A framework for defining fire danger to support fire management operations in Australia†
Jennifer J. Hollis A E * , Stuart Matthews A F , Wendy R. Anderson C , Miguel G. Cruz

A
B
C
D
E Present address:
F Present address:
G Present address:
H Present address:
Abstract
Development of the Australian Fire Danger Rating System began in 2017 with a project aimed at demonstrating the feasibility of a new fire danger rating system through a Research Prototype (AFDRSRP) that accounted for variability in Australian vegetation types, was nationally applicable, modular and open to continuous improvement.
In this manuscript, we identify and define transition points and categories for the AFDRSRP. We discuss user responses to the categorisation during a live trial evaluation of the AFDRSRP and reflect on limitations and potential improvements.
A review of available literature, broad consultation with stakeholders and reanalysis of fire impact data were used to determine suitable thresholds for categorising fire danger within the AFDRSRP.
Fire danger categories within the AFDRSRP reflect transitions in fire behaviour that result in application of different fire management strategies or are associated with variation in serious consequences and impacts.
The AFDRSRP incorporated the best available science, supported by a well-defined framework for categorising and defining fire danger making it suitable for application across Australian fire jurisdictions and range of fuel types.
The framework allows fire managers to assess the accuracy and appropriateness of forecasted fire danger.
Keywords: bushfire risk assessment, consequences, categorical thresholds, difficulty of suppression, fire behaviour, fire danger rating, forecast system, fuel type.
Introduction
Prior to September 2022, when the Australian Fire Danger Rating System (AFDRS) was implemented operationally across Australia, forecasting fire danger had remained largely unchanged since the late 1950s and 1960s when McArthur’s fire danger indices for forests and grasslands were developed (McArthur, 1958, 1960, 1967). The McArthur approach used the relationship between fire danger and fire behaviour to provide a daily estimation of difficulty of suppression and fire spread using meteorological forecasts. Few alternative approaches or revisions influenced operational practice on a national scale prior to 2022 (Hollis et al. 2024) despite advances in fire behaviour science in Australian vegetation (e.g. Cheney et al. (1998, 2012), Anderson et al. (2015), Cruz et al. (2013)), possibly due to a philosophical separation of fire danger from fire behaviour (Cheney and Gould 1995) together with the enormity of the task at hand. To improve fire danger forecasting to suit local and operational needs, many fire managers adopted their own variations of categorical definitions based on the indices. In 2014, the Australia and New Zealand Emergency Management Committee and Ministerial Council for Police and Emergency Management agreed that development of a new, national fire danger rating system was a national priority. This specifically included changes to better utilise the latest science and understanding of fire behaviour and to provide a more accurate way of assessing fire risk to support better decision making. The revision would also allow fire agencies to confidently deliver clearer information to the community (Australian Emergency Management Committee 2011). Subsequently, requirements of the new system were determined in 2015 by the National Fire Danger Rating Working Group (Cube Group 2015). Development of a Research Prototype (AFDRSRP) began in 2017 to demonstrate the feasibility of a system that accounts for variability in Australian vegetation types, was nationally applicable, modular and open to continuous refinement and improvement. A live trial was conducted (October 2017–March 2018) to assess performance and reliability of the AFDRSRP (Grootemaat et al. 2024).
Categorising fire danger by breaking up a continuous fire danger index metric into discrete classes is fundamental to deriving a fire danger rating system. This enables timely dissemination of important messages relating to probable fire danger to operational fire practitioners and the community. Early approaches such as that used by McArthur (1967), categorised an index against measures of suppression difficulty. Frequency of occurrence of a given fire danger index has also been used to define category boundaries (e.g. Van Wagner (1974)). In this approach the average number of days that a given fire danger category is prescribed. For example, if an ‘extreme’ category occurs on average for 3% of the days in the fire season, then the category lower threshold is defined as the 97th percentile of the fire danger index. In Canada, provinces and territories use this method to define the fire danger classes (Stocks et al. 1989) of the Canadian Forest Fire Weather Index System (Van Wagner 1987). A refinement of this approach links the fire danger index and fire activity, e.g. number of fires, number of fires above a certain size or area burned (Andrews et al. 2003; Alexander 2008). In Portugal, Viegas et al. (2004) derived fire danger category thresholds by analysing the relationship between wildfire occurrence and the Fire Weather Index (FWI: Van Wagner 1987) at a district level providing a daily forecast for number of fires and area burnt related to the fire danger category.
Methods based on fire danger climatology do not account for differences in observed fire behaviour across the spectrum of fuel types, with the possibility of encountering heightened levels of fire behaviour activity under moderate fire danger levels (Alexander 2008). Alexander (2008, 2010) suggests a categorical approach based on fire behaviour interpretations can overcome this.
In Australia the original index scale for both forest and grass fuels was subdivided into five fire danger categories for reasons of simplicity; i.e. Low, Moderate, High, Very High and Extreme (McArthur 1966, 1967). These were subsequently revised to six in 2009 (Figs 1 and 2) with introduction of additional Severe and Catastrophic along with merging Low and Moderate categories (Australian Emergency Management Committee - National Bushfire Warnings Taskforce 2009). Operational adoption of these has varied, largely without integration into fire management publications and materials. Fogarty et al. (2010) later advocated that significant changes to thresholds were needed and that categories did not align adequately to potential community consequences.
Roadside signage communicating categorisation of Australian fire danger rating after revision to six categories in 2009 (source: Jen Hollis).
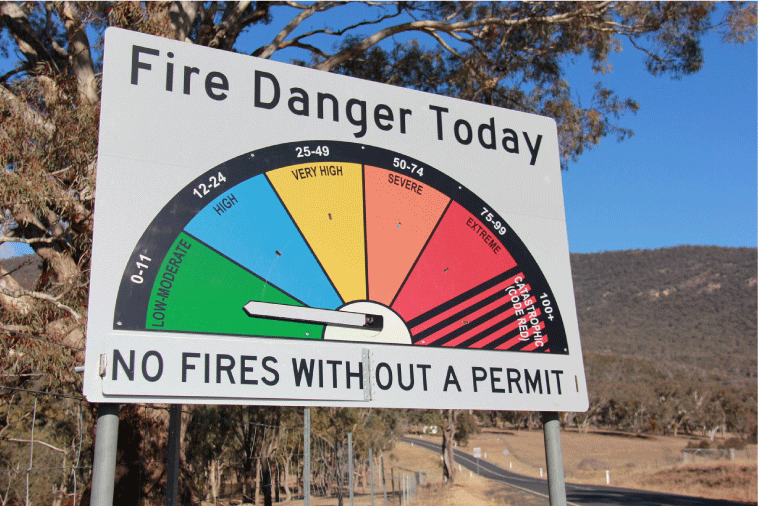
Categorisation of Australian forest fire danger rating on the original index scale, subdivided into five fire danger categories and after revision to six categories in 2009.
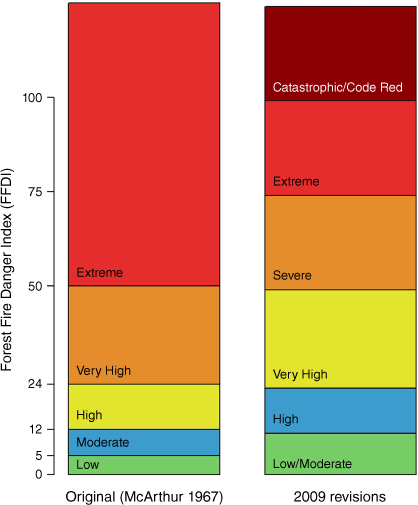
This manuscript is the second of five papers presented in this Special Section on the Australian Fire Danger Rating System. In this manuscript, we identify and present the defined categories and thresholds for the Australian Fire Danger Rating System.
Defining fire danger
Fire danger has many interpretations and applications that have evolved over the past century (Hollis et al. 2024). In Australia, McArthur (1977) expressed fire danger as a function of probable fire behaviour for specified fuel and weather. Until the 1990s, most fire management authorities found this simplified approach sufficient for representing suppression difficulty and setting readiness levels (Cheney et al. 1990). Cheney et al. (1990) preferred to limit fire danger rating to reflect the severity of weather conditions, rather than the combined effects described by McArthur suggesting that if a fire can start and spread, but there are no values (such as human life and property) at risk, there is no fire danger (Cheney 1988). Of course, the difficulty in the detail pertains to the definition of ‘values’, as many variations can be considered.
Arriving at a suitable framework that effectively incorporates current scientific knowledge and understanding of its components and the diversity of operational standards and fire suppression requirements for a wide array of fuel (or vegetation) types can be particularly challenging and complex (Deeming 1983; Muller 1993; Alexander 2010). It is possible that one defined scheme may be limiting or problematic (Cheney 1988; Alexander 2008). Five broad components are commonly identified in literature (e.g. described by Douglas (1957), McArthur and Dwyer (1958), McArthur (1977), Chandler et al. (1983), San-Miguel-Ayanz et al. (2003), National Wildfire Coordinating Group (2011) and Di Giuseppe et al. (2016)) and are in Box 1.
Fire behaviour
Fire danger is intrinsically linked with the sustainability of fire propagation and ensuing fire behaviour. Fire intensity and spotting potential are the primary fire behaviour factors influencing the ability of fire suppression resources to contain a fire. Many operational training manuals have categorised fire danger by fire behaviour, often described as flame height, rate of spread or fireline intensity (Table 1). Other factors including spotting potential, perimeter increase or area growth (Australian Emergency Management Committee - National Bushfire Warnings Taskforce 2009) are used less frequently. These variables include potential candidates for establishing category thresholds for the AFDRSRP.
Type | Variable | Burrows (1984) | Burrows (1986) | Cheney and Sullivan (1997) | Sneeuwjagt and Peet (1998) | Tolhurst and Cheney (1999) | Marsden-Smedley et al. (1999) | NSW Rural Fire Service (2005) | Victorian Country Fire Authority (2005) | Forest Fire Management Group (2007) | Fire and Emergency Services Authority of Western Australia (2009) | Marsden-Smedley (2009) | Queensland Department of National Parks Recreation Sport and Racing (2012) | Western Australian Department of Environment and Conservation (2013) | NSW Rural Fire Service (2014) | Victorian Country Fire Authority (2014) | Victorian Country Fire Authority (2015) | Western Australian Department of Parks and Wildlife (2015) | Western Australian Department of Parks and Wildlife (2016) | Western Australian Department of Parks and Wildlife (2017) | Department of Environment Land Water and Planning (2017) | Shah et al. (2022) | |
---|---|---|---|---|---|---|---|---|---|---|---|---|---|---|---|---|---|---|---|---|---|---|---|
Fire danger | Forest Fire Danger Index | × | × | × | × | × | × | × | × | × | × | × | |||||||||||
Grass Fire Danger Index | × | × | × | × | × | × | |||||||||||||||||
Scrub Fire Danger Rating | × | ||||||||||||||||||||||
Moorland Fire Danger Rating | × | ||||||||||||||||||||||
Fire behaviour | Rate of spread | × | × | × | × | × | × | ||||||||||||||||
Flame height/length | × | × | × | × | × | × | × | ||||||||||||||||
Fire intensity | × | × | × | × | × | × | × | × | |||||||||||||||
Seasonal dryness | Soil Dryness Index | × | × | × | × | ||||||||||||||||||
Keetch–Byram Drought Index | × | × | |||||||||||||||||||||
Fuel moisture | Fine fuel moisture content | × | × | × | × | × | × | × | |||||||||||||||
Hazard stuck moisture | × | ||||||||||||||||||||||
Surface moisture content | × | × | × | ||||||||||||||||||||
Profile moisture content | × | ||||||||||||||||||||||
Available Fuel Factor | × | ||||||||||||||||||||||
Weather | Wind speed (10 m) | × | × | × | × | × | |||||||||||||||||
Wind speed (2 m) | × | ||||||||||||||||||||||
Relative humidity | × | × | × | × | × | × | |||||||||||||||||
Temperature | × | × | × | × | × | ||||||||||||||||||
Days since rain | × | × | |||||||||||||||||||||
C-Haines | × | ||||||||||||||||||||||
Fuel characteristics | Grass curing | × | × | × | × | ||||||||||||||||||
Fine fuel load | × | ||||||||||||||||||||||
Other | Topography | × | |||||||||||||||||||||
Fire size | × |
Difficulty of control
An assessment of suppression difficulty indicates the potential effort needed to contain and mop-up a fire based on expected behaviour (McArthur and Dwyer 1958; Merrill and Alexander 1987; Alexander 2008). Descriptions of suppression difficulty together with suggested measures of control have been associated with fire danger rating in Australia since early systems were developed in the 1930s and 1940s (Gisborne 1933; Wallace 1936; Cromer 1946; Douglas 1957; McArthur and Dwyer 1958) and are often found in training manuals and fire danger publications (Table 1). Similar descriptions of suppression effort relating to fire danger and behaviour are used outside Australia (e.g. Andrews (1987); de Groot (1987); Alexander and Lanoville (1989); Alexander (2008)).
Fire consequences
McArthur-based fire danger forecasts lack an adequate alignment with community loss or the destructiveness of a fire (Fogarty et al. 2010; Harris et al. 2012; Kilinc et al. 2013). A relationship between energy release and community loss was determined by Harris et al. (2012) for a dataset from southern Australia, allowing the estimation of the likelihood of community impacts. The measure (described as the power of the fire) was calculated using fire perimeter or fireline length. Kilinc et al. (2013) reported a similar relationship using fireline intensity that could be used to predict likelihood of community loss in the absence of fireline length.
Blanchi et al. (2010) and Blanchi et al. (2014) established an important connection between wind speed, Forest Fire Danger Index (FFDI) and consequences such as potential house loss and fatalities using a historical dataset of fire events. Blanchi et al. (2014) found fire events involving five or more deaths historically occurred on days where the FFDI at 13:00 hours was over 50 (Extreme rating) with particular weather thresholds (temperatures above 33°C, wind speeds above 24 km h−1 and relative humidity below 16%). Importantly, Blanchi et al. (2012) recognised there was a prominence of life loss associated with the time of a wind change as well as with proximity to forest fuel types, with over 78% of all fatalities occurring within 30 m of forest. Many post-bushfire surveys have established relationships predicting possible community loss, however as Blanchi et al. (2012) point out, most studies do not provide adequate descriptions of observed fire behaviour.
A large component of fire danger is dependent on values at risk (Cheney 1988). For example, potential fatalities or property loss may be more dependent on environmental circumstances, particularly terrain and spatial relationships on the wildland/urban interface (such as the distance to wildland vegetation) than on fire behaviour or suppression difficulty (McArthur and Cheney 1967; Ramsay et al. 1987; Ahern and Chladil 1999; Chen and McAneney 2004; Leonard and Blanchi 2005; Crompton et al. 2010; Gibbons et al. 2012; Newnham et al. 2012). Fogarty et al. (2010) also note that a grassland fire is unlikely to occur with the same degree of potential threat to life and property as a forest fire burning under Catastrophic conditions highlighting the importance of fuel specific fire danger rating approaches.
Ignition potential (both probability and potential number of ignition sources)
The probability of fire ignitions in the landscape is dependent on several factors including an alignment of suitable weather conditions together with either human (including infrastructure) or natural sources of ignition (Penman et al. 2013; Collins et al. 2015; Miller et al. 2017). McArthur’s system did not capture likelihood of both human induced and natural causes of ignition.
Fuel hazard and fuel availability (sustainability of the event)
Moisture content of fuels and associated fuel availability influences the likelihood of sustained fire ignition and the rate of fire growth (Sullivan 2017). It also determines how much fuel will be consumed, the resultant heat release and suppression difficulty (including the occurrence of hold-over fires). It is a driving variable in many fire spread models used operationally in Australia (Cruz et al. 2015).
Materials and methods
To develop meaningful fire danger categories with practical outcomes for the AFDRSRP, we identified thresholds where fire behaviour and therefore operational responses and strategies change or there is potential for increase in the severity of consequences. We assumed that two fire danger rating categories would be too few and that eight would be too many. We drew upon: (1) a review of literature including publications, reports and training manuals for established fire danger and fire behaviour relationships, noting particular threshold values that identify changes in management and decision-making (Table 1); (2) an analysis of wildfire impacts; and (3) a process of consultations with fire managers.
Development of the AFDRSRP focused on operationally relevant transition points and categories based on potential fire behaviour, control, consequences, conditional on ignitions, and for free burning fires without suppression. Probability of ignition, and the likely number of fires, which is arguably important to fire danger, was not included at this time. The resulting framework was not established to extend to public notifications (or warnings) or levels of community readiness and preparedness.
Analysis of wildfire impacts
The wildfire dataset assembled by Kilinc et al. (2013) was reanalysed to identify transition points that mark significant differences in potential consequences. The dataset included 337 fire runs propagating in southern Australian fuel types comprising forests, grasslands and mixed vegetation types. Data was derived from bushfire case studies, reports, aerial imagery (photography and line scans), and newspaper articles. A large portion of the dataset came from Victoria and Western Australia.
Using a fractional loss model, Kilinc et al. (2013) found fireline intensity (IB) provided a useful predictor of the likelihood of community loss. Based on this, we tested different fireline intensity thresholds that would lead to significant differences in potential percent house losses. The following step-wise model was fitted to the data:
where m1, m2 are percentage house loss and T is an IB threshold. As the data were fractional loss, binomial errors were assumed, m1, m2 and T were fitted using generalised linear modelling with the glm function in the statistical package R (R Core Team 2018), and the overall residual sum of squares from the glm models was minimised.
Calculation of IB assumed a nominal heat content value of 18 600 kJ kg−1 in calculations of fireline intensity (Byram 1959). An outlier in the Kilinc et al. (2013) dataset (identified as one of the runs of the Kilmore East fire), was removed from calculations because the value far exceeded that published by Cruz et al. (2012) and because the point would have disproportionately influenced outcomes.
Consultation
A process of consultation was established with key stakeholders, namely fire scientists and fire managers across Australia to understand jurisdictional variations in interpretation and response to different fire danger levels and to identify potential candidate thresholds for categorisation. Consultation occurred by way of workshops, surveys and practical exercises. Through stakeholders, transition points were identified with defined fire danger categories against each fire spread model (NSW Rural Fire Service 2017).
After a live trial evaluation of the AFDRSRP (Grootemaat et al. 2024), a survey of participants was used to provide an assessment of fire danger categories. This information was collated and used as a basis for small working group discussions to target improvements of categorical descriptions for both Forest and Grassland rating definitions in the context of background and associated literature (Matthews et al. 2019).
Results
The fire danger framework and description involved two steps: (1) identifying transition points; and (2) defining fire danger categories for each fuel type against indicative fire behaviour and weather, prescribed burn implications, fire suppression implications and consequences.
The consultation process with stakeholders informed the identification of transition points and what was needed to define useful fire danger categories. Stakeholders were asked ‘what operational differences existed between an FFDI of 60 (Severe), compared to an FFDI of 100 or greater (Catastrophic)’. Fire managers described differences relating to the level of readiness or preparedness for pre-deployment of resources to strategic locations (NSW Rural Fire Service 2017). Differences in escalation of notifications including public warnings, fire bans and restrictions to industry (e.g. harvest bans) and public places (e.g. park closures) were also described. A decrease in confidence in fire spread models alongside an increase in liaison and integration between state and local governments and industry was also identified. No differences in fire behaviour or strategic suppression options were recognised suggesting that at above FFDI of 50 (Severe, Extreme and Catastrophic) thresholds could be better linked to the variation in potential consequences.
Transition points
The consultation process and literature review identified up to five transition points to set thresholds (Tables 2−6, Fig. 5). Fire behaviour and variables to determine each transition point differ between fuel types’ structural characteristics. Likewise, operational strategies and potential impacts vary with fuel type and proximity and value of assets. As proposed by Alexander (2008), points of transition and descriptions that define each fire danger category were uniquely aligned with each fuel type via the associated fire spread model. Simpler fuel complexes, such as spinifex and temperate shrublands, were identified as requiring fewer transition points.
Transition Point 1 | |||
---|---|---|---|
The point where a fire becomes self-sustaining (below which a fire is likely to self-extinguish). | |||
Fire spread model | Threshold | Background to support measure | |
Forest | IB: 100 kW m−1 | Estimated, looking at various experimental burns and case studies that were not self-sustaining. | |
Grassland | IB: 50 kW m−1 | Working estimate, based on expert opinion. | |
Spinifex | SI: 0 | Based on the Spread Index threshold below which fire is ‘unlikely to spread’ (Spread Index ≤0) from Burrows et al. (2018). | |
Pine | IB: 70 kW m−1 | Below 70 kW m−1, fire intensity is likely to be too low to achieve prescribed burning objectives in Pinus elliottii stands (Wade 1983). | |
Northern Grassland (savanna) | IB: 100 kW m−1 | Fireline intensity <100 kW m−1 for self-extinguishing fires (Department of National Parks Recreation Sport and Racing 2012: adapted from Edwards, A 2009-Bushfire CRC). | |
Mallee-Heath | SSSF: 50% | Likelihood of sustained propagation threshold is 50% (Cruz et al. 2015) below which, fire is unlikely to self-sustain. | |
Shrubland | IB: 50 kW m−1 | Working estimate, based on expert opinion. | |
Buttongrass | ROS: 30 m h−1 | Based on Threshold 1, as specified by Marsden-Smedley et al. (1999). |
IB, fireline intensity; SI, spread index; SSSF, probability of a self-sustained surface fire; ROS, forward rate of spread. Fire spread models that were applied are: Forest, Cheney et al. (2012); Grassland, Cheney et al. (1998); Spinifex, Burrows et al. (2018); Pine, based on Cruz et al. (2008); Northern Grassland, Cheney and Sullivan (2008); Mallee-Heath, Cruz et al. (2013); Shrubland, Anderson et al. (2015); Buttongrass, Marsden-Smedley and Catchpole (1995a), Marsden-Smedley and Catchpole (1995b).
Transition Point 2 | |||
---|---|---|---|
Upper limit recommended for prescribed burn conditions. | |||
Fire spread model | Measure | Background to support measure | |
Forest | IB: 750 kW m−1 | Estimated, based on the point above which short distance spotting is increasingly likely. 750 kW m−1 maximum intensity was established as the recommended upper limit for burning Silvertop Ash Forests (Cheney et al. 1992). | |
Cheney (1991) suggests that fire control with hand tools becomes unlikely above 1000 kW m−1. Under approximately 1200 kW m−1, initial attack is likely to be successful and fire behaviour did not increase by a factor of three and could be contained using ‘usual’ resource availability and within the first 8 h after initial attack (McCarthy and Tolhurst 1998). | |||
Grassland | IB: 2000 kW m−1 | In the absence of trees, fires are unlikely (approx. 29%) to breach a small (3 m) firebreak under 2000 kW m−1 (Wilson 1988). This likelihood is even less (5%) for firebreaks >5 m. | |
Spinifex | SI: 2 | Based on approximations assuming an approx. 2500 m h−1 maximum rate of spread for prescribed burning (Burrows, N and Butler, R, pers. comm.). | |
Pine | IB: 700 kW m−1 | Above 700 kW m−1, fire intensity has negative impacts on forest stand and forest floor productivity making it too high to be considered optimal for prescribed burning in Pinus elliottii stands (Wade 1983). Short distance spotting is increasingly likely. Cruz et al. (2008) estimate that PPPY surface fires are 6–600 m h−1. | |
Northern Grassland (savanna) | IB: 4000 kW m−1 | Maximum intensity recommended for burning grasslands is 2000 kW m−1 (Department of Parks and Wildlife 2013) however prescribed burning is often conducted above this threshold (Howard, T and McCaw, L, Pers. Comm.) | |
Fireline intensity should be within 100–10 000 kW m−1 (Department of National Parks Recreation Sport and Racing 2012). | |||
Fires >2000 kW m−1 create a mosaic of burnt and unburnt patches (Tropical Savannas CRC 2001) together with the prescribed burn implications from Allan et al. (2003). | |||
When trees are present, fires are unlikely (approx. 35%) to breach a 10 m firebreak under 5000 kW m−1 (Wilson 1988). | |||
Mallee-Heath | SSSF: 50% and CFO: 33% | Surface fires start becoming influenced by intermittent crowning but largely contained within road networks and fuel breaks. Likelihood of crown fire propagation is 33% (Cruz et al. 2015). | |
Shrubland | IB: 500 kW m−1 | Point above which fires tend to crown intermittently and active suppression may be required. Prescribed burn conditions based on scrub-rolled fuel (Butler, R, Pers. Comm.). | |
Buttongrass | ROS: 450 m h−1 | Based on Threshold 2, as specified by Marsden-Smedley et al. (1999). |
IB, fireline intensity; SI; spread index; SSSF, probability of surface fire propagation; CFO, crown fire occurrence; ROS, forward rate of spread; PPPY, Pine Plantation Pyrometrics model.
Fire spread models that were applied are: Forest, Cheney et al. (2012); Grassland, Cheney et al. (1998); Spinifex, Burrows et al. (2018); Pine, based on Cruz et al. (2008); Northern Grassland, Cheney and Sullivan (2008); Mallee-Heath, Cruz et al. (2013); Shrubland, Anderson et al. (2015); Buttongrass, Marsden-Smedley and Catchpole (1995a), Marsden-Smedley and Catchpole (1995b).
Transition Point 3 | |||
---|---|---|---|
(a) Upper limit for effective ‘offensive’ fire management strategies above which ‘defensive’ strategies are increasingly applied. Transition to increased likelihood of medium distance spotting. Upper limit of effective use of aerial suppression to directly control a fire. | |||
Fire spread model | Measure | Background to support measure | |
Forest | IB: 4000 kW m−1 | 4000 kW m−1 is the widely agreed threshold for ‘offensive’ suppression strategies (Country Fire Authority 2005; NSW Rural Fire Service 2005; Fire and Emergency Services Authority of Western Australia 2009: citing Muller 2008). | |
Above 4000 kW m−1 aerial suppression resources are likely to be largely ineffective at directly controlling a fireline (Hirsch and Martell 1996; Wotton et al. 2017). | |||
Under approximately 4000 kW m−1 (3836 kW m−1), extended first attack is likely to be successful whereby fires are likely to be controlled at a reasonable size (<400 ha) and in a relatively short amount of time (<24 h) (McCarthy and Tolhurst 1998). Transition point identified for application by Beaver (unpubl. data) for the CFA Victoria. | |||
As fireline intensity increases above 2000 kW m−1 up to 4000 kW m−1 offensive aerial suppression strategies are likely to decrease in their effectiveness at holding a fire, particularly if unsupported effectively by ground crews, but are still likely to have a damping effect, impacting fire progression and development (Loane and Gould 1986; Plucinski et al. 2007). | |||
Grassland | IB: 8000 kW m−1 | Estimated. In the absence of trees, fires are approx. 88% and 98% likely to breach a small (3 m) firebreak for fires over 5000 and 10 000 kW m−1 respectively (Wilson 1988). | |
Pine | IB: 4000 kW m−1 | Estimated based on the Forest threshold together with PPPY onset of crowning (Cruz et al. 2008). |
(b) Upper limit for containment within established road networks and fuel breaks without the need for additional suppression. Above which fires tend to be damaging and active suppression may be required when in close proximity to assets. | |||
---|---|---|---|
Spinifex | SI: 10 | Working estimate, based on expert opinion (Burrows, N and Butler, R, pers. comm.). | |
Damaging wildfires with potential to threaten life, structural assets and conservation/cultural assets (Burrows and Butler 2013). | |||
Northern Grassland (savanna) | IB: 2000 kW m−1 | Scorch & Leaf char height based on curve from Tropical Savannas CRC (2001) with fires >2000 kW m−1 typically burn all available fuel. | |
Conditions >2000 kW m−1 best suited for controlling woody plants (Tropical Savannas CRC 2001). |
(c) Fires transition to active crown fires often requiring active suppression around assets with insufficient breaks. | |||
---|---|---|---|
Mallee-Heath | CFO: 66% | Likelihood of crown fire propagation is 66% (Cruz et al. 2015). |
(d) Fires transition to active crown fires quickly, often requiring active suppression but mostly contained by established road networks and fuel breaks. | |||
---|---|---|---|
Shrubland | IB: 4000 kW m−1 | Working estimate, based on expert opinion. |
(e) Based on Threshold 3, as specified by Marsden-Smedley et al. (1999a). | |||
---|---|---|---|
Buttongrass | ROS: 1020 m h−1 | Based on Threshold 3, as specified by Marsden-Smedley et al. (1999). |
IB, fireline intensity; SI; spread index; SSSF, probability of surface fire propagation; CFO, crown fire occurrence; ROS, forward rate of spread; PPPY, Pine Plantation Pyrometrics model.
Fire spread models that were applied are: Forest, Cheney et al. (2012); Grassland, Cheney et al. (1998); Spinifex, Burrows et al. (2018); Pine, based on Cruz et al. (2008); Northern Grassland, Cheney and Sullivan (2008); Mallee-Heath, Cruz et al. (2013); Shrubland, Anderson et al. (2015); Buttongrass, Marsden-Smedley and Catchpole (1995a), Marsden-Smedley and Catchpole (1995b).
Transition point 4 | |||
---|---|---|---|
(a) The threshold above which community losses may be expected. | |||
Fire spread model | Measure | Background to support measure | |
Forest | IB: 10 000 kW m−1 | 97% of all house loss has historically occurred at intensities above 10 000 kW m−1 (Kilinc et al. 2013) with long distance spotting more likely. | |
Upper limit of effective use of aerial suppression to hold a fire. Above 10 000 kW m−1 aerial resources will not be effective at holding fire (Hirsch and Martell 1996; Wotton et al. 2017). | |||
Transition point identified for application by Beaver (unpubl. data) for the CFA Victoria. | |||
Above 11 000 kW m−1, first attack is likely to be unsuccessful and fire behaviour is likely to increase by more than a factor of three and containment is not possible within 24 h (McCarthy and Tolhurst 1998). | |||
Grassland | IB: 15 000 kW m−1 | Working estimate, based on expert opinion. | |
Pine | IB: 10 000 kW m−1 | Working estimate, based on the Forest threshold. |
(b) Active crown fires with the potential to be damaging with high levels of threat when in close proximity to people and assets. | |||
---|---|---|---|
Mallee-Heath | CFO: 100% | Crown fire propagation is certain (Cruz et al. 2015). |
(c) Fires transition to active crown fires quickly, with potentially damaging consequences and requiring wide fuel breaks. | |||
---|---|---|---|
Shrubland | IB: 20 000 kW m−1 | Working estimate, based on expert opinion. |
(d) Based on Threshold 4, as specified by Marsden-Smedley et al. (1999a). | |||
---|---|---|---|
Buttongrass | ROS: 2040 m h−1 | Based on Threshold 4, as specified by Marsden-Smedley et al. (1999). |
IB, fireline intensity; SSSF, probability of surface fire propagation; CFO, crown fire occurrence; and ROS, forward rate of spread.
Fire spread models that were applied are: Forest, Cheney et al. (2012); Grassland, Cheney et al. (1998); Pine, based on Cruz et al. (2008); Mallee-Heath, Cruz et al. (2013); Shrubland, Anderson et al. (2015); Buttongrass, Marsden-Smedley and Catchpole (1995a), Marsden-Smedley and Catchpole (1995b).
Transition point 5 | |||
---|---|---|---|
(a) Increased likelihood of community loss and significant consequences. | |||
Fire spread model | Measure | Background to support measure | |
Forest | IB: 30 000 kW m−1 | Above 30 000 kW m−1, losses are increasingly likely with 70% house loss occurring historically under these conditions (Kilinc et al. 2013). | |
Grassland | IB: 25 000 kW m−1 | Working estimate, based on expert opinion. | |
Pine | IB: 30 000 kW m−1 | Working estimate, based on the Forest threshold. |
(b) Based on Threshold 5, as specified by Marsden-Smedley et al. (1999a). | |||
---|---|---|---|
Buttongrass | ROS: 4200 m h−1 | Based on Threshold 5, as specified by Marsden-Smedley et al. (1999). |
IB, fireline intensity; ROS, forward rate of spread.
Fire spread models that were applied are: Forest, Cheney et al. (2012); Grassland, Cheney et al. (1998); Pine, based on Cruz et al. (2008); and Buttongrass, Marsden-Smedley and Catchpole (1995a); Marsden-Smedley and Catchpole (1995b).
After identifying transition points, it was necessary to identify a suitable variable to establish thresholds that would enable forecast model development. The consultation process identified rate of fire spread, flame height, flame dimensions and fireline intensity as potential candidates. However, stakeholders were unable to agree on the best variable, possibly due to differences between jurisdictions and applications.
Some transition points are adequately captured by processes within fire behaviour models, as was the case for semi-arid Mallee-Heath and Spinifex fuel types (e.g. identified as probabilities of transitions between self-sustained surface fires or crown fires within the Mallee-Heath model developed by Cruz et al. (2013)). For Buttongrass fuels, we used the categories established by Marsden-Smedley et al. (1999) by directly applying the specified rate of spread thresholds against the identified transition points. Fireline intensity was used as the variable to allocate transition points between categories for the dry eucalypt forest, grassland, exotic pine plantation, northern grasslands (savanna) and temperate shrubland fuel types (Tables 2−6).
We applied fireline intensity as a meaningful and objective threshold (Alexander 2008, 2010) with alignments to a diversity of established relationships with fire behaviour and operational strategies. Fireline intensity is widely used to characterise the active flaming zone and as an index of fire behaviour (Beck et al. 2002) that can be directly related to variables such as flame height or length (Newman 1974; Andrews et al. 2011; Alexander and Cruz 2012a; Davies et al. 2019) or the distance spot fires may be transported (Morris 1987). Fireline intensity also captures variation in fire danger between fuel types where rate of spread alone fails to account for potential heat output; e.g. grassland fires compared to forest fires (Cheney 1976). Fireline intensity captures transitional processes between, for example, surface to crowning fires (Van Wagner 1977; Werth et al. 2011)) and has a statistically significant, positive relationship with the proportion of fuel consumed by both controlled fires and wildfires (Hollis et al. 2011; Werth et al. 2011; Cruz et al. 2017). For woody fuels, this has important practical implications related to fuel load reduction as well as impacts on habitat, carbon and climate change management (Hollis et al. 2011). It can be used to identify appropriate fire behaviour and environmental conditions to target specific prescribed burn objectives (Andrews and Rothermel 1982) and is often used as a proxy of fire severity (Keeley 2009). This includes potential damage to vegetation and trees (McArthur 1962; McArthur and Cheney 1966; Van Wagner 1973; Reinhardt and Ryan 1988; Taylor and Armitage 1996) and the height of lethal crown scorching in forests (Van Wagner 1973; Alexander and Cruz 2012b).
Fireline intensity is associated with suppression difficulty in many ratings of fire danger (Hodgson 1968; Burgan 1979; Andrews and Rothermel 1982; Andrews et al. 2011; Werth et al. 2011). Fireline intensity is used to gauge likelihood of initial-and direct-attack success (Burrows 1984; Loane and Gould 1986; McCarthy and Tolhurst 1998) and effectiveness of aerial suppression (Hirsch and Martell 1996; Plucinski et al. 2007; Wotton et al. 2017) although these relationships are used inconsistently by fire managers in Australia. Fireline intensity can be applied to provide a sensible approximation for the width of firebreak that could be breached (Wilson 1988; Fogarty 1996). It is also indicative of approximate radiation intensity levels (kW m−2), which is important for firefighter safety, particularly in determining safe separation distances between firefighters and flame fronts (Fogarty 1996).
Potential consequences of fire such as house loss can also be estimated through a relationship with fireline intensity (Wilson and Ferguson 1986; Gill 1998; Wang 2006; Geoscience Australia 2007; Harris et al. 2012).
Consequence based transition points
The Kilinc et al. (2013) dataset showed 97% of all house loss has historically occurred at intensities above 10 000 kW m−1 in forest fuel types and so this value was selected as the threshold above which community losses may be expected. An additional threshold was sought to represent an intensity above which percentage house loss increases significantly. The findings of Kilinc et al. (2013) suggested a change in slope of cumulative IB at 50 000 kW m−1 on a logarithmic scale (Fig. 3a); however, on a linear scale, we found no such cut-off (Fig. 3b).
(a) Illustrating a possible change in slope of cumulative loss at fireline intensity of 50 000 kW m−1 on a logarithmic scale (fig. 4.9 from Kilinc et al. (2013)), and (b) showing that no such cut off was apparent on the linear scale.
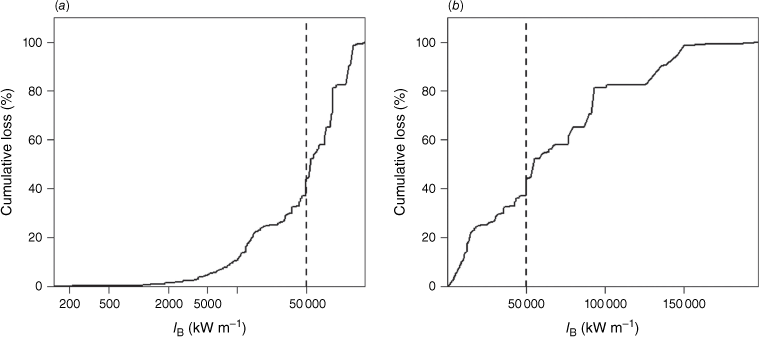
Table 7 shows m1 and m2 for a range of thresholds of IB, together with the corresponding root mean square error (RMSE) (for forest, grassland and mixed vegetation). The range of RMSE values is small, indicating the results are not sensitive to the choice of threshold intensity. For forests, while the minimum RMSE occurs at IB = 55 000 kW m−1 the difference between m1 and m2 is actually larger for a threshold of IB = 30 000 kW m−1. For grassland the minimum RMSE occurs at IB = 45 000 kW m−1 where the largest difference in m1 and m2 occurs. Over the whole data set, the minimum RMSE occurs at 55 000 kW m−1, but the difference between m1 and m2 is similar for a threshold of IB = 30 000 kW m−1. Fig. 4 shows the cut-offs of 10 000 and 55 000 kW m−1 for fires in forest fuels with means in the three categories shown as horizontal bars.
Fireline intensity (kW m−1) | Mean percent loss | m1 – m2 | RMSE | |||
---|---|---|---|---|---|---|
m1 | m2 | |||||
IB < 10 | 10 ≤ IB < T | IB ≥ T | ||||
(a) Forest (n = 135) | ||||||
30 000 | 12.0 (43) | 15.9 (39) | 38.9 (53) | 23.0 | 28.5 | |
40 000 | 12.0 | 18.5 (51) | 40.0 (41) | 21.0 | 28.5 | |
50 000 | 12.0 | 22.2 (57) | 42.5 (35) | 20.2 | 28.1 | |
55 000 (min) | 12.0 | 21.6 (59) | 43.7 (33) | 22.1 | 27.5 |
(b) Grass (n = 162) | ||||||
---|---|---|---|---|---|---|
30 000 | 15.2 (92) | 16.5 (50) | 25.8 (20) | 9.3 | 28.5 | |
40 000 | 15.2 | 16.4 (56) | 28.1 (14) | 11.6 | 28.1 | |
45 000 (min) | 15.2 | 16.3 (58) | 33.7 (12) | 17.4 | 27.3 | |
50 000 | 15.2 | 17.6 (64) | 23.8 (6) | 6.2 | 28.7 |
(c) Mixed fuels (n = 336) | ||||||
---|---|---|---|---|---|---|
30 000 | 15.2 (149) | 16.4 (103) | 36.5 (84) | 20.2 | 28.2 | |
40 000 | 15.2 | 19.1 (125) | 36.9 (62) | 17.7 | 28.0 | |
50 000 | 15.2 | 21.6 (141) | 39.0 (46) | 17.3 | 27.7 | |
55 000 (min) | 15.2 | 20.7 (145) | 41.1 (42) | 20.4 | 27.4 |
The numbers of points are shown in parenthesis. m1 and m2 are percentage house loss and T is an IB threshold.
Percentage loss showing the cut-offs of 10 000 and 55 000 kW m−1 for fires in forest fuels with means in the three categories shown as horizontal bars.
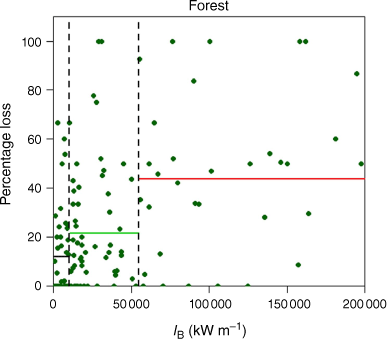
A value with better discriminating power upon which to establish the fifth transition point was not clear in the analyses. A fireline intensity of 30 000 kW m−1 was selected for forest fuels based on evidence from Kilinc et al. (2013) that 86% of house loss in Australia occurred under conditions where this value was exceeded (Table 8). Although the Pearson correlation coefficient between intensity and percentage house loss was only 0.31 in forest fuels, it could be assumed that under these conditions, consequences including house loss are increasingly likely compared to conditions characterised by fireline intensity <30 000 kW m−1 where less than 14% of house losses have occurred. Notably, the correlation with fireline intensity was considerably less in grass fuels (r = 0.06).
Fuel type | Percent house loss by AFDRS Category and fireline intensity thresholds (kW m−1) | ||||||
---|---|---|---|---|---|---|---|
Category 1 | Category 2 | Category 3 | Category 4 | Category 5 | Category 6 | ||
Forest | 0 ≤ IB < 100 | 100 ≤ IB < 750 | 750 ≤ IB < 4000 | 4000 ≤ IB < 10 000 | 10 000 ≤ IB < 30 000 | 30 000 ≤ IB | |
1 | 3 | 11 | 86 | ||||
Grassland | 0 ≤ IB < 50 | 50 ≤ IB < 2000 | 2000 ≤ IB < 8000 | 8000 ≤ IB < 15 000 | 15 000 ≤ IB < 25 000 | 25 000 ≤ IB | |
6 | 29 | 39 | 10 | 17 |
Percentages rounded for clarity.
Development of descriptions defining fire danger categories
Supporting fire danger with well-defined categories is an integral part of the system, ensuring consistency and relevance. Each category for the AFDRSRP was defined in terms of important elements that describe fire danger, namely indicative fire behaviour and fire weather, fire suppression and containment and consequences (an example for the Forest fuel type is in Appendix 1). Implications for prescribed burning for each category were also added at the request of stakeholders to aid identification of broad scale, suitable burning conditions (NSW Rural Fire Service 2017).
For each category, a general description and possible range for fire behaviour variables was provided including rate of spread, flame height and potential spotting. These quantities were derived from existing models (e.g. forest flame heights determined using the equation documented by Noble et al. (1980)) using the range of fireline intensity defining each category. Rate of fire spread was derived by rearranging the fireline intensity equation with a specified range of fuel characteristics. The range in fuel load was estimated using documented observations, together with a higher value representing highest average conditions. Where possible, rates of spread and descriptions of fire behaviour were checked against empirical evidence from experimental and wildfire case studies, particularly in fuel types where calculations were determined based on equations developed for other fuel types. For example, fire behaviour from descriptions documented by Douglas (1964) and case studies from Douglas (1974) were used to cross-check descriptions of fire behaviour for Pine fuels.
The consultation process identified atmospheric instability (Mills and McCaw 2010; Potter 2012) and potential wind changes (Cheney et al. 2001) as important considerations affecting fire behaviour and potential fire danger. Stakeholders determined these variables should not influence the FBI, but be identified via ‘red flag warnings’ for fire managers to consider and interpret using their expertise concerning local implications (Appendix 1a).
Typical prescribed burn conditions were captured within Category 2 (see Appendix 1a–f for Forest fuels) with a lower limit below which fires are unlikely to spread and likely to self-extinguish together with an upper limit, beyond which conditions are likely to make containment particularly difficult for each fuel type. The upper limit was particularly difficult to establish due to variation between fuel types, but also because well-documented, clearly defined and established relationships with fireline intensity are scant other than those described by Cheney (1978) and Cheney et al. (1992). The category itself aimed to capture fire behaviour mostly involving surface, near-surface, elevated and bark fuels (Cheney et al. 2012), which can be adequately contained with appropriate planning and sufficient resources.
Recognising that conditions outside the range of Category 2 may also be suitable for prescribed burning (depending on burn objectives) each category described the implications of conditions on prescribed burning opportunities. Each fuel type was addressed independently with the aim of providing general, helpful descriptors, rather than rules or a replacement to using appropriate fire behaviour models or prescribed burn planning.
Descriptions for fire suppression and containment provided sufficient information to aid preparation and readiness setting but were general enough not to limit operational application of possible containment (or delayed containment) strategies. The consultation and review process established that McArthur descriptions of suppression difficulty broadly reflected Australian practice, so descriptions of fire suppression and containment were largely based on McArthur alignments with fire danger (Table 1) capturing common themes, language and strategies used to describe fire containment, suppression and potential difficulties observed. Additional technical descriptions were incorporated (e.g. potential aircraft support, initial attack strategies) to better reflect modern suppression strategies or local practices. Important messages about firefighter safety were also described, including red flag warnings for potential wind changes associated with ‘dead man zone’ events (Cheney et al. 2001).
McArthur (1966) captured potential fire impacts in terms of fire area within the Grassland Fire Danger system by including the maximum burnt area at various times (0.5, 1, 2 and 4 h) as well as the average final fire size. Fogarty and Alexander (1999) also provided estimates of forward spread distance, perimeter length and maximum breadth for four elapsed time periods (0.5, 1, 1.5 and 2 h). Potential fire area and perimeter (credible worst case) for the AFDRSRP for 0.5-h and 4-h fire runs (assuming no suppression) were provided for each category. A length to breadth ratio (L:B) and fuel load best suited to the fuel type (Cruz et al. 2015) with wind speeds ranging from 10 to 40 km h−1 was applied. The potential fire area and perimeter were based on maximum potential rate of spread for the category rather than the mean. Fire area and perimeter calculations assumed a worst-case scenario resulting in estimates that will in most cases be considerably exaggerated compared to what is observed. ‘Reference time to five hectares’ was provided at the request of stakeholders for native Forest, Grassland and Pine forest fuel types based on the shortest time for each category under a reference set of conditions suited to the fuel type.
While fire danger and weather conditions described by Blanchi et al. (2010) and Blanchi et al. (2012) are indicative of potential fatalities, without direct links to fire behaviour variables (such as rate of spread, fireline intensity or flame height) we were unable to use them to forecast potential consequences in the AFDRSRP. Instead, possible consequences were described in the context of potential for life and house loss.
For Forest and Grassland fuel types, the percentages of house loss that has historically occurred under these conditions were estimated using the Kilinc et al. (2013) dataset. For other fuel types (e.g. Spinifex, Northern Grassland), we extrapolated general descriptions of consequence in consultation with fire practitioners and subject matter experts.
Live trial performance and revisions
Of the 28 live trial participants who returned evaluations or case study observations during the 2017/2018 data collection period, 16 completed post-trial surveys. This included at least two responses from each jurisdiction except the Northern Territory. A total of 94% of respondents reported that the AFDRSRP framework would enable improved understanding and decision making. Responses showed that the four descriptive categories: (1) fire behaviour and fire weather; (2) implications for prescribed burning; (3) fire suppression and containment; and (4) consequences) encompassed fire danger adequately, however each of the specific descriptions could be improved to better communicate important messages. The general description of ‘Relevance' and 'Indicative fire behaviour and fire weather’ sections (Appendix 1a–e) were considered most important.
Small working groups for native Forest and Grassland fuel types provided improved definitions for each category (Matthews et al. 2019) and it was agreed that AFDRSRP thresholds and transition points were appropriate. Revisions were made associated with improved descriptions, terminology and incorporating additional important information. General improvements (e.g. language, layout) were also made to all categorical definitions.
Discussion
The encouraging, positive performance of the AFDRSRP (Grootemaat et al. 2024) suggests that the thresholds applied and categorical definitions provided, enable effective communication of fire danger to operational fire practitioners. Key knowledge gaps were identified together with suggestions for additional investigation of threshold accuracy and exploration of ‘consequence’ datasets.
Applying fireline intensity to delineate fire danger categories worked well for the AFDRSRP however it was not without its issues. It is not easily measured or visualised by fire practitioners making it difficult to ascertain and compare across fuel types. In addition to this, of the three variables used to calculate fireline intensity, fuel load can be difficult to estimate accurately, potentially introducing large amounts of error into the delineation of fire danger categories (Cheney 1990). For fuel types where fuel load (or hazard) is used to determine rate of spread, a bias or erroneous estimation of fuel load results in the variable effectively being applied twice for calculations. Potential errors arising from the use of fuel load may be mitigated by improving fuel related data quality and through adequate practitioner training programs and resources. Even with relatively accurate estimations of fuel load, the use of fireline intensity may mean that some conditions are not suitably representing potential fire danger. For example, in fast, wind-driven grassfires in low fuel loads associated with largely eaten-out fuels, potential consequences may be linked more to associated spotting rather than the passage of the headfire, as was the case with two separate runs of the 1983 Cudgee fire in Victoria where 28 and 31 (houses were destroyed predominantly due to spotting (Kilinc et al. 2013). Further investigation is needed to confirm how these conditions could be best represented within the AFDRS.
Given the necessary complexity of fire danger forecasting together with evaluation and improvements that can only come with time and scientific advances, it would be unrealistic to expect that any new system would be complete and performing consistently well in a relatively short development period (Jolly 2009). It is essential that managers of the system commit to continuous improvement driven by a combination of scientific knowledge and documented operational experience (Luke and McArthur 1978; Taylor and Alexander 2006) with a team of skilled individuals that provide oversight and calibration (Jolly 2009). Analysis of fire events through case studies is also particularly important to inform and use an evolving system. Any changes over time to the AFDRS (e.g. by incorporating new fire spread models) will have subsequent impacts on various linked components (e.g. thresholds used to delineate categories, fuel layers). In a system where continuous improvement is important, this must always be a consideration with revisions requiring careful scrutiny and investigation prior to implementation (Cheney and Gould 1995).
Concluding remarks
There have been many advances in recent years to better understand and predict fire behaviour and fire danger including development of more accurate and suitable fire spread models and improved understanding of the roles of drought and atmospheric stability in fire development. The AFDRSRP incorporated the best available science, supported by a well-defined framework for categorising and defining fire danger that allows for continuous improvement. The AFDRSRP defined fire danger using four descriptive groups suitable for application across Australian fire jurisdictions and range of fuel types. Initial evaluation of the AFDRSRP through a live trial during the 2017/2018 fire season showed that the framework could improve end-users ability to forecast and understand fire danger in Australia resulting in better support for a range of fire management decision making.
The framework allowed fire managers to assess the accuracy and appropriateness of forecasted fire danger. The framework may also enable better planning, preparedness, resourcing and determination of suppression tactics. By having one national framework for defining and categorising fire danger it is hoped an environment suitable for easier agency and departmental liaison is created. It should also enable improved identification of opportunities to further develop fire behaviour and fire danger forecasting skill alongside advances in science and experience.
Data availability statement
Data sharing is not applicable as no new data were generated or analysed during this study.
Declaration of funding
The AFDRS was funded by grants from the Australian Government’s National Emergency Management Projects programme and the NSW Rural Fire Service’s Bush Fire Risk Mitigation and Resilience Programme. The project also received considerable in-kind support from the Bureau of Meteorology and the NSW Rural Fire Service.
Author contributions
J. Hollis: literature review, methodology and framework development, consultation, original draft, writing, review and editing. S. Matthews: website development, live trial data analysis, writing, review and editing. W. Anderson: data analysis for determination of consequence-based thresholds, writing, review and editing. M. Cruz: writing, review and editing. P. Fox-Hughes: climatology, analysis of weather data, writing, review and editing. S. Grootemaat: model code preparation, live trial data analysis, writing, review and editing. B. Kenny: development of national fuel data, writing, review and editing. S. Sauvage: development and support of computer systems, writing, review and editing.
Acknowledgements
The AFDRSRP relied on expertise and participation of many fire managers and researchers and we thank those that were part of early project planning, development and support. We thank operational staff and researchers who contributed to the design and function of the AFDRSRP as well as many staff and volunteers from around Australia who collected live trial observations. We especially thank Jim Gould, Matt Plucinski, Phil Cheney, Lachie McCaw, Neil Burrows, Trevor Howard, Pedro Palheiro, Ryan Butler, Alen Slijepcevic, Tim Wells, Musa Kilinc and Peter Leeson who have provided significant time, expertise and assistance developing categorical definitions and thresholds. We also thank Paulo Fernandes and Marty Alexander for their valuable comments and suggestions throughout development of the system, the Associate Editor for the Special Section, and the two anonymous reviewers for their contributions towards improving our manuscript.
References
Alexander ME (2010) ‘Feasibility study for the setting up of a Global Wildland Fire Danger Rating System.’ (Study Report for Contract No. P200915719ALEX Submitted to: European Commission, Joint Research Centre, Institute of Environment and Sustainability, Land Management and Natural Hazards Unit: Ispra, Italy)
Alexander M, Cruz M (2012a) Graphical aids for visualizing Byram’s fireline intensity in relation to flame length and crown scorch height. The Forestry Chronicle 88, 185-190.
| Crossref | Google Scholar |
Alexander ME, Cruz MG (2012b) Interdependencies between flame length and fireline intensity in predicting crown fire initiation and crown scorch height. International Journal of Wildland Fire 21, 95-113.
| Crossref | Google Scholar |
Allan G, Johnson A, Cridland S, Fitzgerald N (2003) Application of NDVI for predicting fuel curing at landscape scales in northern Australia: can remotely sensed data help schedule fire management operations? International Journal of Wildland Fire 12, 299-308.
| Crossref | Google Scholar |
Anderson WR, Cruz MG, Fernandes PM, McCaw L, Vega JA, Bradstock RA, Fogarty L, Gould J, McCarthy G, Marsden-Smedley JB, Matthews S, Mattingley G, Pearce HG, van Wilgen BW (2015) A generic, empirical-based model for predicting rate of fire spread in shrublands. International Journal of Wildland Fire 24, 443-460.
| Crossref | Google Scholar |
Andrews PL, Loftsgaarden DO, Bradshaw LS (2003) Evaluation of fire danger rating indexes using logistic regression and percentile analysis. International Journal of Wildland Fire 12, 213-226.
| Crossref | Google Scholar |
Beck JA, Alexander ME, Harvey SD, Beaver AK (2002) Forecasting diurnal variations in fire intensity to enhance wildland firefighter safety. International Journal of Wildland Fire 11, 173-182.
| Crossref | Google Scholar |
Blanchi R, Lucas C, Leonard J, Finkele K (2010) Meteorological conditions and wildfire-related houseloss in Australia. International Journal of Wildland Fire 19, 914-926.
| Crossref | Google Scholar |
Blanchi R, Leonard J, Haynes K, Opie K, James M, Oliveira FDd (2014) Environmental circumstances surrounding bushfire fatalities in Australia 1901–2011. Environmental Science & Policy 37, 192-203.
| Crossref | Google Scholar |
Burrows N, Gill M, Sharples J (2018) Development and validation of a model for predicting fire behaviour in spinifex grasslands of arid Australia. International Journal of Wildland Fire 27, 271-279.
| Crossref | Google Scholar |
Chen K, McAneney J (2004) Quantifying bushfire penetration into urban areas in Australia. Geophysical Research Letters 31, 1-4.
| Crossref | Google Scholar |
Cheney NP (1976) Bushfire disasters in Australia, 1945–1975. Australian Forestry 39, 245-268.
| Crossref | Google Scholar |
Cheney NP (1990) Quantifying bushfires. Mathematical and Computer Modelling 13, 9-15.
| Crossref | Google Scholar |
Cheney N, Gould J, Catchpole W (1998) Prediction of fire spread in grasslands. International Journal of Wildland Fire 8, 1-13.
| Crossref | Google Scholar |
Cheney P, Gould J, McCaw L (2001) The Dead-Man Zone - A neglected area of firefighter safety. Australian Forestry 64, 45-50.
| Crossref | Google Scholar |
Cheney NP, Gould JS, McCaw WL, Anderson WR (2012) Predicting fire behaviour in dry eucalypt forest in southern Australia. Forest Ecology and Management 280, 120-131.
| Crossref | Google Scholar |
Collins KM, Price OF, Penman TD (2015) Spatial patterns of wildfire ignitions in south-eastern Australia. International Journal of Wildland Fire 24, 1098-1108.
| Crossref | Google Scholar |
Cromer DAN (1946) Hygrothermographic fire danger rating and forecasting. Australian Forestry 10, 52-71.
| Crossref | Google Scholar |
Crompton RP, McAneney J, Chen J, Pielke Jr RA, Haynes K (2010) Influence of location, population, and climate on building damage and fatalities due to Australian bushfire: 1925–2009. Weather, Climate, and Society 2, 300-310.
| Crossref | Google Scholar |
Cruz MG, Alexander ME, Fernandes PM (2008) Development of a model system to predict wildfire behaviour in pine plantations. Australian Forestry 71, 113-121.
| Crossref | Google Scholar |
Cruz MG, Sullivan AL, Gould JS, Sims NC, Bannister AJ, Hollis JJ, Hurley RJ (2012) Anatomy of a catastrophic wildfire: the Black Saturday Kilmore East fire in Victoria, Australia. Forest Ecology and Management 284, 269-285.
| Crossref | Google Scholar |
Cruz MG, McCaw WL, Anderson WR, Gould JS (2013) Fire behaviour modelling in semi-arid mallee-heath shrublands of southern Australia. Environmental Modelling & Software 40, 21-34.
| Crossref | Google Scholar |
Cruz MG, Alexander ME, Plucinski MP (2017) The effect of silvicultural treatments on fire behaviour potential in radiata pine plantations of South Australia. Forest Ecology and Management 397, 27-38.
| Crossref | Google Scholar |
Davies GM, Legg CJ, Smith AA, MacDonald A (2019) Development and participatory evaluation of fireline intensity and flame property models for managed burns on Calluna-dominated heathlands. Fire Ecology 15, 30.
| Crossref | Google Scholar |
Di Giuseppe F, Pappenberger F, Wetterhall F, Krzeminski B, Camia A, Libertá G, San Miguel J (2016) The potential predictability of fire danger provided by numerical weather prediction. Journal of Applied Meteorology and Climatology 55, 2469-2491.
| Crossref | Google Scholar |
Douglas DR (1964) Some characteristics of major fires in coniferous plantations. Australian Forestry 28, 119-124.
| Crossref | Google Scholar |
Fogarty LG (1996) Two rural/urban interface fires in the Wellington suburb of Karori: Assessment of associated burning conditions and fire control strategies. Forest Research Bulletin No. 197. Forest and Rural Fire Science and Technology Series Report No. 1. (New Zealand Forest Research Institute, Rotorua in association with New Zealand Fire Service Commission and National Rural Fire Authority: Wellington, New Zealand)
Gibbons P, van Bommel L, Gill AM, Cary GJ, Driscoll DA, Bradstock RA, Knight E, Moritz MA, Stephens SL, Lindenmayer DB (2012) Land management practices associated with house loss in wildfires. PLoS One 7, e29212.
| Crossref | Google Scholar | PubMed |
Gill M (1998) A richter-type scale for fires? Available at http://www.firebreak.com.au/reslet2.html
Gisborne HT (1933) The wood cylinder method of measuring forest inflammability. Journal of Forestry 31, 683-689.
| Google Scholar |
Grootemaat S, Matthews S, Kenny BJ, Runcie J, Hollis JJ, Sauvage S, Fox-Hughes P, Holmes A (2024) Live trial performance of the Australian Fire Danger Rating System- Research Prototype. International Journal of Wildland Fire In press.
| Crossref | Google Scholar |
Harris S, Anderson W, Kilinc M, Fogarty L (2012) The relationship between fire behaviour measures and community loss: an exploratory analysis for developing a bushfire severity scale. Natural Hazards 63, 391-415.
| Crossref | Google Scholar |
Hirsch KG, Martell DL (1996) A review of initial attack fire crew productivity and effectiveness. International Journal of Wildland Fire 6, 199-215.
| Crossref | Google Scholar |
Hodgson A (1968) Control burning in eucalypt forests in Victoria, Australia. Journal of Forestry 66, 601-605.
| Google Scholar |
Hollis JJ, Matthews S, Cruz MG, Fox-Hughes P, Grootemaat S, Heemstra S, Kenny BJ, Savage S (2024) Intruduction to the Australian Fire Danger Rating System. International Journal of Wildland Fire In press.
| Crossref | Google Scholar |
Hollis JJ, Anderson WR, McCaw WL, Cruz MG, Burrows ND, Ward B, Tolhurst KG, Gould J (2011) The effect of fireline intensity on woody fuel consumption in southern Australian eucalypt forest fires. Australian Forestry 74, 81-97.
| Crossref | Google Scholar |
Keeley JE (2009) Fire intensity, fire severity and burn severity: a brief review and suggested usage. International Journal of Wildland Fire 18, 116-126.
| Crossref | Google Scholar |
Marsden-Smedley J, Catchpole WR (1995a) Fire behaviour modelling in Tasmanian Buttongrass Moorlands. II. Fire behaviour. International Journal of Wildland Fire 5, 215-228.
| Crossref | Google Scholar |
Marsden-Smedley JB, Catchpole WR (1995b) Fire behaviour modeling in Tasmanian buttongrass moorlands, Part 1. International Journal of Wildland Fire 5, 203-214.
| Crossref | Google Scholar |
Marsden-Smedley JB, Rudman T, Catchpole WR, Pyrke A (1999) Buttongrass moorland fire behaviour prediction and management. Tasforests 11, 87-107.
| Google Scholar |
McArthur AG, Cheney NP (1966) The characterisation of fires in relation to ecological studies. Australian Forest Research 2, 36-45.
| Google Scholar |
McCarthy G, Tolhurst KG (1998) ‘Effectiveness of firefighting first attack operations by the Department of Natural Resources and Environment from 1991/92-1994/95.’ (Fire Management Branch & Victoria, Centre for Forest Tree Technology, Fire Management Branch, Dept. of Natural Resources and Environment: East Melbourne, Australia)
Miller C, Plucinski M, Sullivan A, Stephenson A, Huston C, Charman K, Prakash M, Dunstall S (2017) Electrically caused wildfires in Victoria, Australia are over-represented when fire danger is elevated. Landscape and Urban Planning 167, 267-274.
| Crossref | Google Scholar |
Newman M (1974) Toward a common language for aerial delivery mechanics. Fire Management Notes 35, 18-19.
| Google Scholar |
Newnham GJ, Siggins AS, Blanchi RM, Culvenor DS, Leonard JE, Mashford JS (2012) Exploiting three dimensional vegetation structure to map wildland extent. Remote Sensing of Environment 123, 155-162.
| Crossref | Google Scholar |
Noble IR, Bary GAV, Gill AM (1980) McArthur’s fire-danger meters expressed as equations. Australian Journal of Ecology 5, 201-203.
| Crossref | Google Scholar |
Penman TD, Bradstock RA, Price O (2013) Modelling the determinants of ignition in the Sydney Basin, Australia: implications for future management. International Journal of Wildland Fire 22, 469-478.
| Crossref | Google Scholar |
Plucinski MP, Gould JS, McCarthy G, Hollis J (2007) The effectiveness and efficiency of aerial firefighting in Australia, Part 1. Bushfire CRC Technical Report Number A0701. (Bushfire Cooperative Research Centre Program A: Safe prevention, preparation and suppression of bushfires: Melbourne, Australia)
Potter BE (2012) Atmospheric interactions with wildland fire behaviour – I. Basic surface interactions, vertical profiles and synoptic structures. International Journal of Wildland Fire 21, 779-801.
| Crossref | Google Scholar |
Ramsay GC, McArthur NA, Dowling VP (1987) Preliminary results from an examination of house survival in the 16 February 1983 bushfires in Australia. Fire and Materials 11, 49-51.
| Crossref | Google Scholar |
R Core Team (2018) ‘A language and environment for statistical computing.’ (R Foundation for Statistical Computing: Vienna, Austria). Available at https://www.R-project.org/
Reinhardt ED, Ryan KC (1988) How to estimate tree mortality resulting from underburning. Fire Manage Notes 49, 30-36.
| Google Scholar |
San-Miguel-Ayanz J, Carlson JD, Alexander M, Tolhurst KG, Morgan G, Sneeuwjagt RJ, Dudley M (2003) Current methods to assess fire danger potential. In ‘Wildland fire danger estimation and mapping: The role of remote sensing data. Vol. 4’. Series in remote sensing. (Ed. E Chuvieco) Chapter 2. (World Scientific Publishing: Singapore)
Shah SU, Yebra M, Van Dijk AIJM, Cary GJ (2022) A New Fire Danger Index developed by random forest analysis of remote sensing derived fire sizes. Fire 5, 152.
| Crossref | Google Scholar |
Stocks BJ, Lawson BD, Alexander ME, Van Wagner CE, McAlpine RS, Lynham TJ, Dube DE (1989) The Canadian Forest Fire Danger Rating System: an overview. Forestry Chronicle 65, 450-457.
| Crossref | Google Scholar |
Sullivan AL (2017) Inside the inferno: fundamental processes of wildland fire behaviour. Current Forestry Reports 3, 150-171.
| Crossref | Google Scholar |
Taylor SW, Alexander ME (2006) Science, technology, and human factors in fire danger rating: the Canadian experience. International Journal of Wildland Fire 15, 121-135.
| Crossref | Google Scholar |
Taylor SW, Armitage OB (1996) SCORCH: a fire-induced tree-mortality prediction model for Canadian forests. In ‘Integrated forest vegetation management: options and applications’. (Eds PG Comeau, GJ Harper, ME Blache, J Boateng, LA Gileson) (Canadian Forest Service, Pacific Forestry Centre and British Columbia Ministry of Forests, Research Branch: Victoria, British Columbia)
Van Wagner CE (1973) Height of Crown Scorch in forest fires. Canadian Journal of Forest Research 3, 373-378.
| Crossref | Google Scholar |
Van Wagner CE (1977) Conditions for the start and spread of crown fire. Canadian Journal of Forest Research 7, 23-34.
| Crossref | Google Scholar |
Viegas DX, Reis RM, Cruz MG, Viegas MT (2004) Calibração do Sistema Canadiano de Perigo de Incêndio para Aplicação em Portugal. Silva Lusitana 12, 77-93.
| Google Scholar |
Wallace WR (1936) Forest fire wather research in Western Australia. Australian Forestry 1, 17-24.
| Crossref | Google Scholar |
Werth PA, Potter BE, Clements CB, Finney MA, Goodrick SL, Alexander ME, Cruz MG, Forthofer JA, McAllister SS (2011) ‘Synthesis of knowledge of extreme fire behaviour: Volume 1 for fire managers.’ (United States Department of Agriculture, Forest Service, Pacific Northwest Research Station: Portland, Oregon)
Wilson A (1988) Width of firebreak that is necessary to stop grass fires: some field experiments. Canadian Journal of Forest Research 18, 682-687.
| Crossref | Google Scholar |
Wilson AAG, Ferguson IS (1986) Predicting the probability of house survival during bushfires. Journal of Environmental Management 23, 259-270.
| Crossref | Google Scholar |
Wotton BM, Flannigan MD, Marshall GA (2017) Potential climate change impacts on fire intensity and key wildfire suppression thresholds in Canada. Enviromental Research Letters 12, 095003.
| Crossref | Google Scholar |
A Quick Guide Collection containing the complete set of Fire Danger Rating Tables can be found in the Online Appendix.