Climate change, sea-level rise and the Gippsland shoreline
Kathleen L. McInnes
A
Abstract
Global warming due to the accumulation of heat-trapping greenhouse gases in the atmosphere is driving changes in the climate system that will have serious implications for global coastlines. Sea-level rise is a major concern for low-lying coastal communities. Oceans and ice caps have long response times to global warming, and coastal environments are increasingly likely to experience hazards such as coastal inundation and erosion over the coming decades. Sea levels will not increase uniformly due to a range of physical processes. In the vicinity of the Gippsland coastline, sea-level rise will be slightly higher than the global-averaged sea-level rise because of the influence of the East Australian Current on regional sea levels. Global warming is also driving tropical expansion and a southward shift in the major climate and weather systems of the planet. Associated with these changes is an enhancement of wind speeds in the westerly wind belt which is causing changes in the wave climate of the Southern Ocean. This, in turn, may influence the coastline of southern Australia. The warming of the oceans and atmosphere is intensifying severe weather systems through greater wind speeds and rainfall totals. These various factors will cause global to local scale changes, with a potentially compounding effect on coastal hazards as a result of changes in local sea levels, wind and wave climate, severe weather systems and extreme sea levels in the Gippsland Lakes region.
Keywords: climate change, erosion, inundation, sea-level acceleration, sea-level extremes, sea-level rise, storm surge, subsidence, wave climate.
Sea-level trends and causes of sea-level rise
Global mean sea level has risen by approximately 25 cm since 1880 (Bureau of Meteorology and CSIRO 2022). Sea levels are measured at the coast by a network of coastal tide gauges and over the broader ocean surface by satellite altimeters. Global-averaged trends from these sources over the period 2007–2018 have been 3.6 ± 1.7 and 3.8 ± 0.3 mm year−1 respectively and have exhibited accelerating trends over the period 1993–2018 of 0.063 ± 0.120 and 0.053 ± 0.026 mm year−2 respectively at rates that are consistent with those associated with the mid to high emission scenarios considered by the Intergovernmental Panel on Climate Change (IPCC) (Wang et al. 2021). In Australia, the rate of sea-level rise based on Australian gauges from the Australian National Collection of Homogenized Observations of Relative Sea Level (ANCHORS) dataset over 1966–2019 is 1.94 mm year−1 and over 1993–2019 is 3.74 mm year−1 (Hague et al. 2022), again indicating that sea-level rise is accelerating.
The main cause of sea-level rise is global warming. This is causing warming of the atmosphere and oceans and in turn the melting of land-based ice and snow in glaciers and ice sheets, which then flows to the sea. In addition, the warming oceans expand and contribute to a vertical rise in sea level.
Sea-level rise due to climate processes is not uniform. Variations in wind stresses, freshwater and heat fluxes cause spatial differences in ocean density due to temperature (the thermosteric component), salinity (the halosteric component) and ocean circulation, which in turn affect sea levels. Variations in atmospheric pressure also influence sea levels with climatological high- and low-pressure centres causing regional depression or elevation of sea levels. The various processes associated with sea-level rise and extremes are illustrated in Fig. 1.
Climate-sensitive processes and components that can influence global and regional sea levels. The term ‘ocean properties’ refers to ocean temperature, salinity and density, which influence and are dependent on ocean circulation (SLE, sea-level equivalent; mCDW, modified Circumpolar Deep Water; GIA, glacial isostatic adjustment). White arrows indicate ocean circulation. Pinning points indicate where the grounding line is most stable and ice-sheet retreat will slow (source: Fox-Kemper et al. 2021).
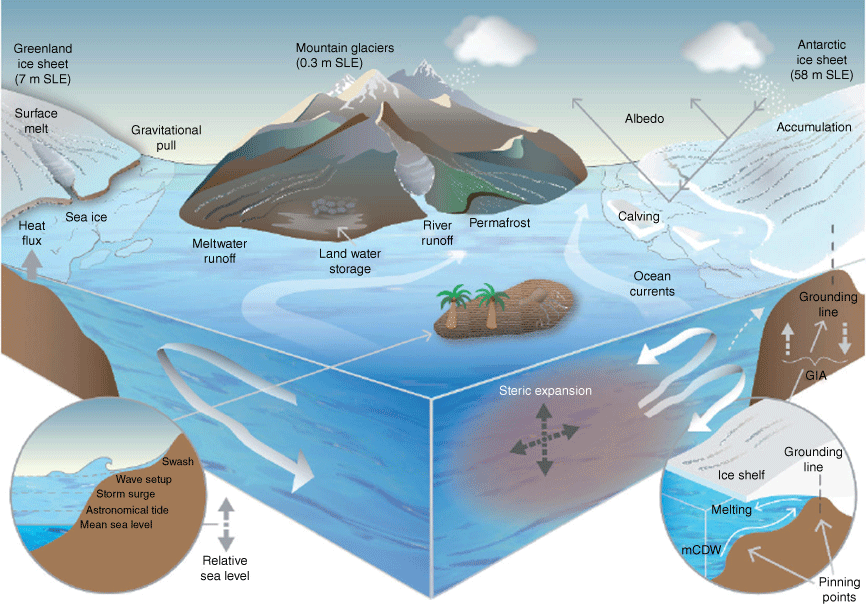
Emission scenarios and climate change
The starting point for projecting future climates using climate models is a set of scenarios about future anthropogenic greenhouse gas and aerosol emissions. These are linked to socio-economic pathways because factors such as future population growth, energy generation, geopolitics and globalisation ultimately determine the amount of greenhouse gases and aerosols that will be emitted.
Emission scenarios have varied across IPCC reports. In recent years Shared Socioeconomic Pathways (SSPs) have been developed to describe how global population, society and economics may change in the future. Table 1 presents the range of global temperature increase and the 5–95% uncertainty range associated with these scenarios. The scenarios SSP1-1.9 and SSP1-2.6 are broadly linked to the Paris Targets of limiting global-averaged warming to 1.5 and 2.0°C above pre-industrial levels by 2100. It is important to note that current commitments to greenhouse gas emissions reductions will see global temperatures increase by approximately 3.2 [2.2–3.5]°C (5–95% range) by 2100 (IPCC 2023).
Scenario | Global-averaged temperature increase (°C by 2081–2100 relative to 1995–2014) | Global-averaged sea-level rise (m in 2100 relative to 1995–2014) | Global-averaged sea-level rise (m in 2150 relative to 1995–2014) | Global-averaged rate of sea-level rise (mm year−1 over 2080–2100) | Sea-level rise projection for East Gippsland (m in 2100 relative to 1995–2014) | |
---|---|---|---|---|---|---|
SSP1-1.9 | 0.7 (0.2–1.5) | 0.38 (0.28–0.55) | 0.57 (0.37–0.86) | 4.2 (2.4–6.6) | 0.39 [0.24–0.58] | |
SSP1-2.6 | 1.2 (0.6–2.0) | 0.44 (0.32–0.62) | 0.68 (0.46–0.99) | 5.2 (3.2–8.0) | 0.44 (0.29–0.64) | |
SSP2-4.5 | 2.0 (1.4–3.0) | 0.56 (0.44–0.76) | 0.92 (0.66–1.33) | 7.7 (5.2–11.6) | 0.59 (0.43–0.82) | |
SSP3-7.0 | 3.1 (2.2–4.7) | 0.68 (0.55–0.90) | 0.92 (0.66–1.33) | 10.4 (7.4–14.8) | 0.74 (0.57–0.99) | |
SSP5-8.5 | 4.0 (2.7–5.7) | 0.77 (0.63–1.01) | 1.98 (0.98–4.82) | 12.1 (8.6–17.6 | 0.84 (0.65–1.11) | |
SSP5-8.5A | 4.0 (2.7–5.7) | 0.88 (0.63–1.60) | 1.98 (0.98–4.82) | 15.8 (8.6–30.1) | 0.96 (0.65–1.70) |
Sources: IPCC table 4.2 in Lee et al. (2021), table 9.9 in Fox-Kemper et al. (2021). Column six contains projections for 2100 for the East Gippsland location marked in Fig. 2d.
Sea-level projections
Sea-level projections for 2100 from the IPCC in its Sixth Assessment (AR6) report are provided in Table 1 for the years 2100 and 2150. The processes included in the projections are assessed by the IPCC to be of ‘medium confidence’ and include changes due to thermal expansion, the mass balance of glaciers and ice sheets, and terrestrial water storage. The IPCC also provide scenarios they assess to have ‘low confidence’ of occurring on the time scales considered, due to poorly understood ice sheet dynamical processes that could lead to their more rapid disintegration (DeConto and Pollard 2016; Fox-Kemper et al. 2021) (these are provided in the final row of Table 1). The provision of the ‘low confidence’ projections is for risk-averse decision-makers who wish to assess the implications of possible, though less likely, high-end sea-level rise scenarios.
Because of the slow response times of the oceans to greenhouse gas emissions, even limiting global-average increases in temperature to those stipulated by the Paris Targets will only slow but will not stop sea levels from rising. Another important consideration is the rate of sea-level rise (see the fifth column of Table 1). Limiting global warming will keep rates of rise to more manageable levels only slightly higher than those currently being measured, whereas the higher warming scenarios (SSP3-7.0 and SSP5-8.5) could see yearly rates of rise of over 10 mm year−1, which will challenge or overwhelm the capacity of some natural and human systems to adapt. Higher rates of warming could also trigger more rapid disintegration of the Antarctic ice sheet leading to much larger rates of sea-level rise. There remains high uncertainty about how and when this climate tipping point will be crossed (Armstrong McKay et al. 2022), which would cause such processes to come into play. This remains a priority research area.
Locally, sea-level rise may vary markedly from global mean sea-level rise values. Factors that can cause localised departures include local ocean dynamics such as density and circulation, spatial redistribution of sea level due to gravitational, rotational and deformational changes in the Earth in response to ice-sheet mass changes (sometimes referred to as sea-level fingerprints) and vertical land movement (VLM), which affects relative sea-level rise. Fig. 2 shows the projected SLR for 2080–2100 relative to 1995–2014 under SSP5-8.5. The processing of these fields is based on Fox-Kemper et al. (2021) but differs slightly in that the VLM term includes only the glacial isostatic adjustment (GIA) contribution and not localised sources of VLM. It illustrates how the three components of ocean dynamics, fingerprints and GIA vary in terms of their contribution to projected sea-level rise for Australia. Sea-level rise projections for the East Gippsland coast at 2100 are also provided in column six of Table 1 (see Fig. 2d for location). Compared to the global-averaged values for 2100 in column three, values in East Gippsland are up to 0.1 m higher.
Projected regional sea-level change (unit: cm) for the Australian region over 2080–2100 relative to 1995–2014 under SSP5-8.5 for (a) sterodynamic sea level (including global mean thermal expansion), (b) sum of various regional sea-level contributions due to changes of land-ice mass and groundwater, (c) regional sea level due to GIA-only VLM as used by the IPCC AR5, and (d) total sea level including all global and regional contributions in (a–c). The ‘+’ in (d) marks the location for the sea-level projections provided in column six of Table 1.
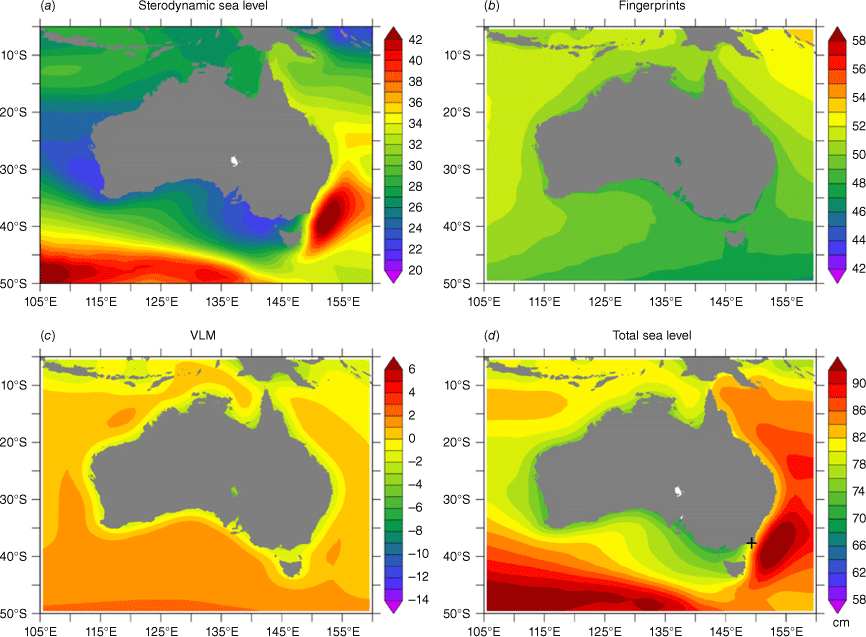
Fig. 2a indicates that higher sea levels are projected along the southeastern coast of Australia, due to the strengthening of the East Australian Current with values up to 6 cm higher than the global-averaged value of 30 cm. This contributes to large spatial gradients in the amount of projected sea-level rise along the Gippsland coastline. The so-called ‘Fingerprint’ pattern in Fig. 2b relates to the redistribution of sea level across the global oceans due to mass loss from glaciers, and the ice sheets of Antarctica and Greenland. As mass is lost from these sources, the gravitational exertion on the surrounding oceans weakens causing a redistribution of the ocean surface so that the sea-level rise around the ice sheets is reduced and that in far-field (such as the tropical Pacific) is enhanced.
VLM, i.e. subsidence or uplift, affects the relative sea-level rise experienced at the coast and is an important consideration in coastal adaptation. Various factors may contribute locally to VLM. On a global scale, the most widespread contribution to VLM is from GIA, a steady land motion arising from the loss of the continental ice sheets after the last Ice Age. The contribution of GIA to relative sea-level rise is illustrated in Fig. 2c. Combining these three sources of spatial variation in sea-level rise allows the global mean sea-level rise projections in Table 1 to be converted to local values (Fig. 2d).
Separate from GIA discussed above, other sources of VLM may occur in coastal settings. Tectonic activity, sediment settling due to groundwater or hydrocarbon extraction, sediment compaction and terrestrial loading because of changes in rainfall over land can all contribute to VLM (Hammond et al. 2021). Parts of East Gippsland are known to be experiencing subsidence due to mining, irrigation and offshore oil and natural gas extraction (Ng et al. 2015). Using global positioning system (GPS) data Riddell et al. (2020) and Hammond et al. (2021) find subsidence rates in the region of 0–2 mm year−1. Ng et al. (2015) used interferometric synthetic aperture radar (InSAR) data to map VLM across the Gippsland Basin. Their results indicate areas of vertical displacement across the region that are within ±10 mm year−1. Subsiding coastal areas were found along the Lakes Entrance coastline.
Changes in climate circulation and weather patterns
In addition to mean sea-level rise, temporary weather-driven increases to sea levels occur as a result of storm surges and waves. These extreme sea-level events can cause erosion and temporary coastal inundation. In East Gippsland, eastward travelling frontal systems are typically responsible for causing storm surges (McInnes et al. 2013; Colberg et al. 2019) and east coast depressions are a major contributor to extreme waves (O’Grady and McInnes 2010; O’Grady et al. 2015).
The location and behaviour of these weather systems are expected to change due to the observed poleward expansion of the tropics and poleward shift of mid-latitude weather systems caused by anthropogenic climate change (e.g. Cai and Cowan 2013; Pepler et al. 2018; Yang et al. 2020). This change is associated with a southward extension of the subtropical ridge and a southward contraction of the band of westerlies in which frontal systems are embedded.
Climate change will also cause changes to the rainfall of severe storms. The moisture-holding capacity of the atmosphere is related to atmospheric temperature, increasing at a rate of ~7% per degree warming (Fowler et al. 2021). A general pattern of more intense peak precipitation and weaker precipitation during less intense times has been found in Australian storms at higher temperatures, regardless of the climatic region and season (Wasko and Sharma 2015). It is speculated that warming temperatures are invigorating storm dynamics, which could lead to increases in the magnitude and frequency of short-duration floods (Wasko and Sharma 2015).
Projections of extreme sea levels and waves
Storm surges are caused by the falling atmospheric pressure and onshore wind-induced piling up of water at the coast. Storm surges in combination with astronomical tides are referred to as storm tides and can cause coastal inundation. Extreme coastal sea levels are enhanced by wave-breaking processes that cause wave setup along the open coastline (O’Grady et al. 2019). These processes are illustrated in the left circle of Fig. 1.
Hydrodynamic modelling studies that consider future climate conditions, including the ongoing tropical expansion, indicate there would be slight reductions in storm surge heights along the southern Australian coastline (Colberg and McInnes 2012; Vousdoukas et al. 2018; Colberg et al. 2019) due to the southward migration of the storm systems. A weak declining trend in the frequency of deep cyclone days associated with East Coast Lows has also been observed (Pepler and Dowdy 2021).
Ocean wave characteristics such as height, direction and period are projected to change as a result of global-warming-induced changes in atmospheric circulation patterns (Morim et al. 2019). Along Australia’s southern coastline, wave period is projected to increase by around 5% by 2080–2100 relative to 1979–2004 and become more southerly. Along the Gippsland coast, a decline of 10% on average over the same period is projected (Morim et al. 2019). Over the Southern Ocean, the 1-in-100 year wave height is projected to increase by 5–15% by the end of the 21st century (2081–2100), compared with the 1979–2005 period (Meucci et al. 2020).
Sea-level rise and changes in weather patterns may cause coastal erosion of sandy shorelines and estuaries. For example, O’Grady et al. (2015) found that the southward shift in weather patterns predicted in global climate models resulted in an increase in westward wind-driven currents and wave forcing in summer which could influence sediment transport. In southeastern Australia, analysis of trends in a 31-year record of shoreline position found that there is a strong geologic control on shoreline erosion, and changes in shoreline position (both erosion and accretion) are relatively localised and mainly due to factors such as shore protection structures and other engineered modifications to the coast. The largest changes to date have been found near the entrances of large tidal inlets (Konlechner et al. 2020).
Summary
The Gippsland Lakes are facing a number of challenges posed by climate change. Foremost is the issue of sea-level rise that will increasingly impact the low-lying estuarine lagoon system in the decades to come. Sea levels have risen 25 cm since 1880 and are accelerating at a rate consistent with the mid- to high-emission scenarios considered by the IPCC. Projections of sea-level rise are not spatially uniform due to the effects of local ocean dynamics, gravitational, rotational and deformational changes in the Earth in response to ice-sheet mass changes, and glacial isostatic adjustment. Sea-level rise along the eastern seaboard will be higher than the global average due to the strengthening of the East Australian Current. In addition to this, parts of coastal East Gippsland are experiencing subsidence because of oil and gas mining and groundwater pumping, and assessment of these additional contributions to relative sea-level rise will be important to quantify for coastal adaptation. An important consideration in relation to warming targets is that although ongoing sea-level rise is inevitable on centennial timescales from emissions and warming that have already occurred, the amount of sea-level rise and the yearly rates of rise vary considerably between the different scenarios and will influence the adaptive capacity of natural and human systems in the coastal zone.
Sea-level rise will exacerbate the coastal impacts of severe weather events by increasing the inland reach of storm surges and waves and therefore the associated inundation and erosion hazards. Changes in circulation systems will also influence severe storm tracks, intensities and frequencies of associated oceanic hazards such as waves, storm surges, inundation and erosion. The effects of these changes are less certain, requiring further research to understand the extent of the large-scale changes and their local impacts. Finally, it is worth emphasising that sea-level rise, changes to the ocean extreme events and the rainfall associated with the atmospheric storms that cause them have the potential to compound the associated impacts in the coastal zone, and require consideration in planning and adaptation responses.
Data availability
Data reproduced in this paper can be sourced from IPCC reports at https://www.ipcc.ch/report/ar6/wg1/.
Declaration of funding
The writing of this review was supported by the CSIRO and the National Environmental Science Program through the Earth Systems Science Hub.
Acknowledgements
The author acknowledges Dr Xuebin Zhang for the production of Fig. 2 and SLR values in column six of Table 1.
References
Armstrong McKay DI, Staal A, Abrams JF, Winkelmann R, Sakschewski B, Loriani S, Fetzer I, Cornell SE, Rockström J, Lenton TM (2022) Exceeding 1.5°C global warming could trigger multiple climate tipping points. Science 377(6611), eabn7950.
| Crossref | Google Scholar | PubMed |
Bureau of Meteorology and CSIRO (2022) State of the Climate 2022. 28 pp. Available at https://www.csiro.au/en/research/environmental-impacts/climate-change/state-of-the-climate
Cai W, Cowan T (2013) Southeast Australia autumn rainfall reduction: a climate-change-induced poleward shift of ocean–atmosphere circulation. Journal of Climate 26(1), 189-205.
| Crossref | Google Scholar |
Colberg F, McInnes KL (2012) The impact of future changes in weather patterns on extreme sea levels over southern Australia. Journal of Geophysical Research: Oceans 117(C8), C08001.
| Crossref | Google Scholar |
Colberg F, McInnes KL, O’Grady J, Hoeke R (2019) Atmospheric circulation changes and their impact on extreme sea levels around Australia. Natural Hazards and Earth System Sciences 19(5), 1067-1086.
| Crossref | Google Scholar |
DeConto RM, Pollard D (2016) Contribution of Antarctica to past and future sea-level rise. Nature 531, 591-597.
| Crossref | Google Scholar | PubMed |
Fowler HJ, Lenderink G, Prein AF, et al. (2021) Anthropogenic intensification of short-duration rainfall extremes. Nature Reviews Earth & Environment 2(2), 107-122.
| Crossref | Google Scholar |
Fox-Kemper B, Hewitt HT, Xiao C, Aðalgeirsdóttir G, Drijfhout SS, Edwards TL, Golledge NR, Hemer M, Kopp RE, Krinner G, Mix A, Notz D, Nowicki S, Nurhati IS, Ruiz L, Sallée J-B, Slangen ABA, Yu Y (2021) Ocean, Cryosphere and Sea Level Change. In ‘Climate Change 2021: The Physical Science Basis. Contribution of Working Group I to the Sixth Assessment Report of the Intergovernmental Panel on Climate Change’. (Eds V Masson-Delmotte, P Zhai, A Pirani, SL Connors, C Péan, S Berger, N Caud, Y Chen, L Goldfarb, MI Gomis, M Huang, K Leitzell, E Lonnoy, JBR Matthews, TK Maycock, T Waterfield, O Yelekçi, R Yu, B Zhou) pp. 1211–1362. (Cambridge University Press, Cambridge, United Kingdom and New York, NY, USA). doi:10.1017/9781009157896.011
Hague BS, Jones DA, Trewin B, Jakob D, Murphy BF, Martin DJ, Braganza K (2022) ANCHORS: A multi-decadal tide gauge dataset to monitor Australian relative sea level changes. Geoscience Data Journal 9(2), 256-272.
| Crossref | Google Scholar |
Hammond WC, Blewitt G, Kreemer C, Nerem RS (2021) GPS imaging of global vertical land motion for studies of sea level rise. Journal of Geophysical Research: Solid Earth 126(7), e2021JB022355.
| Crossref | Google Scholar |
IPCC (2023) Summary for Policymakers. In ‘Climate Change 2023: Synthesis Report. A Report of the Intergovernmental Panel on Climate Change. Contribution of Working Groups I, II and III to the Sixth Assessment Report of the Intergovernmental Panel on Climate Change’. (Eds Core Writing Team, H Lee, J Romero). 34 p. (IPCC: Geneva, Switzerland) doi:10.59327/IPCC/AR6-9789291691647.001
Konlechner TM, Kennedy DM, O’Grady JJ, Leach C, Ranasinghe R, Carvalho RC, Luijendijk AP, McInnes KL, Ierodiaconou D (2020) Mapping spatial variability in shoreline change hotspots from satellite data; a case study in southeast Australia. Estuarine, Coastal and Shelf Science 246, 107018.
| Crossref | Google Scholar |
Lee J-Y, Marotzke J, Bala G, Cao L, Corti S, Dunne JP, Engelbrecht F, Fischer E, Fyfe JC, Jones C, Maycock A, Mutemi J, Ndiaye O, Panickal S, Zhou T (2021) Future Global Climate: Scenario-Based Projections and Near- Term Information. In ‘Climate Change 2021: The Physical Science Basis. Contribution of Working Group I to the Sixth Assessment Report of the Intergovernmental Panel on Climate Change’. (Eds V Masson-Delmotte, P Zhai, A Pirani, SL Connors, C Péan, S Berger, N Caud, Y Chen, L Goldfarb, MI Gomis, M Huang, K Leitzell, E Lonnoy, JBR Matthews, TK Maycock, T Waterfield, O Yelekçi, R Yu, B Zhou) pp. 553–672. (Cambridge University Press, Cambridge: UK and New York, NY, USA) 10.1017/9781009157896.006
McInnes KL, Macadam I, Hubbert G, O’Grady J (2013) An assessment of current and future vulnerability to coastal inundation due to sea-level extremes in Victoria, southeast Australia. International Journal of Climatology 33(1), 33-47.
| Crossref | Google Scholar |
Meucci A, Young IR, Hemer M, Kirezci E, Ranasinghe R (2020) Projected 21st century changes in extreme wind-wave events. Science Advances 6(24), eaaz7295.
| Crossref | Google Scholar | PubMed |
Morim J, Hemer M, Wang XL, et al. (2019) Robustness and uncertainties in global multivariate wind-wave climate projections. Nature Climate Change 9(9), 711-718.
| Crossref | Google Scholar |
Ng AH-M, Ge L, Li X (2015) Assessments of land subsidence in the Gippsland Basin of Australia using ALOS PALSAR data. Remote Sensing of Environment 159, 86-101.
| Crossref | Google Scholar |
O’Grady JG, McInnes KL (2010) Extreme wind waves and their relationship to storm surges in northeastern Bass Strait. Australian Meteorology and Oceanography Journal 60, 265-275.
| Google Scholar |
O’Grady JG, McInnes KL, Colberg F, Hemer MA, Babanin AV (2015) Longshore wind, waves and currents: climate and climate projections at Ninety Mile Beach, southeastern Australia. International Journal of Climatology 35, 4079-4093.
| Crossref | Google Scholar |
O’Grady JO, McInnes KL, Hemer MA, Hoeke RK, Stephenson A, Colberg F (2019) Extreme water levels for Australian beaches using empirical equations for shoreline wave setup. Journal of Geophysical Research-Oceans 124, 5468-5484.
| Crossref | Google Scholar |
Pepler A, Dowdy A (2021) Intense east coast lows and associated rainfall in eastern Australia. Journal of Southern Hemisphere Earth Systems Science 71(1), 110-122.
| Crossref | Google Scholar |
Pepler A, Ashcroft L, Trewin B (2018) The relationship between the subtropical ridge and Australian temperatures. Journal of Southern Hemisphere Earth Systems Science 68(1), 201-214.
| Crossref | Google Scholar |
Riddell AR, King MA, Watson CS (2020) Present-day vertical land motion of Australia from GPS observations and geophysical models. Journal of Geophysical Research: Solid Earth 125(2), e2019JB018034.
| Crossref | Google Scholar |
Vousdoukas MI, Mentaschi L, Voukouvalas E, Verlaan M, Jevrejeva S, Jackson LP, Feyen L (2018) Global probabilistic projections of extreme sea levels show intensification of coastal flood hazard. Nature Communications 9, 2360.
| Crossref | Google Scholar |
Wang J, Church JA, Zhang X, Gregory JM, Zanna L, Chen X (2021) Evaluation of the local sea-level budget at tide gauges since 1958. Geophysical Research Letters 48(20), e2021GL094502.
| Crossref | Google Scholar |
Wasko C, Sharma A (2015) Steeper temporal distribution of rain intensity at higher temperatures within Australian storms. Nature Geoscience 8(7), 527-529.
| Crossref | Google Scholar |
Yang H, Lohmann G, Lu J, Gowan EJ, Shi X, Liu J, Wang Q (2020) Tropical expansion driven by poleward advancing midlatitude meridional temperature gradients. Journal of Geophysical Research: Atmospheres 125(16), e2020JD033158.
| Crossref | Google Scholar |