Propagation probability and spread rates of self-sustained smouldering fires under controlled moisture content and bulk density conditions
Nuria Prat-Guitart A E , Guillermo Rein B , Rory M. Hadden C , Claire M. Belcher D and Jon M. Yearsley AA School of Biology and Environmental Science, Earth Institute, University College Dublin, Dublin D4, Republic of Ireland.
B Department of Mechanical Engineering, Imperial College London, London SW7 2AZ, UK.
C School of Engineering, University of Edinburgh, The King’s Buildings, Mayfield Road, Edinburgh EH9 3JL, UK.
D wildFIRE Lab, Hatherly Laboratories, University of Exeter, Exeter EX4 4PS, UK.
E Corresponding author. Email: prat.nur@gmail.com
International Journal of Wildland Fire 25(4) 456-465 https://doi.org/10.1071/WF15103
Submitted: 26 May 2015 Accepted: 27 December 2015 Published: 3 March 2016
Journal Compilation © IAWF 2016
Abstract
The consumption of large areas of peat during wildfires is due to self-sustained smouldering fronts that can remain active for weeks. We studied the effect of peat moisture content and bulk density on the horizontal propagation of smouldering fire in laboratory-scale experiments. We used milled peat with moisture contents between 25 and 250% (mass of water per mass of dry peat) and bulk densities between 50 and 150 kg m–3. An infrared camera monitored ignition, spread and extinction of each smouldering combustion front. Peats with a bulk density below 75 kg m–3 and a moisture content below 150% self-sustained smouldering propagation for more than 12 cm. Peat with a bulk density of 150 kg m–3 could self-sustain smouldering propagation up to a critical moisture content of 115%. A linear model estimated that increasing both moisture content and bulk density significantly reduced the median fire spread rate (which ranged between 1 and 5 cm h–1). Moisture content had a stronger effect size on the spread rate than bulk density. However, the effect of bulk density on spread rate depends upon the moisture content, with the largest effect of bulk density at low moisture contents.
Additional keywords: fire behaviour, horizontal front, lateral, peat fire, peatland, propagation dynamics.
Introduction
Smouldering is an incomplete form of combustion affecting organic materials such as the peat stored in peatlands and forest soils (Rein 2013). The propagation of smouldering fires is known to be very slow compared with flaming fires, moving at few centimetres per hour (Wein 1983; Frandsen 1991). The consumption of large areas of peat is often caused by self-sustained smouldering fires, which remain active and slowly propagating for weeks or months (Rein 2013).
During a peat fire, the carbon stored in the ground is released to the atmosphere. Incomplete smouldering combustion in peat produces a higher proportion of carbon emissions (e.g. CO, CH4) than flaming fires in vegetation (Hadden 2011). These gases contribute significantly to global emissions of greenhouse gases (Turetsky et al. 2015). Smouldering peat fires also affect the roots of vegetation close to the surface, often causing lethal plant damage and habitat losses (Miyanishi and Johnson 2002; Page et al. 2002; Davies et al. 2013). The landscape after a peat fire is often heterogeneous, as peat is consumed in irregular patches (Shetler et al. 2008). In the burnt areas, deep layers of dense peat become the new surface, with a different constitution and properties (Prat-Guitart et al. 2011). These post-burn surfaces are often opportunities for colonising species and have the potential to change biodiversity (Benscoter and Vitt 2008).
Factors driving smouldering fire ignition
The ignition of a smouldering fire in peat is often caused by a heat source near the surface, such as a lighting strike, adjacent flaming vegetation (Rein 2013) or burning pine cones (Kreye et al. 2013). The start of a smouldering fire is controlled by the properties of the ignition source (intensity and duration), peat conditions (primarily moisture content, bulk density and mineral content) and oxygen availability (Frandsen 1987; Ohlemiller 2002; Hadden et al. 2013; Huang and Rein 2014). Of these, peat moisture content is the main factor limiting the ignition of peat (Van Wagner 1972; Frandsen 1987, 1991). Water in peat acts as a heat sink, requiring a large amount of energy to evaporate the water before the peat reaches temperatures at which the pyrolysis process begins (Rein 2013). The probability of peat ignition and initial horizontal propagation of at least 10 cm from an ignition source has been estimated in previous studies (Frandsen 1997; Lawson et al. 1997; Reardon et al. 2007). When the moisture content (MC) of the peat is between 110 and 200% (gravimetric moisture content, mass of water per mass of dry peat expressed as a percentage), there is a 50% probability of starting a smouldering peat fire (Frandsen 1987; Frandsen 1997; Reardon et al. 2007; Rein et al. 2008). Frandsen (1997) predicted the probability of ignition and early horizontal propagation as a function of MC (%), mineral content (%) and bulk density (kg m–3). Reardon et al. (2007), however, predicted the ignition and early propagation using only moisture and mineral content, suggesting that bulk density was implicitly included in the quantification of the other two peat properties.
Self-sustained smouldering propagation
Once ignited, a smouldering fire propagates by drying and igniting the fuel ahead of the smouldering front (Frandsen 1997; Huang et al. 2015). In smouldering combustion, peat particles undergo endothermic pyrolysis, forming char, also known as regime I, followed by exothermic oxidation reactions where char is converted to ash, regime II (Hadden et al. 2013; Huang et al. 2015). The energy released during the exothermal oxidations is transferred to the surrounding environment, some being radiated to the atmosphere and some conducted to the peat particles ahead of the smouldering front. If the combustion of peat particles in the smouldering front produces sufficient energy to overcome the heat losses to the surroundings, the smouldering front spreads away from the ignition point and becomes an independent self-sustained front (Ohlemiller 1985). A smouldering front can then propagate into the peat both vertically and horizontally. However, it is the front propagating horizontally that is primarily responsible for the large areas of peat consumed, as vertical propagation is generally extinguished by deeper layers of wet peat (Wein 1983; Miyanishi and Johnson 2002; Usup et al. 2004). The propagation mechanisms of smouldering fires in peats are complex and further research is needed to understand how peat conditions affect the dynamics of self-sustained fire propagation.
In this paper, we analyse the horizontal propagation dynamics of smouldering fires moving away from an ignition source under a range of controlled moisture content and bulk density conditions. We use β regressions to estimate the propagation distance as a function of moisture content and bulk density. We also estimate the spread rate of the fire when self-sustained smouldering propagation was observed. Finally, we use a linear model to relate the properties of the peat to the spread rate of smouldering fires. The purpose of this experimental research is to enable key peat conditions (moisture content and bulk density) that influence smouldering propagation to be understood.
Materials and methods
Experimental set-up
Laboratory smouldering experiments were designed to control environmental and peat conditions. Commercial milled peat (Shamrock Irish Moss Peat, Bord Na Móna, Newbridge, Republic of Ireland) was used to be consistent with previous studies (Belcher et al. 2010; Hadden et al. 2013) and because commercially milled peat reduces extraneous sources of variation due to its homogeneous properties (Frandsen 1987, 1991; Zaccone et al. 2014; Prat et al. 2015). The peat was placed in a 22 × 18 × 6-cm insulated burnbox made of fibreboard with a thermal conductivity of 0.07–0.11 W m–1 K–1, similar to that of peat (Frandsen 1987, 1991; Benscoter et al. 2011; Garlough and Keyes 2011). Peats were oven-dried at 80°C for 48 h. Water was added to the dry peat until the required MC was achieved. The moist peat was sealed in a plastic bag for 24 h before the experiment to allow equilibration. The prepared peats had 25, 100, 150, 200 and 250% MC. This range of moisture contents represents peat conditions that are susceptible to smouldering ignition (Frandsen 1987; Rein et al. 2008; Benscoter et al. 2011).
A range of peat bulk densities (ρ, dry mass of peat per unit volume of wet peat) was included in our experimental data. Two bulk density treatments (BD1, BD2) were created for each moisture content: (1) the peat was spread into the burnbox until it filled the volume (BD1); and (2) the peat was compressed into the burnbox until it filled the volume (BD2). This second treatment increased bulk density by reducing the bulk volume and the air spaces inside the sample. The range of ρ obtained was representative of peat and duff from boreal and temperate peatlands (Frandsen 1997; Benscoter et al. 2011; Wellock et al. 2011; Davies et al. 2013; Thompson and Waddington 2014).
An electric igniter coil was situated along one side of the burnbox and used to ignite a 2-cm-wide section of dry peat (~0% MC). The coil delivered 100 W for 30 min, similar to the heat provided by surface burning vegetation (Rein et al. 2008). This ignition protocol was sufficient to start a smouldering front in the dry peat section, which then attempted to spread to the adjacent peat sample. An infrared camera (ThermaCAM SC640, FLIR Systems, Wilsonville, OR) was used to image the radiative energy flux from the smouldering peat surface (Prat-Guitart et al. 2015). The position of the smouldering front was identified using the infrared images, which provided information at a resolution of 0.05 × 0.05 cm (one pixel). The camera took images every minute, creating sequences of between 300 and 700 images for each burn test. Experiments for each combination of MC and bulk density treatment were replicated four times. Moisture evaporation from the peat was negligible in the absence of a smouldering front (Table S1 in the supplementary material, available online). We therefore assumed that moisture content of unburnt peat well ahead of the smouldering front was constant throughout the duration of the burning experiments.
Self-propagation distance of peat fires
Once the fire self-extinguished, we recorded the final distance (D) of the smouldering front from the igniter. A value between 0 and 1 indicated the fraction (y) of peat consumed along a transect across the width of the burnbox at distance D from the igniter. These fractions were transformed to avoid zeros and ones by yD = (y(N – 1) + 1/2)/N, where N is the sample size (Smithson and Verkuilen 2006). Beta regressions were used to estimate the association of yD with the peat bulk density ρ (kg m–3) and moisture content MC with a logit link function for the expectation of yD given by:

where βDs are the regression coefficients. A total of seven β regressions were fitted for values of D at 6, 8, 10, 12, 14, 16 and 18 cm. Each regression was a different analysis to avoid autocorrelation of residuals. A β regression can be viewed as a flexible form of logistic regression that allows for a continuous response variable (modelled by β distribution) and skew in the response distribution (modelled by the precision parameter of β distribution) (Cribari-Neto and Zeileis 2010). Similarly to our β regressions, logistic regressions were used in past studies with success or failure data to estimate the probability of peat ignition and early propagation 10 cm away from the ignition region (Frandsen 1997; Lawson et al. 1997; Reardon et al. 2007).
Image processing
The infrared images were corrected for the distortion caused by the angle of the infrared camera. The burnbox surface area was represented by ~150 000 pixels, each of them giving information about the dynamics of the smouldering front during the experiment. For every pixel, we built a profile of the radiated energy flux throughout the duration of the burn (Prat-Guitart et al. 2015). The radiative energy flux increased when an approaching smouldering front heated the area, indicating that the peat was being dried before the start of the combustion processes, pyrolysis and oxidation. The start of the smouldering combustion (tL) was defined as the time a pixel’s radiative energy flux reached at a rate of 10 W m–2 min–1 or more. For every experiment, we obtained a matrix of tL giving the time when the leading edge of the smouldering front reached each pixel.
As a method to prevent boundary effects from the burnbox edges to the smouldering front, 2 cm of pixels close to the sides were removed from each image. The pixels from the 6 cm closest to the igniter were also excluded to avoid effects of the ignition heating coil. The area of pixels left, ~60% of the burnbox surface, was used for the subsequent image analysis and estimation of the spread rates. Image processing was undertaken using Matlab and the Image Processing Toolbox (version R2012b 8.0.0.783, MathWorks Inc., Natick, MA).
Estimation of horizontal spread rates
For each burn, we split the tL matrix into subregions of 2 × 2 cm. We then estimated the spread rate and direction of spread for each subregion by fitting a generalised least-squares model, assuming a linear smouldering front across the subregion. This approach allows all the data within a subregion to inform our estimates of spread rate and direction. The fitted model is:
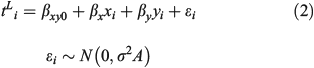
where x and y are the position of the ith pixel within a subregion. The coefficients βx and βy give the rate at which tLi increases per unit increase in x and y respectively; ϵi is the error term assumed to be normal-distributed with mean zero and with variance–covariance matrix σ2A. The spatial correlation structure of A was described with a Gaussian semivariogram (Pinheiro and Bates 2000). The model was fitted using a maximum likelihood. The spread rate of the leading front in the x direction was then estimated as:

where S is the subregion spread rate and Δx is the length of a pixel (typically 0.05 cm). A spread rate was estimated for each subregion of the burnbox and then a median spread rate () and median absolute deviation were estimated for each experimental burn.
We looked for detectable changes in spread rate during the long burns (burns lasting more than 7 h). We tested the constancy of the smouldering spread rate away from the igniter (x direction) across the entire burnbox by regressing the median time taken for the smouldering front to reach a pixel against linear and quadratic terms in the distance from the igniter (see supplementary material). The quadratic term is expected to be zero if spread rate is constant. For each treatment, the significance of the quadratic term was tested using the F test.
Effect of moisture content and bulk density on the spread rate
The effects of MC and ρ on were examined using a linear model. Even though the bulk density of the peat was based on a compression treatment (BD1 and BD2), we took bulk density to be a continuous variable. The two explanatory variables were standardised (by subtracting the mean and dividing by the standard deviation). Spread rates were log-transformed so that model residuals were close to normality. Forward stepwise model selection was used to arrive at a best-fit model that minimised the Akaike information criterion, AIC (Burnham and Anderson 2002). Only the model with the lowest AIC is reported in the results (Eqn 4):

where is the median spread rate of each burn k, β0, β1, β2 and β3 are the coefficients of the dependent parameters and ϵk is the residuals assumed to be normally distributed. The data analyses were done with R project statistical software (version 3.0.2, R Core Team 2013), the betareg package (Cribari-Neto and Zeileis 2010) the ape package (Paradis et al. 2004) and the nlme package (Pinheiro et al. 2015).
Results
The milled peats used had an intrinsic bulk density between 50 to 150 kg m–3 (Fig. 1). Each MC treatment had a range of bulk densities. Peats with low moisture content tended to have higher bulk densities than peats with high moisture content (Spearman correlation –0.4, P value 0.02).
![]() |
The smouldering front always self-propagated across the entire box (20 cm) when the moisture content was 25 or 100% (Fig. 2). At these moisture contents, the smouldering fire was observed to propagate as a single linear front. The smouldering front always self-extinguished before reaching the end of the burnbox in peats of 200 and 250% MC. The fronts that self-extinguished were irregular for the last 1–2 cm of propagation. Peats with 150% MC had intermediate behaviour, with fronts self-extinguishing in 75% of the experimental burns (Fig. 2a). Peats with 200 and 250% MC did not self-sustain propagation in cases with high bulk density. Only peats with 100% MC (low and high bulk density) and peats with 150% MC and low bulk density sustained smouldering for more than 7 h. For these long burns, we found no evidence that the spread rate was changing across the burnbox, as indicated by the non-significant quadratic term for each of the peat conditions (F tests for peats with 100% MC and low bulk density F1,48 = 1.2, P = 0.28; 100% and high bulk density F1,49 = 2.9, P = 0.09; and 150% MC F1,31 = 2.0, P = 0.17).
![]() |
Expected self-propagation distances from an ignition source
Peats at low moisture content were more likely to sustain smouldering propagation for a longer distance independently of the peat density (Fig. 3). For example, at D = 12 cm, peats with 25 and 100% MC had an expected fraction of peat burnt (PyD) of 0.72. At short distances (between 6 and 10 cm from the ignition region), PyD was associated with both the moisture content and the bulk density of the peat (Table 1), whereas PyD at longer distances (≥12 cm from an ignition area) were mainly controlled by the moisture content of the peat (Table 1, D = 12 cm, Fig. S1).
![]() |
![]() |
Effect of peat condition on the smouldering spread rates
The spread rates estimated per subregion, S, ranged between 0.6 and 9.1 cm h–1 (Table 2). Owing to self-extinction of the fire, experimental burns with moisture contents of 150, 200, 250% MC had a lower number of subregions where S could be estimated.
The best-fit model is shown in Table 3. The spread rates, , were well explained by the model (R2 = 0.77). There was a significant effect of MC and ρ on the spread rates of smouldering fires, where the continuous increase of MC had a stronger effect on the spread rates than the increase of ρ (Fig. 4). The interaction term was also significant, indicating that for low MC, the change in ρ had a small impact on the spread rates. However, the decrease of spread rates due to the increase of ρ was stronger with higher MC (e.g. –0.015 ± 0.005 cm kg–1 m–3 h–1 for peats with 25% MC and –0.022 ± 0.009 cm kg–1 m–3 h–1 for peats with 100% MC).
![]() |
![]() |
Discussion
Our results indicate that peat moisture content is the main factor predicting the self-sustained propagation of peat fires. High peat bulk density increases the effect of moisture content on the dynamics of smouldering propagation. Peats ≤100% MC had a 70% probability of self-sustaining propagation beyond the initial 12 cm (PyD ≥ 0.72). Under these conditions, oxidation reactions along the smouldering fronts produced sufficient energy to overcome heat losses, dry the peat and ensure self-sustained propagation (Benscoter et al. 2011; Huang and Rein 2015). Even though the front propagated for 20 cm in all bulk densities tested (Fig. 2), the spread rates were significantly slower when bulk density was high (Table 2).
Peats above 150% MC had a high probability of extinction after propagating through 12 cm of peat. This suggests that when the moisture content is higher than 150%, the amount of energy required to evaporate water ahead of the smouldering front is too high for propagation to be self-sustained for more than 12 cm. For distances of ≥12 cm, we found no effect of bulk density on propagation (Table 1). This could be because (i) the bulk density does not affect the fraction of peat burnt at D ≥ 12 cm, suggesting that moisture content of the peat is the main predictor of PyD, or (ii) there is an effect of bulk density on PyD when D ≥ 12 cm but our data have limited power to detect this effect. To increase the power to detect effects of bulk density, future research should consider a larger sample size and greater variety of moisture content and bulk density treatments within the range tested.
The estimated PyD smouldering propagation for distances up to 10 cm from an ignition source is comparable with the probability of ignition and early propagation estimated in previous studies on natural peat soils (Frandsen 1997; Lawson et al. 1997; Reardon et al. 2007). In those studies, the 50% probability of ignition and 10-cm propagation had a moisture content threshold of 120% MC for Sphagnum and feather moss peats with bulk densities between 20 and 60 kg m–3 and mineral contents below 30% (mass of mineral content per total mass of dry peat) (Frandsen 1997). In our analysis, peats below 160% MC and similar bulk densities have a PyD = 0.5 at D = 12 cm, indicating that there is a 50% probability of self-sustained smouldering for more than 12 cm (Fig. 2). However, denser peats with 130 kg m–3 have a PyD = 0.5 at D = 10 cm only when the peat moisture content is below 113% MC (Fig. 3). Using milled peats, Frandsen (1987) established a comparable threshold for peat ignition and early propagation of 110% MC and bulk density of 130 kg m–3.
Compared with peats with low bulk density, the peats with high bulk density produce more energy owing to the oxidation of a greater mass of peat particles (Ohlemiller 1985). However, the modification of bulk density through compression implies that high-bulk-density peats hold a larger mass of water per unit volume. For a successful self-propagation, all this water needs to be evaporated by the energy released from the adjacent smouldering front. Frandsen (1991) suggested that the rate of mass consumption is not sensitive to the bulk density of the peat. In that sense, the energy required to keep on-going self-sustained smouldering propagation should be proportional to the mass of peat being consumed. We found that the spread rate of the smouldering front is sensitive to the bulk density of the peat and the effect depends on the moisture content of the peat (Table 3). For example, the spread rate in peats with high bulk density and low moisture contents (i.e. 25, 100% MC) is not affected as much as in peats with high moisture contents (i.e. 150–250% MC). Peats with high moisture content and high bulk density have a reduced rate of O2 diffusion and a larger amount of water to be evaporated before combustion. These conditions cause slower spread rates and shorter propagation distances (Ohlemiller 2002; Belcher et al. 2010; Hadden et al. 2013). The effect of oxygen availability to the smouldering reaction zone was not considered in Frandsen (1991), as a constant oxygen flow was supplied through the burning peat to avoid the extinction of the fire.
The spread rate of the smouldering fronts was analysed for the first time as a function of peat conditions. The effects of moisture content and bulk density on spread rates are consistent with the estimates of energy required to dry and heat the peat (estimated energy required to start thermal decomposition of peat for each peat moisture content and bulk density treatment is shown in Fig. S2 and Fig. S3). A greater mass of water per unit volume requires more energy to evaporate and start combustion (Fig. S2). However, peats with 100% MC and bulk density below 100 kg m–3 have a higher energy demand and the fire propagated more slowly than in peats with 150 and 200% MC and bulk density below 75 kg m–3 (Fig. S3). For a given moisture content, there is more energy needed to carry on smouldering combustion when the bulk density increases (Fig. S3). Increasing peat’s bulk density, there is a larger energy production during the oxidation of the larger mass of peat. However, this energy produced is less than the energy necessary to evaporate the water in the peat. As a consequence, the spread rate of the fire is slower or not self-sustained (Fig. 4).
Controlled smouldering tests
It should be noted that our experiments were at a laboratory scale and peat conditions were controlled. Therefore, caution should be taken when using our results at the field scale. The peat conditions (i.e. bulk density, mineral content, peat composition) can be very heterogeneous in real ecosystems (McMahon et al. 1980). Our laboratory-scale experiments intentionally removed these sources of variation. This allowed us to focus on the effect of two important peat conditions (moisture content and the bulk density) on the smouldering propagation dynamics.
Our burnbox size was designed to be suitable for the study of horizontal propagation across greater distances than in previous studies (Frandsen 1987; Frandsen 1997; Reardon et al. 2007), enhancing our understanding of propagation in larger sample sizes. The duration of our experiment and the size were limited by a maximum burn duration of 12 h in order to minimise the effect of diurnal variation in ambient temperature and humidity. The spread rates and the expected fractions of peat burnt were both estimated assuming constant moisture content and bulk density throughout the duration of an experiment. During our experiments, there were no moisture content changes that could have a substantial effect on the smouldering fire propagation (Table S1). However, substantial changes of moisture content or bulk density during the experiment duration could cause variation in the estimated spread rates with the distance.
The ignition of the peat along one side of the burnbox enabled a linear propagation of smouldering fronts moving perpendicularly to the igniter coil. This ignition method was developed to estimate spread rates from infrared images that assume linear propagation (Prat-Guitart et al. 2015). A depth of only 5 cm of peat was used in the present study to focus solely on horizontal smouldering propagation, avoiding vertical spread of the smouldering front and limiting the multidimensional spread of a peat fire. Previous experimental studies have examined peat ignition in deeper samples (Rein et al. 2008; Benscoter et al. 2011). However, deeper peat samples had smouldering fronts propagating horizontally and vertically, making the study of propagation dynamics more complex. The properties of the burnbox material created similar thermal insulation as if the peat sample were surrounded by more peat (Frandsen 1987, 1991; Benscoter et al. 2011; Garlough and Keyes 2011). In these insulated conditions, a sample depth of 5 cm has a small impact on our results and they can be compared with other experiments looking at horizontal propagation in bigger samples.
Application to peatland fires
In this study, the smouldering dynamics were studied in an area of 22 × 18 cm with homogeneous moisture content conditions, comparable with the size of a dry patch of peat moss (Petrone et al. 2004). In peatlands, the moisture content of the surface peat layers is regulated by the distribution of moss species and the position of the water table (Thompson and Waddington 2013b; Waddington et al. 2015). A heterogeneous distribution of Sphagnum mosses is likely to cause a heterogeneous spatial distribution of peat moisture content, creating patches of 20–50-cm diameter (Benscoter and Wieder 2003; Petrone et al. 2004). During drought, the surface layer dries owing to lack of rain, which may then be followed by a lowering of the water table (Chivers et al. 2009; Sherwood et al. 2013; Kettridge et al. 2015). In such circumstances, dry peats in the surface layers have less than 250% MC (Benscoter et al. 2011; Terrier et al. 2014; Lukenbach et al. 2015), thus being vulnerable to peat fires.
After a peat fire, the new surface layer is closer to the water table and consequently has a reduced fire danger. Previous studies suggested that peat fires are common in peatland ecosystem cycles (Turetsky et al. 2002). The consumption of surface layers of peat reduces the accumulation of organic material, allowing Sphagnum mosses to access the water table, being less dependent on external water inputs (Benscoter and Vitt 2008). Post-fire surfaces also enable the roots of vegetation to take up ground water and nutrients from deep mineral layers.
In peatlands, peat bulk density strongly depends on the vegetation cover and temporal changes in the water table behaviour (Davies et al. 2013; Sherwood et al. 2013; Thompson and Waddington 2013a). Deep peat layers often have a higher degree of decomposition and a higher bulk density compared with surface layers (Benscoter et al. 2011; Thompson and Waddington 2014). Following turf-cutting in drained peatlands, new dense and dry layers of bare peat become exposed at the surface and are vulnerable to peat fires.
Peats with 25% MC were included in the analysis to have a representation of very dry peats in our sample. However, such dry peats are uncommon in natural peatlands (Terrier et al. 2014; Lukenbach et al. 2015), being restricted to the surface of drained peatlands under extreme drought. In the present study, bulk density was experimentally manipulated using two peat compression treatments, which produced a range of bulk densities. Dry peats (25% MC) were only experimentally tested with high bulk densities between 108 and 145 kg m–3. The high bulk density of 25% MC peats is due in part to the structure of milled peats and the low expansion of peat particles when a small quantity of water is added to the peat sample (Huang and Rein 2015). The reduced expansion of the dry peat (25% MC) compared with the greater expansion of relatively wetter peat (≥100% MC) caused the negative collinearity between moisture content and bulk density. If we exclude peats with 25% MC, we find no collinearity between MC and ρ (Spearman correlation –0.07, P value 0.7). Therefore, the negative collinearity between MC and ρ (Fig. 1) is caused by the peats with 25% MC. This collinearity could contribute to the interaction reported in the spread rate model (Table 3) and affect extrapolated predictions of spread rates (Dormann et al. 2013). The same spread rate model but excluding peats with 25% MC had similar β0, β1 (MC) and β2 (ρ) coefficients but no significant interaction term. Therefore, the main effects of moisture content and bulk density on the spread rates are qualitatively not affected by the collinearity.
All the milled peats used in the present study had a low mineral content of less than 5%. Natural peats are characterised as having less than 20–35% mineral content (Turetsky et al. 2015) and often <6% (Benscoter et al. 2011). Previous studies have suggested that large quantities of mineral content could reduce the probability of peat ignition and posterior fire propagation (Frandsen 1987; Hungerford et al. 1995). Our peats had an intrinsic mineral content of 2.6 ± 0.2%, similar to the 3.7% of Frandsen’s (1987) peats. This implies that our low-mineral-content peats would give an upper limit on the spread rates and propagation distance. However, small quantities of certain minerals such as salts of calcium or magnesium, common in plant material and soil, have been shown to have no effect on propagation (Benscoter et al. 2011) or rather enhance heat conduction in the fuel media that could help the smouldering propagate faster (Frandsen 1998; Reardon et al. 2007).
Differences in bulk density can be associated with other properties of peat soils such as soil structure, particle size, pore space and decomposition (Ingram 1978). The variation of these physicochemical properties can also affect the energy produced during peat oxidation and the energy transferred through peat particles (Reardon et al. 2007; Huang et al. 2015). The presence of artefacts (e.g. roots, stones) may also play a role in creating variability in peat conditions that could affect the propagation of smouldering fires. Twigs and roots, for example, have been reported to promote the propagation of smouldering fires (Miyanishi and Johnson 2002; Davies et al. 2013); this is likely a result of local changes to MC around the root.
The hydrology of peatlands as well as peat properties should be carefully observed in order to estimate variations in moisture and bulk density as we have shown that these peat conditions strongly influence the propagation of smouldering fires even on a fine scale. The spatial variability and dynamics of peat conditions remains a challenge to studies of peat fires in the field (McMahon et al. 1980; Hungerford et al. 1995) and highlights why laboratory-scale studies are required to understand measured effects on smouldering. The control of individual properties such as moisture content and bulk density can then be used to piece together the broader relationship between peat conditions and smouldering in the natural environment. Milled peats like those used here have been the most utilised alternative to reduce the variability of natural peats and study the influence of external factors (moisture, mineral content, bulk density, oxygen availability, etc.) on smouldering combustion of peat (Frandsen 1987, 1991; Belcher et al. 2010; Hadden et al. 2013; Zaccone et al. 2014; Prat et al. 2015).
Conclusions
This study has built on previous work on ignition and early horizontal propagation of smouldering fires in peats. We coupled laboratory-scale observations of smouldering fires with statistical models to estimate and analyse the fire spread rate and the expected fraction of peat burnt at distance longer than 12 cm. Our findings enable an understanding of the effects of a variety of peat moisture content and bulk density conditions on smouldering propagation dynamics. Self-sustained fronts were observed to propagate in peats with moisture content below 150% MC. The bulk density of the peat was also found to affect the propagation of smouldering fires. The increase of bulk density enhances the effects of moisture content on the propagation dynamics.
Our approaches highlighted that laboratory-scale experimental research can contribute to the theoretical insights of the behaviour of smouldering fires. Data from this study are fundamental to integrate a wide range of realistic peat conditions and their associated horizontal and vertical dynamics to modelling approaches at larger scales.
Acknowledgements
The authors thank the School of Mechanical Engineering at University College Dublin, especially David Timoney for support, John Gahan for his help during laboratory set up and Xinyan Huang for valuable discussions. This work was carried out as part of the Earth and Natural Sciences Doctoral Studies Programme, which was funded by the Higher Education Authority (HEA), and is co-funded by the European Regional Development Fund (ERDF). Claire M. Belcher acknowledges a European Research Council Starter Grant ERC-2013-StG-335891-ECOFLAM.
References
Belcher CM, Yearsley JM, Hadden RM, McElwain JC, Rein G (2010) Baseline intrinsic flammability of Earth’s ecosystems estimated from paleoatmospheric oxygen over the past 350 million years. Proceedings of the National Academy of Sciences 107, 22448–22453.| Baseline intrinsic flammability of Earth’s ecosystems estimated from paleoatmospheric oxygen over the past 350 million years.Crossref | GoogleScholarGoogle Scholar | 1:CAS:528:DC%2BC3MXkslSksg%3D%3D&md5=9e16f6b4906b930f6e6b6cc82bcf9713CAS |
Benscoter BW, Vitt DH (2008) Spatial patterns and temporal trajectories of the bog ground layer along a post-fire chronosequence. Ecosystems 11, 1054–1064.
| Spatial patterns and temporal trajectories of the bog ground layer along a post-fire chronosequence.Crossref | GoogleScholarGoogle Scholar |
Benscoter BW, Wieder RK (2003) Variability in organic matter lost by combustion in a boreal bog during the 2001 Chisholm fire. Canadian Journal of Forest Research 33, 2509–2513.
| Variability in organic matter lost by combustion in a boreal bog during the 2001 Chisholm fire.Crossref | GoogleScholarGoogle Scholar |
Benscoter BW, Thompson DK, Waddington JM, Flannigan MD, Wotton BM, de Groot WJ, Turetsky MR (2011) Interactive effects of vegetation, soil moisture and bulk density on depth of burning of thick organic soils. International Journal of Wildland Fire 20, 418–429.
| Interactive effects of vegetation, soil moisture and bulk density on depth of burning of thick organic soils.Crossref | GoogleScholarGoogle Scholar | 1:CAS:528:DC%2BC3MXlsFGlsLo%3D&md5=bb9dc7530f05c7164513f9764ff90128CAS |
Burnham KP, Anderson DR (Eds) (2002) ‘Model selection and multimodel inference, 2nd edn’ (Springer: New York).
Chivers MR, Turetsky MR, Waddington JM, Harden JW, McGuire AD (2009) Effects of experimental water table and temperature manipulations on ecosystem CO2 fluxes in an Alaskan rich fen. Ecosystems 12, 1329–1342.
| Effects of experimental water table and temperature manipulations on ecosystem CO2 fluxes in an Alaskan rich fen.Crossref | GoogleScholarGoogle Scholar | 1:CAS:528:DC%2BD1MXhsFyku73L&md5=7ee1401f89cdf4e945810ce2481eb94bCAS |
Cribari-Neto F, Zeileis A (2010) Beta regression in R. Journal of Statistical Software 34, 1–24.
| Beta regression in R.Crossref | GoogleScholarGoogle Scholar |
Davies GM, Gray A, Rein G, Legg CJ (2013) Peat consumption and carbon loss due to smouldering wildfire in a temperate peatland. Forest Ecology and Management 308, 169–177.
| Peat consumption and carbon loss due to smouldering wildfire in a temperate peatland.Crossref | GoogleScholarGoogle Scholar |
Dormann C, Elith J, Bacher S, Buchmann C, Carl G, Carre G, Garcia-Marquez JR, Gruber B, Lafourcade B, Leitao PJ, Munkemuller T, McClean C, Osborne PE, Reineking B, Schroder B, Skidmore AK, Zurell D, Lautenbach S (2013) Collinearity: a review of methods to deal with it and a simulation study evaluating their performance. Ecography 36, 27–46.
| Collinearity: a review of methods to deal with it and a simulation study evaluating their performance.Crossref | GoogleScholarGoogle Scholar |
Frandsen WH (1987) The influence of moisture and mineral soil on the combustion limits of smouldering forest duff. Canadian Journal of Forest Research 17, 1540–1544.
| The influence of moisture and mineral soil on the combustion limits of smouldering forest duff.Crossref | GoogleScholarGoogle Scholar |
Frandsen WH (1991) Burning rate of smouldering peat Northwest Science 65, 166–172.
Frandsen WH (1997) Ignition probability of organic soils. Canadian Journal of Forest Research 27, 1471–1477.
| Ignition probability of organic soils.Crossref | GoogleScholarGoogle Scholar |
Frandsen WH (1998) Heat flow measurements from smoldering porous fuel. International Journal of Wildland Fire 8, 137–145.
| Heat flow measurements from smoldering porous fuel.Crossref | GoogleScholarGoogle Scholar |
Garlough EC, Keyes CR (2011) Influences of moisture content, mineral content and bulk density on smouldering combustion of ponderosa pine duff mounds. International Journal of Wildland Fire 20, 589–596.
| Influences of moisture content, mineral content and bulk density on smouldering combustion of ponderosa pine duff mounds.Crossref | GoogleScholarGoogle Scholar |
Hadden RM (2011) Smouldering and self-sustaining reactions in solids : an experimental approach. PhD thesis, University of Edinburgh, UK.
Hadden RM, Rein G, Belcher CM (2013) Study of the competing chemical reactions in the initiation and spread of smouldering combustion in peat. Proceedings of the Combustion Institute 34, 2547–2553.
| Study of the competing chemical reactions in the initiation and spread of smouldering combustion in peat.Crossref | GoogleScholarGoogle Scholar | 1:CAS:528:DC%2BC2cXhvV2ntbY%3D&md5=da028100e0c21709cc3c744450831705CAS |
Huang X, Rein G (2014) Smouldering combustion of peat: inverse modelling of the drying and the thermal and decomposition kinetics. Combustion and Flame 161, 1633–1644.
| Smouldering combustion of peat: inverse modelling of the drying and the thermal and decomposition kinetics.Crossref | GoogleScholarGoogle Scholar | 1:CAS:528:DC%2BC2cXht1Cjs7Y%3D&md5=e7864a5a79d8c1e0b1c78da98ab6794eCAS |
Huang X, Rein G (2015) Computational study of critical moisture content and depth of burn in peat fires. International Journal of Wildland Fire 24, 798–808.
| Computational study of critical moisture content and depth of burn in peat fires.Crossref | GoogleScholarGoogle Scholar |
Huang X, Rein G, Chen H (2015) Computational smoldering combustion: predicting the roles of moisture and inert contents in peat wildfires. Proceedings of the Combustion Institute 35, 2673–2681.
| Computational smoldering combustion: predicting the roles of moisture and inert contents in peat wildfires.Crossref | GoogleScholarGoogle Scholar | 1:CAS:528:DC%2BC2cXhtFSrurzJ&md5=85887a318f8e07bb526b6999a25fa14aCAS |
Hungerford R, Frandsen WH, Ryan KC (1995) Ignition and burning characteristics of organic soils. In ‘Fire in wetlands: a management perspective. Proceedings of the tall timbers fire ecology conference, no. 19’, 3–6 November 1993, Tallahassee, FL. (Eds SI Cerulean, RT Engstrom) pp. 78–91. (Tallahassee, FL)
Ingram H (1978) Soil layers in mires: function and terminology. Journal of Soil Science 29, 224–227.
| Soil layers in mires: function and terminology.Crossref | GoogleScholarGoogle Scholar |
Kettridge N, Turetsky MR, Sherwood JH, Thompson DK, Miller CA, Benscoter BW, Flannigan MD, Wotton BM, Waddington JM (2015) Moderate drop in water table increases peatland vulnerability to post-fire regime shift. Scientific Reports 5, 8063
| Moderate drop in water table increases peatland vulnerability to post-fire regime shift.Crossref | GoogleScholarGoogle Scholar | 1:CAS:528:DC%2BC2MXosVKmu7w%3D&md5=2541728f0227f11c994cba084023d8b4CAS | 25623290PubMed |
Kreye JK, Varner JM, Dugaw CJ, Cao J, Szecsei J, Engber EA (2013) Pine cones facilitate ignition of forest floor duff. Canadian Journal of Forest Research 43, 512–516.
| Pine cones facilitate ignition of forest floor duff.Crossref | GoogleScholarGoogle Scholar |
Lawson BD, Frandsen WH, Hawkes BC, Dalrymple GN (1997) Probability of sustained smouldering ignition for some boreal forest duff types. Natural Resources Canada, Canadian Forest Service, Northern Forestry Centre, Forest Management Note 63. (Edmonton, AB)
Lukenbach MC, Hokanson KJ, Moore PA, Devito KJ, Kettridge N, Thompson DK, Wotton BM, Petrone RM, Waddington JM (2015) Hydrological controls on deep burning in a northern forested peatland. Hydrological Processes 29, 4114–4124.
| Hydrological controls on deep burning in a northern forested peatland.Crossref | GoogleScholarGoogle Scholar |
McMahon C, Wade D, Tsoukalas S (1980) Combustion characteristics and emissions from burning organic soils. In ‘Proceedings of the 73rd annual meeting of the Air Pollution Control Association’, 22–27 June 1980, Montreal, QC. Air Pollution Control Association, Paper No. 15.5. (Pittsburgh, PA)
Miyanishi K, Johnson EA (2002) Process and patterns of duff consumption in the mixedwood boreal forest. Canadian Journal of Forest Research 32, 1285–1295.
| Process and patterns of duff consumption in the mixedwood boreal forest.Crossref | GoogleScholarGoogle Scholar |
Ohlemiller TJ (1985) Modeling of smoudering combustion propagation. Progress in Energy and Combustion Science 11, 277–310.
| Modeling of smoudering combustion propagation.Crossref | GoogleScholarGoogle Scholar | 1:CAS:528:DyaL28XhsFWrtbY%3D&md5=8e5d3e8d446f025e09432ab85ba8f6c3CAS |
Ohlemiller TJ (2002) Smoldering combustion. In ‘SFPE handbook of fire protection engineering, 3rd edn’. (Eds PJ DiNeeno, D Drysdale, CL Beyler, WD Walton) pp. 200–210. (National Fire Protection Association: Quincy, MA).
Page S, Siegert F, Rieley JO, Boehm HV, Jayak A, Limink S (2002) The amount of carbon released from peat and forest fires in Indonesia during 1997. Nature 420, 61–65.
| The amount of carbon released from peat and forest fires in Indonesia during 1997.Crossref | GoogleScholarGoogle Scholar | 1:CAS:528:DC%2BD38XosVCmu78%3D&md5=3f089b4c40fe826c65676bd34c6bf1c3CAS | 12422213PubMed |
Paradis E, Claude J, Strimmer K (2004) APE: analyses of phylogenetics and evolution in R language. Bioinformatics 20, 289–290.
| APE: analyses of phylogenetics and evolution in R language.Crossref | GoogleScholarGoogle Scholar | 1:CAS:528:DC%2BD2cXms1eitg%3D%3D&md5=4e2a86462dfa26af2984d16e09cd8178CAS | 14734327PubMed |
Petrone RM, Price JS, Carey SK, Waddington JM (2004) Statistical characterization of the spatial variability of soil moisture in a cutover peatland. Hydrological Processes 18, 41–52.
| Statistical characterization of the spatial variability of soil moisture in a cutover peatland.Crossref | GoogleScholarGoogle Scholar |
Pinheiro J, Bates D (2000) Mixed-effects models in S and S-PLUS. (Springer: New York, NY)
Pinheiro J, Bates D, DebRoy S, Sarkar D, R Core Team (2015) nlme: linear and nonlinear mixed effects models. R package version 3.1–122. Available at http://CRAN.R-project.org/package=nlme [Verified 25 January 2016]
Prat N, Belcher CM, Hadden RM, Rein G, Yearsley JM (2015) A laboratory study of the effect of moisture content on the spread of smouldering in peat fires. Flamma 6, 35–38.
Prat-Guitart N, Valor T, Krivtsov V (2011) Post-fire effects in an organic peat soil in Scotland. In ‘Treballs de la Societat Catalana de Geografia, Vol. 71–72’. (Ed. X Ubeda) pp. 93–114. (IEC: Barcelona).
Prat-Guitart N, Rein G, Belcher CM, Hadden RM, Yearlsey JM (2015) Infrared analysis as a tool for studying the dynamics of horizontal smouldering propagation in laboratory peat fires. In ‘Coal and peat fires, a global perspective, peat – geology, combustion and case studies’. (Eds GB Stracher, A Prakash, G Rein) pp. 121–139. (Elsevier: Amsterdam)
R Core Team (2013) ‘R: A language and environment for statistical computing.’ (R Foundation for Statistical Computing: Vienna, Austria)
Reardon AJ, Hungerford R, Ryan KC (2007) Factors affecting sustained smouldering in organic soils from pocosin and pond pine woodland wetlands. International Journal of Wildland Fire 16, 107–118.
| Factors affecting sustained smouldering in organic soils from pocosin and pond pine woodland wetlands.Crossref | GoogleScholarGoogle Scholar |
Rein G (2013) Smouldering fires and natural fuels. In ‘ Fire phenomena in the Earth system. An interdisciplinary approach to fire science’. (Ed CM Belcher) pp. 15–34. (Wiley–Blackwell: London)
Rein G, Cleaver N, Ashton C, Pironi P, Torero JL (2008) The severity of smouldering peat fires and damage to the forest soil. Catena 74, 304–309.
| The severity of smouldering peat fires and damage to the forest soil.Crossref | GoogleScholarGoogle Scholar |
Sherwood JH, Kettridge N, Thompson DK, Morris PJ, Silins U, Waddington JM (2013) Effect of drainage and wildfire on peat hydrophysical properties. Hydrological Processes 27, 1866–1874.
| Effect of drainage and wildfire on peat hydrophysical properties.Crossref | GoogleScholarGoogle Scholar |
Shetler G, Turetsky MR, Kane ES, Kasischke ES (2008) Sphagnum mosses limit total carbon consumption during fire in Alaskan black spruce forests. Canadian Journal of Forest Research 38, 2328–2336.
| Sphagnum mosses limit total carbon consumption during fire in Alaskan black spruce forests.Crossref | GoogleScholarGoogle Scholar | 1:CAS:528:DC%2BD1cXptFynt7o%3D&md5=e09af89026f8ff3020de202191a5c0ecCAS |
Smithson M, Verkuilen J (2006) A better lemon squeezer? Maximum-likelihood regression with beta-distributed dependent variables. Psychological Methods 11, 54–71.
| A better lemon squeezer? Maximum-likelihood regression with beta-distributed dependent variables.Crossref | GoogleScholarGoogle Scholar | 16594767PubMed |
Terrier A, Groot WJ, Girardin MP (2014) Dynamics of moisture content in spruce–feather moss and spruce–sphagnum organic layers during an extreme fire season and implications for future depths of burn in Clay Belt black spruce forests. International Journal of Wildland Fire 23, 490–502.
| Dynamics of moisture content in spruce–feather moss and spruce–sphagnum organic layers during an extreme fire season and implications for future depths of burn in Clay Belt black spruce forests.Crossref | GoogleScholarGoogle Scholar |
Thompson DK, Waddington JM (2013a) Peat properties and water retention in boreal forested peatlands subject to wildfire. Water Resources Research 49, 3651–3658.
| Peat properties and water retention in boreal forested peatlands subject to wildfire.Crossref | GoogleScholarGoogle Scholar |
Thompson DK, Waddington JM (2013b) Wildfire effects on vadose zone hydrology in forested boreal peatland microforms. Journal of Hydrology 486, 48–56.
| Wildfire effects on vadose zone hydrology in forested boreal peatland microforms.Crossref | GoogleScholarGoogle Scholar |
Thompson DK, Waddington JM (2014) A Markov chain method for simulating bulk density profiles in boreal peatlands. Geoderma 232–234, 123–129.
| A Markov chain method for simulating bulk density profiles in boreal peatlands.Crossref | GoogleScholarGoogle Scholar |
Turetsky MR, Wieder K, Halsey L, Vitt D (2002) Current disturbance and the diminishing peatland carbon sink. Geophysical Research Letters 29,
| Current disturbance and the diminishing peatland carbon sink.Crossref | GoogleScholarGoogle Scholar | 1:CAS:528:DC%2BD38XotFehsLk%3D&md5=f76e422cb4b81285a71ac5a98dea4395CAS |
Turetsky MR, Benscoter B, Page S, Rein G, van der Werf GR, Watts A (2015) Global vulnerability of peatlands to fire and carbon loss. Nature Geoscience 8, 11–14.
| Global vulnerability of peatlands to fire and carbon loss.Crossref | GoogleScholarGoogle Scholar | 1:CAS:528:DC%2BC2cXitFKltbbE&md5=cb641b4b2f0b0da63eaaad5827f951a0CAS |
Usup A, Ashimoto YH, Akahashi HT, Ayasaka HH (2004) Combustion and thermal characteristics of peat fire in tropical peatland in central Kalimantan, Indonesia. Tropics 14, 1–19.
| Combustion and thermal characteristics of peat fire in tropical peatland in central Kalimantan, Indonesia.Crossref | GoogleScholarGoogle Scholar |
Van Wagner CE (1972) Duff consumption by fire in eastern pine stands. Canadian Journal of Forest Research 2, 34–39.
| Duff consumption by fire in eastern pine stands.Crossref | GoogleScholarGoogle Scholar |
Waddington JM, Morris PJ, Kettridge N, Granath G, Thompson DK, Moore PA (2015) Hydrological feedbacks in northern peatlands. Ecohydrology 8, 113–127.
| Hydrological feedbacks in northern peatlands.Crossref | GoogleScholarGoogle Scholar |
Wein RW (1983) Fire behaviour and ecological effects in organic terrain. In ‘The role of fire in northern circumpolar ecosystems’. (Eds RW Wein and DA MacLean) pp. 81–95. (John Wiley & Sons Ltd: New York).
Wellock ML, Reidy B, Laperle CM, Bolger T, Kiely G (2011) Soil organic carbon stocks of afforested peatlands in Ireland. Forestry 84, 441–451.
| Soil organic carbon stocks of afforested peatlands in Ireland.Crossref | GoogleScholarGoogle Scholar |
Zaccone C, Rein G, D’Orazio V, Hadden RM, Belcher CM, Miano TM (2014) Smouldering fire signatures in peat and their implications for palaeoenvironmental reconstructions. Geochimica et Cosmochimica Acta 137, 134–146.
| Smouldering fire signatures in peat and their implications for palaeoenvironmental reconstructions.Crossref | GoogleScholarGoogle Scholar | 1:CAS:528:DC%2BC2cXps12nsrs%3D&md5=b10203a5020dd66657feb3bb54e6c23fCAS |