interior Douglas-fir through the removal of small- and medium-sized trees. The removal of understorey trees reduces ladder fuels, hindering vertical fire propagation from the surface into the crown (Agee and Skinner 2005; Harrod et al. 2009; Stephens et al. 2009). The high-intensity thinning treatments reduced the potential for active crown fire more than the low-intensity thinning treatments. Removal of some larger trees by high-intensity thinning also disrupts horizontal continuity of crown fuels, making the stand less susceptible to tree-to-tree crown fire spread (Agee and Skinner 2005; Johnson et al. 2011).
The low- and high-intensity thinning treatments were designed to retain larger trees to increase resistance to fire by lowering tree mortality in the advent of wildfire. Modelling outcomes indicated that both types of thinning generally decreased potential tree mortality; however, the high-intensity thinning treatments led to lower potential tree mortality. The high-intensity thinning involved removal of most of the smaller trees before extending into larger trees, favouring the retention of mostly the largest trees in the stand. Larger trees tend to be more fire-resistant, because they are taller and less likely to endure substantial crown scorch (Peterson and Ryan 1986; Agee and Skinner 2005) and have thicker bark that makes them less susceptible to cambial girdling (Ryan and Reinhardt 1988; van Mantgem and Schwartz 2003). Additionally, some of the thinning prescriptions were designed to prioritise the retention of fire-resistant species to increase stand resistance to fire. Interior Douglas-fir, ponderosa pine and western larch tend to have thicker bark and higher crowns than other common conifer species in the ICH zone, making them particularly fire-resistant (Agee 1993; Jain et al. 2012). The substantial increase in relative composition of fire-resistant species for fuel treatment Type 1 (i.e. high-intensity thinning) demonstrates the potential of more intensive thinning to favourably shift the composition of species.
In this study, the community forest managers mostly prescribed low-intensity thinning treatments (20 out of 26 stands), partially in response to public opposition to high-intensity thinning treatments (Copes-Gerbitz et al. 2020; Prichard et al. 2021). However, the modelling results of the present study indicate that high-intensity thinning in the dense stands of the ICH zone represented in this study may reduce passive and active crown fire activity and resultant tree mortality, even during dangerous fire weather conditions (e.g. 90th percentile). When developing fuel treatments, managers must consider how to prevent the initiation of crown fire within a stand, as well as how to reduce the behaviour of an already initiated crown fire spreading from adjacent stands (Scott and Reinhardt 2001). In BC, a specific goal of treatments in the wildland–urban interface is to moderate fire behaviour so that crown fires transition to surface fires, providing greater opportunity for safe and successful suppression tactics (British Columbia Wildfire Service 2020; British Columbia Community Forest Association 2024). The modelling outcomes show that stands that undergo low-intensity thinning may be fairly resistant to the initiation of crown fire, but may not necessarily aid the cessation of crown fire. In contrast, stands that receive high-intensity thinning may be more resistant to the initiation and spread of crown fire. Given that there are often trade-offs between wildfire risk reduction and other values such as wildlife habitat and recreational use (Copes-Gerbitz et al. 2020; Devisscher et al. 2021), high-intensity thinning will not be appropriate for every stand. However, strategically placed high-intensity thinning treatments, intermixed with low-intensity thinning treatments, have the potential to moderate landscape-scale fire behaviour and effects (Hessburg et al. 2015; Tubbesing et al. 2019).
Pruning after thinning may not be an efficient strategy for reducing potential of passive crown fire
Pruning live and dead branches to reduce ladder fuels is a common wildfire management practice in the western US and Canada (Scott and Reinhardt 2007). Although pruning is generally recommended by government agencies (e.g. California Department of Forestry and Fire Protection 2019; British Columbia Wildfire Service 2023b), there is very little research demonstrating the efficacy, or lack thereof, of pruning (York et al. 2022). For our study area, modelling results indicated that pruning after low- or high-intensity thinning treatments did not substantially increase average live or dead crown base height or reduce potential for passive crown fire. Logistically, it is difficult to prune higher than 3 m above ground using hand tools, which limits the potential additive impacts of pruning. Once smaller trees had been removed through thinning, we found little benefit to pruning the larger residual trees, which generally have higher starting crown base heights. Funding for fuel treatments is limited (Copes-Gerbitz et al. 2020) and pruning is often a substantial proportion of total treatment cost (Erik Leslie, Harrop–Procter Community Forest manager, pers. comm.). Further research on pruning as a fuel reduction technique would help forest managers to prioritise finite resources.
Chipping or pile burning of residue fuel mitigates fire behaviour, but the fire effects associated with chipped fuelbeds remain a concern
Thinning and pruning treatments elevate surface fuel loads when small trees, treetops and branches are scattered over the treatment unit (Schwilk et al. 2009). In the community forests, these residue fuels are commonly ameliorated through pile burning or chipping. Pile burning aims to remove residue fuel from the site, whereas chipping rearranges the residue fuel into a more compact structure (Kreye et al. 2014). Community forest managers are increasingly interested in chipping as a residue fuel management approach, because it is cost effective and does not require follow-up burning (Kreye et al. 2014).
Using models, we found no difference in the change of potential for passive crown fire between pile burning and chipping of residue fuel. As expected, chipping led to a substantial increase in fine fuel load; however, abundant fine fuels were countered by the shallow and compact structure of the chipped fuelbeds. When compact fuelbeds burn, they tend to exhibit low surface fire intensity and flame length, decreasing the potential of crown fire initiation (Glitzenstein et al. 2006; Kreye and Kobziar 2015; Moore et al. 2020).
Community forest managers are additionally concerned with the potential fire effects of alternative residue fuel management approaches. Our modelling results indicated that there was no difference in the change in tree mortality between fuelbeds generated by pile burning or chipping. However, FVS-FFE only models the fire behaviour and effects of the initial flaming front and does not capture potential impacts of long-duration heating (Rebain et al. 2010). In compact fuelbeds, the flaming front is expected to have low intensity and flame length, which has been shown to reduce crown scorch and resultant tree morality (Jerman et al. 2004). However, chipped fuelbeds often experience long-term residual flaming and smouldering, which can cause delayed tree mortality from cambial or root injury (Busse et al. 2005; Knapp et al. 2011; Kreye et al. 2014). Given that FVS-FFE does not capture potential delay, the modelled tree mortality may have been underpredicted for chipped fuelbeds. Further research on the fire effects of chipping would help forest managers better weigh trade-offs between wildfire resistance and treatment cost.
Post-treatment surface fuel load targets were difficult to achieve
Reducing surface fuel load is a common core principle of fuel treatments (Agee and Skinner 2005). A reduction in surface fuel load can lower surface fire intensity and flame length, reducing the chance of crown fire initiation (Van Wagner 1977; Prichard et al. 2010; Stephens et al. 2012). Furthermore, lower fire intensity can prevent trees from being killed by crown scorch or cambium injury (Van Wagner 1973; Ryan and Reinhardt 1988). Following this principle, many of the prescriptions prepared by the community forest managers aimed to reduce fine surface fuel load to below pre-treatment levels. However, our post-treatment field measurements show that fine surface fuel load increased substantially following higher intensity thinning combined with pile burning, demonstrating the difficulty of reducing surface fuel loads due to the influx of fine residue fuel generated by thinning and pruning. In contrast, for the lower-intensity thinning treatments combined with pile burning, fine surface fuel load was similar pre- and post-treatment. Combined, these findings provide important feedback for managers, as they are not always achieving their post-treatment surface fuel load targets.
Conclusion
This research contributes to understanding fuel treatment efficacy (as in Compher 2010) in seasonally dry montane forests of interior BC, where wildfires have had tremendous ecological and social impacts over the past 20 years (Daniels et al. 2024). By combining field measurements, fire behaviour modelling and meta-modelling, we were able to draw three key insights to help guide future fuel treatments and research in dense ICH forests of our study area: (1) high-intensity thinning treatments may be needed to reduce potential of active crown fire; (2) pruning after thinning from below may not further reduce potential of passive crown fire; and (3) further research is needed on chipping as a residue fuel management approach. By permanently marking our study plots, we provide opportunity for future research to evaluate changes in fuel loads through time and to compare observed fire behaviour and effects with model predictions, if wildfires intercept our study plots. We look forward to future collaborations with community forest managers to enhance assessments of fuel treatment efficacy and effectiveness in the montane forests of BC.
Data availability
The data that support this study will be shared on reasonable request addressed to the corresponding author.
Declaration of funding
This work was funded by the British Columbia Ministry of Forests, Lands, Natural Resource Operations and Rural Development and the Natural Sciences and Engineering Research Council (NSERC)—Canada Wildfire Network Grant.
Acknowledgements
As researchers at the University of British Columbia, Vancouver, we work on the traditional, ancestral, and unceded territory of the xʷməθkʷəy̓əm (Musqueam) people. As researchers in the Kootenay region of British Columbia, we work on the traditional, ancestral and unceded territory of the Ktunaxa ʔamakʔis (Ktunaxa), Secwepemcúl’ecw (Secwépemc), Syilx (Okanagan), and snʕickstx tmxʷúlaʔxʷ (Sinixt) people. We thank Daniel Gratton from Creston Community Forest, Erik Leslie from Harrop–Procter Community Forest, Jeff Reyden from Kaslo and District Community Forest, Frances Swan, Erin McLeod and Kathleen Ennis from Nakusp and Area Community Forest, and Stephan Martineau, Tom Bradley and Jesper Nielsen from Slocan Integral Forestry Cooperative for collaborating with the University of British Columbia Tree Ring Lab. Thank you to Jennifer Gunter and Susan Mulkey from the British Columbia Community Forest Association for facilitating this collaborative effort. We thank the editor-in-chief, associate editor and two anonymous reviewers for comments to improve the manuscript.
References
Agee JK, Skinner CN (2005) Basic principles of forest fuel reduction treatments. Forest Ecology and Management 211, 83-96.
| Crossref | Google Scholar |
Ahmed S, LeMay V, Yanchuk A, Robinson A, Marshall P, Bull G (2020) Meta-modelling to quantify yields of white spruce and hybrid spruce provenances in the Canadian boreal forest. Forests 11(6), 609.
| Crossref | Google Scholar |
Alexander ME, Cruz MG, Lopes AMG (2006a) CFIS: a software tool for simulating crown fire initiation and spread. Forest Ecology and Management 234, S133.
| Crossref | Google Scholar |
Baron JN, Hessburg PF, Parisien MP, Greene GA, Gergel SE, Daniels LD (2024) Fuel types misrepresent forest structure and composition in interior British Columbia: a way forward. Fire Ecology 20, 15.
| Crossref | Google Scholar | PubMed |
Beverly JL, Leverkus SER, Cameron H, Schroeder D (2020) Stand-level fuel reduction treatments and fire behaviour in Canadian boreal conifer forests. Fire 3, 35.
| Crossref | Google Scholar |
Braumandl TF, Dykstra PR, Davis G, DeLong D, Fenger M, Ketcheson M, Norris D, Peschke B, Quesnel H, Steeger C, Stewart R, Woods G (1992) A field guide for site identification and interpretation for the Nelson Forest Region. Land Management Handbook 20. (Eds TF Braumandl, MP Curran) (BC Ministry of Forests: Victoria, BC, Canada) Available at https://www.for.gov.bc.ca/hfd/pubs/Docs/Lmh/Lmh20.htm [accessed 1 May 2023]
British Columbia Community Forest Association (2024) Enhancing wildfire resilience: summary report of the BCCFA’s wildfire risk reduction economic recovery initiative. Available at https://bccfa.ca/wp-content/uploads/2024/06/BCCFA-Enhancing-Wildfire-Resilience-Report.pdf [accessed 26 August 2024]
British Columbia Wildfire Service (2018) BC Wildfire Service wildfire risk reduction planning standard 2018. Available at https://www2.gov.bc.ca/assets/gov/public-safety-and-emergency-services/wildfire-status/prevention/fire-fuel-management/wildfire_risk_reduction_planning_standard.pdf [accessed 26 August 2024]
British Columbia Wildfire Service (2020) 2020 fuel management prescription guidance. Available at https://www2.gov.bc.ca/assets/gov/public-safety-and-emergency-services/wildfire-status/prevention/fire-fuel-management/fuels-management/2020_fuel_management_prescription_guidance_final.pdf [accessed 26 August 2024]
British Columbia Wildfire Service (2021) 2021 Provincial Strategic Threat Analysis. (BC Ministry of Forests, Lands, Natural Resource Operations and Rural Development: Victoria, BC, Canada) Available at https://www2.gov.bc.ca/gov/content/safety/wildfire-status/prevention/fire-fuel-management/psta [accessed 26 August 2024]
British Columbia Wildfire Service (2022) Fire weather. Available at https://alpha.gov.bc.ca/gov/content/safety/wildfire-status/prepare/weather-fire-danger/fire-weather [accessed 27 November 2022]
British Columbia Wildfire Service (2023a) Wildfire season summary. Available at https://www2.gov.bc.ca/gov/content/safety/wildfire-status/about-bcws/wildfire-history/wildfire-season-summary [accessed 26 May 2024]
British Columbia Wildfire Service (2023b) 2023 fuel management practices guide: forest fuel management planning, treatment design and implementation methodologies. Available at https://www2.gov.bc.ca/gov/content/safety/wildfire-status/prevention/fire-fuel-management/fuel-management [accessed 18 February 2024]
British Columbia Wildfire Service (2024) Tools for fuel management: 90th percentile Fire Weather Index conditions and tools used for analysis. Available at https://www2.gov.bc.ca/gov/content/safety/wildfire-status/prevention/fire-fuel-management/fuel-management [accessed 26 August 2024]
Busse MD, Hubbert KR, Fiddler GO, Shestak CJ, Powers RF (2005) Lethal soil temperatures during burning of masticated forest residues. International Journal of Wildland Fire 14(3), 267-276.
| Crossref | Google Scholar |
California Department of Forestry and Fire Protection (2019) Board of Forestry Technical Rule Addendum No. 4: illustration of removal of ladder fuels and horizontal continuity of fuels. In ‘California Forest Practice Rules 2023’. p. 202. Available at https://bof.fire.ca.gov/media/hffh3kdv/post-last-week-of-dec-2023-forest-practice-rules-and-act-final.pdf [accessed 15 August 2023]
Canfield R (1941) Application of line intercept method in sampling range vegetation. Journal of Forestry 39, 388-394.
| Google Scholar |
Chavardès RD, Daniels LD, Harvey JE, Greene GA, Marcoux H, Eskelson BNI, Gedalof Z, Brookes W, Kubian R, Cochrane JD, Nesbitt JH, Pogue AM, Villemaire-Côté O, Gray RW, Andison DW (2022) Regional drought synchronized historical fires in dry forests of the Montane Cordillera Ecozone, Canada. International Journal of Wildland Fire 31(1), 67-80.
| Crossref | Google Scholar |
Chiono LA, Fry DL, Collins BM, Chatfield AH, Stephens SL (2017) Landscape-scale fuel treatment and wildfire impacts on carbon stocks and fire hazard in California spotted owl habitat. Ecosphere 8(1), e01648.
| Crossref | Google Scholar |
Collins BM, Kramer HA, Menning K, Dillingham C, Saah D, Stine PA, Stephens SL (2013) Modeling hazardous fire potential within a completed fuel treatments network in the northern Sierra Nevada. Forest Ecology and Management 310, 156-166.
| Crossref | Google Scholar |
Compher C (2010) Efficacy vs effectiveness. Journal of Parenteral and Enteral Nutrition 34(6), 598-599.
| Crossref | Google Scholar | PubMed |
Coogan SCP, Daniels LD, Boychuk D, Burton PJ, Flannigan MD, Gauthier S, Kafka V, Park JS, Wotton BM (2021) Fifty years of wildland fire science in Canada. Canadian Journal of Forest Research 51(2), 283-302.
| Crossref | Google Scholar |
Copes-Gerbitz K, Dickson-Hoyle S, Hagerman SM, Daniels LD (2020) BC community forest perspectives and engagement in wildfire management. Report to the Union of BC Municipalities, First Nations’ Emergency Services Society, BC Community Forest Association and BC Wildfire Service. 49 pp. Available at https://www.ubctreeringlab.ca/post/wildfire-management-in-bc-community-forests-2020
Crosby JS, Chandler CC (1966) Get the most out of your windspeed observation. Fire Control Notes 27, 12-13.
| Google Scholar |
Cruz MG, Alexander ME (2010) Assessing crown fire potential in coniferous forests of western North America: a critique of current approaches and recent simulation studies. International Journal of Wildland Fire 19(4), 377-398.
| Crossref | Google Scholar |
Curtis RO (1982) A simple index of stand density for Douglas-fir. Forest Science 28(1), 92-94.
| Google Scholar |
Daniels LD, Gray RW, Burton, PJ (2020) 2017 Megafires in British Columbia – Urgent need to adapt and improve resilience to wildfire. In ‘The fire continuum – preparing for the future of wildland fire. Proceedings of the Fire Continuum Conference’, 21–24 May 2018, Missoula, MT. (Eds S Hood, S Drury, T Steelman, R. Steffens) pp. 51‒62. (USDA Forest Service, Rocky Mountain Research Station: Fort Collins, CO)
Daniels LD, Dickson-Hoyle S, Baron JN, Copes-Gerbitz K, Flannigan MD, Castellanos Acuna D, Hoffman KM, Bourbonnais M, Wilkinson SL, Roeser D, Harvey JE, Laflamme J, Tiribelli F, Whitehead J, Leverkus SER, Gray RW (2024) The 2023 wildfires in British Columbia, Canada: impacts, drivers, and transformations to coexist with wildfire. Canadian Journal of Forest Research e-First.
| Crossref | Google Scholar |
Davis KT, Peeler J, Fargione J, Haugo RD, Metlen KL, Robles MD, Woolley T (2024) Tamm review: a meta-analysis of thinning, prescribed fire, and wildfire effects on subsequent wildfire severity in conifer dominated forests of the Western US. Forest Ecology and Management 561, 121885.
| Crossref | Google Scholar |
Devisscher T, Spies J, Griess V (2021) Time for change: learning from community forests to enhance the resilience of multi-value forestry in British Columbia, Canada. Land Use Policy 103, 105317.
| Crossref | Google Scholar |
Dickson-Hoyle S, Copes-Gerbitz K, Hagerman SM, Daniels LD (2023) Community Forests advance local wildfire governance and proactive management in British Columbia, Canada. Canadian Journal of Forest Research 2, 290-304.
| Google Scholar |
Forest Practices Board (2023) Forest and fire management in BC: toward landscape resilience. Special Report 61. (Victoria, BC, Canada) Available at https://www.bcfpb.ca/wp-content/uploads/2023/06/SR61-Landscape-Fire-Management.pdf
Foster DE, Battles JJ, Collins BM, York RA, Stephens SL (2020) Potential wildfire and carbon stability in frequent-fire forests in the Sierra Nevada: trade-offs from a long-term study. Ecosphere 11(8), e03198.
| Crossref | Google Scholar |
Fulé PZ, McHugh C, Heinlein TA, Covington WW (2001) Potential fire behavior is reduced following forest restoration treatments. In ‘Ponderosa pine ecosystems restoration and conversation: steps toward stewardship’. Rocky Mountain Research Station Proceedings RMRS-P-22. (Eds RK Vance, CB Edminster, WW Covington, TA Blake) pp. 28–35. (USDA Forest Service: Ogden, UT)
Fulé PZ, Crouse JE, Roccaforte JP, Kalies EL (2012) Do thinning and/or burning treatments in western USA ponderosa or Jeffrey pine-dominated forests help restore natural fire behavior? Forest Ecology and Management 269, 68-81.
| Crossref | Google Scholar |
Gillis MD, Omule AY, Brierley T (2005) Monitoring Canada’s forests: the national forest inventory. Forestry Chronicle 81(2), 214-221.
| Crossref | Google Scholar |
Glitzenstein JS, Streng DR, Achtemeier GL, Naeher LP, Wade DD (2006) Fuels and fire behavior in chipped and unchipped plots: implications for land management near the wildland/urban interface. Forest Ecology and Management 236, 18-29.
| Crossref | Google Scholar |
Government of Canada (2022) Past weather and climate: historical data. Available at https://climate.weather.gc.ca/historical_data/search_historic_data_e.html [accessed 1 October 2022]
Gray RW, Blackwell BA (2008) Fuel management strategies in 60-year-old Douglas-fir/ponderosa pine stands in the Squamish Forest district, British Columbia. In ‘Proceedings of the 2002 fire conference: Managing fire and fuels in the remaining wildlands and open spaces of the Southwestern United States’. General Technical Report PSW-GTR-189. (Ed. MG Narog) pp. 57–64. (USDA Forest Service, Pacific Southwest Research Station: Albany, CA)
Greene GA, Daniels LD (2017) Spatial interpolation and mean fire interval analyses quantify historical mixed-severity fire regimes. International Journal of Wildland Fire 26(2), 136-147.
| Crossref | Google Scholar |
Hagmann RK, Hessburg PF, Prichard SJ, Povak NA, Brown PM, Fulé PZ, Keane RE, Knapp EE, Lydersen JM, Metlen KL, Reilly MJ, Sánchez Meador AJ, Stephens SL, Stevens JT, Taylor AH, Yocom LL, Battaglia MA, Churchill DJ, Daniels LD, Falk DA, Henson P, Johnston JD, Krawchuk MA, Levine CR, Meigs GW, Merschel AG, North MP, Safford HD, Swetnam TW, Waltz AEM (2021) Evidence for widespread changes in the structure, composition, and fire regimes of western North American forests. Ecological Applications 31(8), e02431.
| Crossref | Google Scholar | PubMed |
Hall SA, Burke IC (2006) Considerations for characterizing fuels as inputs for fire behavior models. Forest Ecology and Management 227, 102-114.
| Crossref | Google Scholar |
Harrod RJ, Peterson DW, Povak NA, Dodson EK (2009) Thinning and prescribed fire effects on overstory tree and snag structure in dry coniferous forests of the interior Pacific Northwest. Forest Ecology and Management 258(5), 712-721.
| Crossref | Google Scholar |
Hessburg PF, Agee JK, Franklin JF (2005) Dry forests and wildland fires of the inland Northwest USA: contrasting the landscape ecology of the pre-settlement and modern eras. Forest Ecology and Management 211(1–2), 117-139.
| Crossref | Google Scholar |
Hessburg PF, Churchill DJ, Larson AJ, Haugo RD, Miller C, Spies TA, North MP, Povak NA, Belote RT, Singleton PH, Gaines WL, Keane RE, Aplet GH, Stephens SL, Morgan P, Bisson PA, Rieman BE, Salter RB, Reeves GH (2015) Restoring fire-prone Inland Pacific landscapes: seven core principles. Landscape Ecology 30, 1805-1835.
| Crossref | Google Scholar |
Hessburg PF, Miller CL, Parks SA, Povak NA, Taylor AH, Higuera PE, Prichard SJ, North MP, Collins BM, Hurteau MD, Larson AJ, Allen CD, Stephens SL, Rivera-Huerta H, Stevens-Rumann CS, Daniels LD, Gedalof Z, Gray RW, Kane VR, Churchill DJ, Hagmann RK, Spies TA, Cansler CA, Belote RT, Veblen TT, Battaglia MA, Hoffman C, Skinner CN, Safford HD, Salter RB (2019) Climate, environment, and disturbance history govern resilience of western North American forests. Frontiers in Ecology and Evolution 7, 11770.
| Crossref | Google Scholar |
Hessburg PF, Prichard SJ, Hagmann RK, Povak NA, Lake FK (2021) Wildfire and climate change adaptation of western North American forests: a case for intentional management. Ecological Applications 31(8), e02432.
| Crossref | Google Scholar | PubMed |
Jain TB, Battaglia MA, Han H, Graham RT, Keyes CR, Fried JS, Sandquist JE (2012) A comprehensive guide to fuel management practices for dry mixed conifer forests in the Northwestern United States. General Technical Report RMRS-GTR-292. (USDA Forest Service, Rocky Mountain Research Station: Fort Collins, CO)
Jain P, Barber QE, Taylor SW, Whitman E, Castellanos Acuna D, Boulanger Y, Chavardès RD, Chen J, Englefield P, Flannigan M, Girardin MP, Hanes CC, Little J, Morrison K, Skakun RS, Thompson DK, Wang X, Parisien MA (2024) Drivers and impacts of the record-breaking 2023 wildfire season in Canada. Nature Communications 15, 6764.
| Crossref | Google Scholar | PubMed |
Jennings SB, Brown ND, Sheil D (1999) Assessing forest canopies and understory illumination: canopy closure, canopy cover, and other measures. Forestry 72(1), 59-73.
| Crossref | Google Scholar |
Jerman JL, Gould PJ, Fulé PZ (2004) Slash compression treatment reduced tree mortality from prescribed fire in southwestern ponderosa pine. Western Journal of Applied Forestry 19(3), 149-153.
| Crossref | Google Scholar |
Johnson MC, Kennedy MC, Peterson DL (2011) Simulating fuel treatment effects in dry forests of the western United States: testing the principles of a fire-safe forest. Canadian Journal of Forest Research 41(5), 1018-1030.
| Crossref | Google Scholar |
Kalies EL, Yocom Kent LL (2016) Tamm review: are fuel treatments effective at achieving ecological and social objectives? A systematic review. Forest Ecology and Management 375, 84-95.
| Crossref | Google Scholar |
Ketcheson MV, Braumandl TF, Meidinger D, Utzig G, Demarchi DA, Wikeem BM (1991) Interior Cedar — Hemlock Zone. In ‘Ecosystems of British Columbia’. Special Report Series No. 6. (Eds D Meidinger, J Pojar) pp. 167–181. (BC Ministry of Forests: Victoria, BC, Canada) Available at https://www.for.gov.bc.ca/hfd/pubs/Docs/Srs/Srs06.htm [accessed 1 May 2023]
Knapp EE, Varner JM, Busse MD, Skinner CN, Shestak CJ (2011) Behaviour and effects of prescribed fire in masticated fuelbeds. International Journal of Wildland Fire 20(8), 932-945.
| Crossref | Google Scholar |
Kreye JK, Brewer NW, Morgan P, Varner JM, Smith AMS, Hoffman CM, Ottmar RD (2014) Fire behavior in masticated fuels: a review. Forest Ecology and Management 314, 193-207.
| Crossref | Google Scholar |
Kreye JK, Kobziar LN (2015) The effect of mastication on surface fire behaviour, fuels consumption and tree mortality in pine flatwoods of Florida, USA. International Journal of Wildland Fire 24(4), 573-579.
| Crossref | Google Scholar |
Lenth RV (2023) emmeans: estimated marginal means, aka least-squares means. R package version 1.8.7. Available at https://CRAN.R-project.org/package=emmeans [accessed 20 July 2023]
Low KE, Battles JJ, Tompkins RE, Dillingham CP, Stephens SS, Collins BM (2023) Shaded fuel breaks create wildfire-resilient forest stands: lessons from a long-term study in the Sierra Nevada. Fire Ecology 19, 29.
| Crossref | Google Scholar |
Marcoux HM, Gergel SE, Daniels LD (2013) Mixed-severity fire regimes: how well are they represented by existing fire-regime classification systems? Canadian Journal of Forest Research 43(7), 658-668.
| Crossref | Google Scholar |
Marcoux HM, Daniels LD, Gergel SE, Da Silva E, Gedalof Z, Hessburg PF (2015) Differentiating mixed- and high-severity fire regimes in mixed-conifer forests of the Canadian Cordillera. Forest Ecology and Management 341, 45-58.
| Crossref | Google Scholar |
Moore B, Thompson DK, Schroeder D, Johnston JM, Hvenegaard S (2020) Using infrared imagery to assess fire behaviour in a mulched fuel bed in black spruce forests. Fire 3(3), 37.
| Crossref | Google Scholar |
Parisien MA, Walker GR, Little JM, Simpson BN, Wang X, Perrakis DDB (2013) Considerations for modeling burn probability across landscapes with steep environmental gradients: an example from the Columbia Mountains, Canada. Natural Hazards 66, 439-462.
| Crossref | Google Scholar |
Parisien MA, Barber Q, Bourbonnais M, Daniels L, Hoffman K, Gray R, Jain J, Taylor S, Whitman E, Flannigan M (2023) Abrupt, climate-induced increase in wildfires in British Columbia since the mid-2000s. Communications Earth & Environment 4, 309.
| Crossref | Google Scholar |
Parks SA, Abatzoglou JT (2020) Warmer and drier fire seasons contribute to increases in area burned at high severity in western US forests from 1985 to 2017. Geophysical Research Letters 47(22), e2020GL089858.
| Crossref | Google Scholar |
Pausas JG, Keeley JE, Schwilk DW (2017) Flammability as an ecological and evolutionary driver. Journal of Ecology 105(2), 289-297.
| Crossref | Google Scholar |
Perrakis DB, Cruz MG, Alexander ME, Hanes CC, Thompson DK, Taylor SW, Stocks BJ (2023) Improved logistic models of crown fire probability in Canadian conifer forests. International Journal of Wildland Fire 32(10), 1455-1473.
| Crossref | Google Scholar |
Peterson DL, Ryan KC (1986) Modeling post-fire conifer mortality for long-range planning. Environmental Management 10(6), 797-808.
| Crossref | Google Scholar |
Pinheiro J, Bates D R Core Team (2023) nlme: linear and nonlinear mixed effects models. R package version 3.1-162. Available at https://CRAN.R-project.org/package=nlme [accessed 20 July 2023]
Prichard SJ, Peterson DL, Jacobson K (2010) Fuel treatments reduce the severity of wildfire effects in dry mixed conifer forest, Washington, USA. Canadian Journal of Forest Research 40(8), 1615-1626.
| Crossref | Google Scholar |
Prichard SJ, Hessburg PF, Hagmann RK, Povak NA, Dobrowski SZ, Hurteau MD, Kane VR, Keane RE, Kobziar LN (2021) Adapting western North American forests to climate change and wildfires: 10 common questions. Ecological Applications 31(8), e02433.
| Crossref | Google Scholar | PubMed |
Radcliffe DC, Bakker JD, Churchill DJ, Alvardo EC, Peterson DW, Laughlin MM, Harvey BJ (2024) How are long-term stand structure, fuel profiles, and potential fire behavior affected by fuel treatment type and intensity in Interior Pacific Northwest forests? Forest Ecology and Management 553, 121594.
| Google Scholar |
R Core Team (2023) R: A language and environment for statistical computing. (R Foundation for Statistical Computing: Vienna, Austria) Available at https://www.r-project.org/ [accessed 20 July 2023]
Ryan KC, Reinhardt ED (1988) Predicting postfire mortality of seven western conifers. Canadian Journal of Forest Research 18(10), 1291-1297.
| Crossref | Google Scholar |
Safford HD, Schmidt DA, Carlson CH (2009) Effects of fuel treatments on fire severity in an area of wildland–urban interface, Angora Fire, Lake Tahoe Basin, California. Forest Ecology and Management 258(5), 773-787.
| Crossref | Google Scholar |
Schwilk DW, Keeley JE, Knapp EE, Mciver J, Bailey JD, Fettig CJ, Fiedler CE, Harrod RJ, Moghaddas JJ, Outcalt KW, Skinner CN, Stephens SL, Waldrop TA, Yaussy DA, Youngblood A (2009) The national Fire and Fire Surrogate study: effects of fuel reduction methods on forest vegetation structure and fuels. Ecological Applications 19(2), 285-304.
| Crossref | Google Scholar | PubMed |
Scott JH, Reinhardt ED (2007) Effects of alternative treatments on canopy fuel characteristics in five conifer stands. In ‘Restoring fire-adapted ecosystems: proceedings of the 2005 national silviculture workshop’. General Technical Report PSW-GTR-203. (Ed. RF Powers) pp. 193–209. (USDA Forest Service, Pacific Southwest Research Station: Albany, CA)
Stephens SL (2001) Fire history differences in adjacent Jeffrey pine and upper montane forests in the eastern Sierra Nevada. International Journal of Wildland Fire 10(2), 161-167.
| Crossref | Google Scholar |
Stephens SL, Moghaddas JJ, Edminster C, Fiedler CE, Haase S, Harrington M, Keeley JE, Knapp EE, Mciver JD, Metlen K, Skinner CN, Youngblood A (2009) Fire treatment effects on vegetation structure, fuels, and potential fire severity in western US forests. Ecological Applications 19(2), 305-320.
| Crossref | Google Scholar | PubMed |
Stephens SL, Collins BM, Roller G (2012) Fuel treatment longevity in a Sierra Nevada mixed conifer forest. Forest Ecology and Management 285, 204-212.
| Crossref | Google Scholar |
Stephens SL, Foster DE, Battles JJ, Bernal AA, Collins BM, Hedges R, Moghaddas JJ, Roughton AT, York RA (2023) Forest restoration and fuels reduction work: different pathways for achieving success in the Sierra Nevada. Ecological Applications 34, e2932.
| Google Scholar |
Thompson DK, Schroeder D, Wilkinson SL, Barber Q, Baxter G, Cameron H, Hsieh R, Marshall G, Moore B, Refai R, Rodell C, Schiks T, Verkaik GJ, Zerb J (2020) Recent crown thinning in a boreal black spruce forest does not reduce spread rate nor total fuel consumption: results from an experimental crown fire in Alberta, Canada. Fire 3(3), 28.
| Crossref | Google Scholar |
Tubbesing CL, Fry DL, Roller GB, Collins BM, Fedorova VA, Stephens SL, Battles JJ (2019) Strategically placed landscape fuel treatments decrease fire severity and promote recovery in the northern Sierra Nevada. Forest Ecology and Management 436, 45-55.
| Crossref | Google Scholar |
USDA Forest Service (2019) Forest Inventory and Analysis National Core Field Guide. Volume I: field data collection procedures for phase 2 plots version 9.0. Available at https://www.fia.fs.usda.gov/library/field-guides-methods-proc/
Vaillant NM, Fites-Kaufman JA, Stephens SL (2009) Effectiveness of prescribed fire as a fuel treatment in Californian coniferous forests. International Journal of Wildland Fire 18(2), 165-175.
| Crossref | Google Scholar |
Van Mantgem P, Schwartz M (2003) Bark heat resistance of small trees in Californian mixed conifer forests: testing some model assumptions. Forest Ecology and Management 178(3), 341-352.
| Crossref | Google Scholar |
Van Wagner CE (1973) Height of crown scorch in forest fires. Canadian Journal of Forest Research 3, 373-378.
| Crossref | Google Scholar |
Van Wagner CE (1977) Conditions for the start and spread of crown fire. Canadian Journal of Forest Research 7, 23-34.
| Crossref | Google Scholar |
Van Wagner CE (1993) Prediction of crown fire behavior in two stands of jack pine. Canadian Journal of Forest Research 23, 442-449.
| Crossref | Google Scholar |
York RA, Russell KW, Noble H (2022) Merging prescribed fires and timber harvests in the Sierra Nevada: burn season and pruning influences in young mixed conifer stands. Trees, Forests and People 9, 100309.
| Crossref | Google Scholar |
Zeileis A, Hothorn T (2002) Diagnostic checking in regression relationships. R News 2(3), 7-10.
| Google Scholar |