Arboreal activity of invasive rodents: conservation implications for the control of an island pest
Alexandra H. Nance



A
B
C
Abstract
Invasive rodents pose a substantial threat to biodiversity and are a leading cause of species decline and extinction on islands. Population suppression using ground-based methods is common practice, though arboreal behaviour of rodents may render control programs less effective.
We aimed to quantify species-specific invasive rodent activity (Rattus rattus, R. exulans, Mus musculus) across three forest strata to determine the prevalence of arboreal rodent behaviour in a system that has undergone extensive long-term rodent baiting, and therefore assess the suitability of solely ground-based baiting in this system.
We calculated rodent presence at the ground, mid-storey, and canopy using three detection methods (camera traps, chew cards and tracking tunnels) deployed for 30-day periods across three structurally distinct forest types (canopy heights ranged from 3.5 to 16.7 m). We developed continuous rodent activity indices for each method, which we paired with density estimates. Strata-specific species composition was determined using camera trap images.
Rodent presence was recorded equally across all strata, with R. rattus dominating above-ground strata. Rodent densities differed significantly between forest types, which was largely consistent with activity indices.
Our findings offer an additional explanation for reduced efficacy of long-term ground-based control programs: arboreal behaviour may exacerbate the reduction in efficacy often associated with long-term control.
Effective management of invasive rodent species on islands is a global conservation challenge. Our findings suggest above-ground control may be required in some long-term suppression programs or eradication campaigns, particularly in the presence of the black rat.
Keywords: black rat, distance sampling, house mouse, invasive species, island conservation, Norfolk Island, Pacific rat, rodent management.
Introduction
Invasive rodents, particularly rats (e.g. Rattus rattus, R. exulans, R. norvegicus) and mice (Mus musculus), are a leading cause of species population decline and extinction worldwide (Bellard et al. 2016; Doherty et al. 2016; Dueñas et al. 2021). These species have this effect due to their ability to rapidly reach high abundance, capacity to occupy a wide range of habitats, predatory behaviour, and omnivorous diets (Harper and Bunbury 2015; Witmer and Shiels 2018). Island ecosystems are particularly susceptible to invasive species given their high rates of vulnerable endemic species compared to mainland ecosystems (Kier et al. 2009; Ruffino et al. 2015). Alarmingly, 47% of the world’s islands that are home to one or more highly threatened vertebrate species also harbour one or more invasive Rattus species (Spatz et al. 2017). The ongoing threat invasive rodents pose to island and global biodiversity is therefore severe, with invasive rodent management a major conservation priority for island ecosystems (Duron et al. 2017).
Two major strategies used by conservation managers to protect biodiversity from invasive rodents are eradication (complete removal of rodents from the system; Howald et al. 2007) or population control (suppressing rodent abundance to reduce impact; Howald et al. 2015; Duron et al. 2017). Eradication programs are highly effective, with success rates of up to 96% on uninhabited islands (Keitt et al. 2015). However, in mainland regions or on islands with resident human populations, long-term control is often favoured over eradication due to the associated logistical and sociological aspects of species management (Engeman et al. 2018). Where rodent suppression is the chosen management strategy, long-term ground-based trapping and baiting programs are commonly used (Brown et al. 2015; Howald et al. 2015; Duron et al. 2017). Although long-term control programs can reduce rodent abundance, they can become less effective with time due to genetic or behavioural resistance to rodenticide, or as the proportion of bait- or trap-shy individuals within a population increases (Allsop et al. 2017; Gronwald and Russell 2022). In such situations, it is valuable to explore alternative strategies to improve rodent control outcomes (Fig. 1).
Conceptual diagram detailing the decision-making process for invasive rodent control once invasive rodents have been identified as a management concern (i.e. having a negative ecological impact on native species). Various scenarios or states need to be assessed (green boxes) to determine which actions (blue and yellow boxes) are required. The yellow box identifies the stage within the decision-making process at which the present study sits.
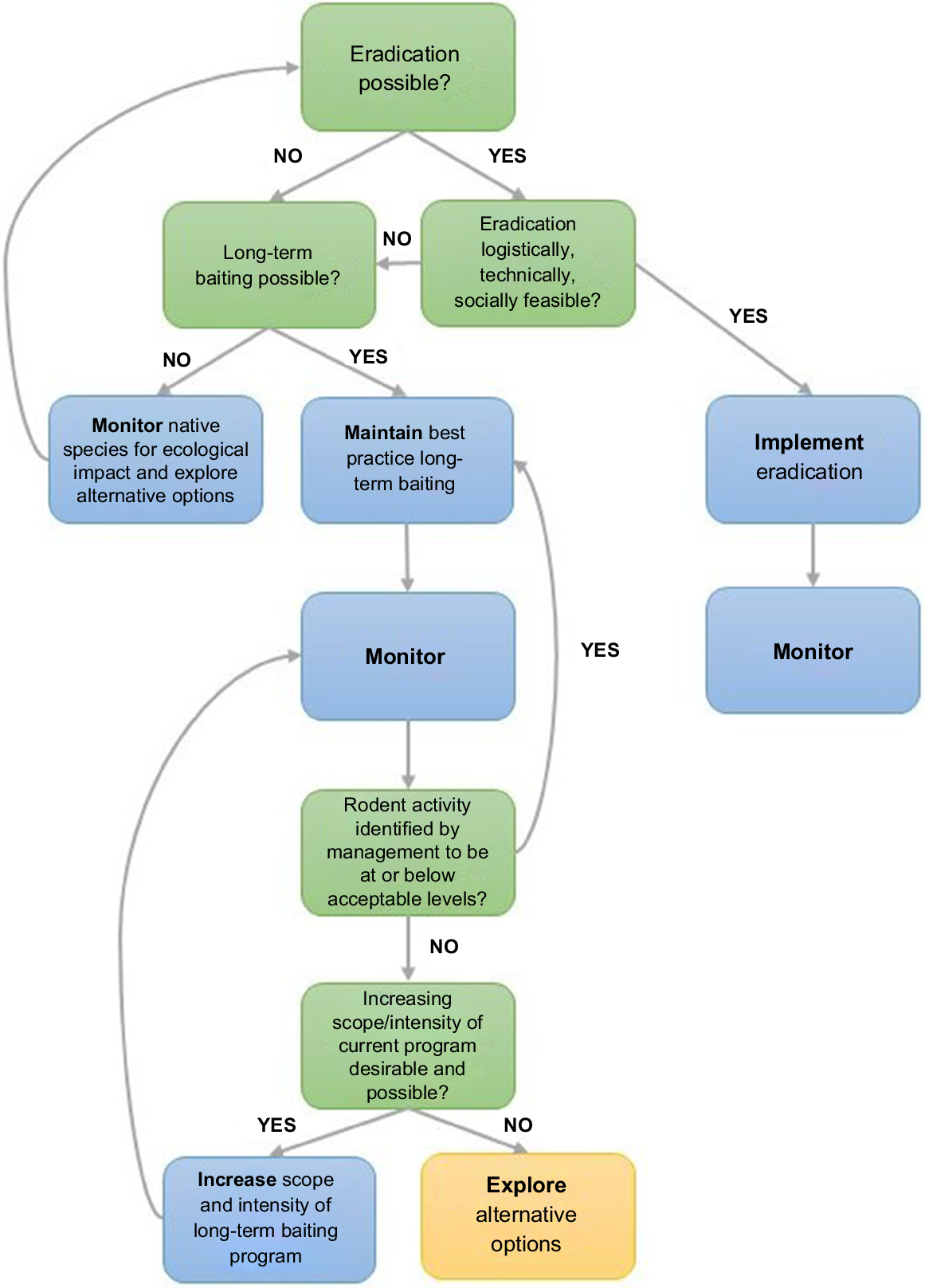
The potential interaction between ground-based control and arboreal behaviour of invasive rodents is an aspect of management that is not often explored despite this behaviour being well documented (see Shiels et al. 2014). Previous research has reported the climbing abilities of Rattus species in simulated environments (Foster et al. 2011) and has demonstrated directly and indirectly that Rattus species prey on arboreal taxa (e.g. Hadfield and Saufler 2009; Smith et al. 2016; Banko et al. 2019; Shiels and Ramírez de Arellano 2019; Nance et al. 2023). Although arboreal rodents may regularly come to ground (Lindsey et al. 1999), their behaviour has raised concerns over the effectiveness of control techniques that are solely ground-based (Nelson et al. 2002; Wegmann et al. 2011). Indeed, arboreal behaviour was considered a factor in the failure of the 2001–2002 rat (R. rattus) eradication attempt on Palmyra Atoll, leading researchers to place bait in the canopy to achieve success during the 2011 operation (Wegmann et al. 2014). This mirrors other studies showing that bait delivered above-ground can increase bait uptake from black rats (Tobin et al. 1997), and decrease uptake from non-target species (Ringler et al. 2021). Ecologically informed rodent management should consider both habitat use and foraging behaviour when placing bait and designing control networks (Krijger et al. 2017). Considering this, a more comprehensive understanding of arboreal rodent behaviour in systems that conduct long-term ground-based control, or those considering an eradication campaign would be beneficial.
In this study, we aimed to determine invasive rodent presence/absence and generate indices of rodent activity across multiple forest strata and forest types in an island system that has been subject to an extensive, long-term ground-baiting program. Understanding arboreal rodent activity in this context has the potential to inform local management strategies. More broadly, such insight is likely to be relevant to systems where long-term ground-based rodent control programs are exhibiting reduced efficacy over time.
Methods
Study system
Norfolk Island, an external Australian territory, is an oceanic island situated in the South Pacific Ocean (29°02′S 167°57′E) with a land area of 34.6 km2 (Geosciences Australia 2021). Due to its geographical isolation (~1100 km from New Zealand and ~1500 km from Australia), this island group is home to many unique species, including 40 endemic plant species, eight endemic land bird species, and >100 known endemic invertebrate species. Fifty-eight species of plants and animals are recognised as threatened (Director of National Parks 2023). Current threats to biodiversity are associated with historical and contemporary human habitation, and include land clearing and degradation, feral and domestic cats (Felis catus), invasive rodents (Rattus rattus, R. exulans and Mus musculus), feral chickens (Gallus gallus) invasive woody and non-woody plants, and climate change (Director of National Parks 2023). Norfolk Island currently supports a resident human population of ~2200 (Australian Bureau of Statistics 2021).
This case study focused on the Norfolk Island National Park (NINP) and the adjoining Botanical Gardens (Fig. 2). Collectively, NINP and the Botanical Gardens protect 465.5 ha of Norfolk Island (Director of National Parks 2023). Plant communities in the park are characterised as sub-tropical rainforest, including native palm-dominated forest, and native hardwood forest (Fig. 2a, b; Director of National Parks 2023). Native palm forest is characterised by the dominance of the endemic Niau palm (Rhopalostylis baueri) and exhibits a high canopy (9–14 m) with an open, structurally simplistic understorey. Native hardwood forest is characterised by the dominance of Norfolk Island pine (Araucaria heterophylla) and hardwood species such as bloodwood (Baloghia inophylla), ironwood (Nestegis apetala), and beech (Myrsine ralstoniae), and exhibits a high canopy (6.5–14 m) with a structurally complex understorey. Exotic red cherry guava (Psidium cattleyanum) is a naturalised and invasive woody species on Norfolk Island and now dominates areas of forest within NINP (Fig. 2c; Director of National Parks 2023). Cherry guava is allelopathic and forms dense thickets that exhibit a lower, more uniform canopy (3.8–11.5 m) than native forests, with little to no understorey (Tng et al. 2016).
Location of 30 sites established to monitor invasive rodent activity in Norfolk Island National Park and adjoining Botanic Gardens (green polygons). Points indicate location of each site shaded by forest type, with typical forest structure of each respective forest type depicted in photographic images: white points represent forest sites dominated by (a) native Niau palm (Rhopalostylis baueri); grey points represent forest sites dominated by (b) Araucaria heterophylla and native hardwood species; black points represent forest sites dominated by (c) invasive red cherry guava (Psidium cattleyanum). Inset shows the location of the National Park relative to the island. Photo credits: A Nance.
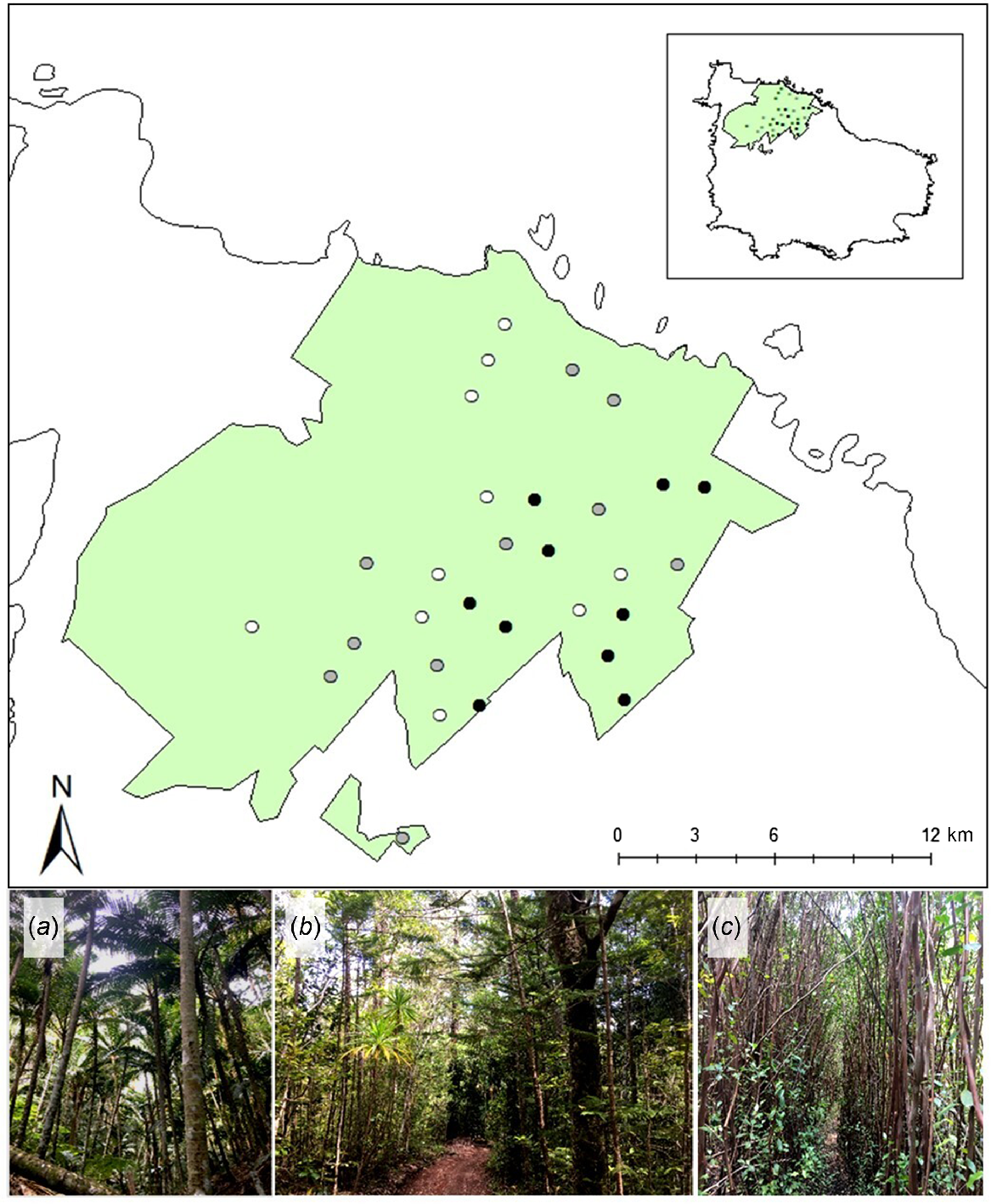
Arboreal behaviour of invasive Rattus species was first documented in NINP in 1988, but is likely to have been prevalent since the arrival of black rats in the 1940s (Robinson 1988). Recent rodent surveys in the park (conducted in 2016) suggested very high levels of arboreality, with >80% of rodent detections made above-ground (R. Clarke, unpublished data). Combined with high levels of rodent activity within the National Park, practitioners and researchers expressed concerns around a solely ground-based baiting strategy for the purposes of long-term control. In line with adaptive management principles (Williams and Brown 2014), this study was conceptualised by practitioners and researchers to address the uncertainty around the extent of arboreal behaviour in the context of long-term ground-based control.
Long-term rodent control
Three species of invasive rodent occur across Norfolk Island: the black rat (R. rattus), Pacific rat (R. exulans), and house mouse (M. musculus; Director of National Parks 2023). Depredation by rodents is listed as a key threatening process in the Norfolk Island National Park, and ground-based rodent control is known to confer some benefit to native species (e.g. Nance et al. 2023). Due to the negative impacts of rodents on native flora and fauna, a ground-based baiting program was initiated in the National Park in 1993 with the objective of suppressing invasive rodents, as opposed to achieving eradication; this has been maintained as a long-term program ever since, and has included regular independent monitoring (Director of National Parks 2023). Since it began, the park has been baited regularly using 1st and 2nd generation rodenticides across a bait station network consisting of at least 900 bait stations. The current baiting network, an expansion of the original, consists of 1800 ground-based bait stations placed at intervals of 50–100 m along bait-lines that bisect the park, and at 25 m intervals around the park boundary (see Supplementary Material). This program utilises multiple forms of first generation rodenticides (diphacinone- or coumatetralyl-based feeding blocks; Zapi SpA, Italy, licenced to Ensystex Australasia Pty Ltd) to reduce the risk of secondary poisoning of the endemic predatory owl (Ninox novaeseelandiae undulata), and also utilises second generation Muskil (combined difenacoum and bromadiolone feeding blocks) outside of the owl breeding season (M. Wilson, pers. comm.). The baits are used on a rotational basis to reduce the likelihood of rodents developing genetic rodenticide resistance. Expert review of the long-term baiting program in 2016 endorsed key elements, including the baiting network size, spatial distribution of bait stations, bait station design, bait products used, baiting frequency, track infrastructure and monitoring protocols (Broome and Corson 2016). Despite this, invasive rodent activity and subsequent predation and herbivory pressure remain at levels that continue to threaten a wide range of endemic plants and animals (Director of National Parks 2023; Nance et al. 2023).
Sampling strategy
We established 30 sites along public walking tracks or non-public service tracks representing three focal forest types: invasive cherry guava (n = 10), native hardwood (n = 10), and native palm forests (n = 10; Fig. 2). There is a paucity of sites in the west and north-west sections of the Park because these areas are dominated by plantations of Eucalyptus sp., and thickets of African olive (Olea europaea; Fig. 2). At each site we established a 100 m transect and a circular buffer (radius = 90 m) from the transect mid-point (2.5 ha area). This buffer area (2.5 ha) corresponded to three times the mean core area occupied by an individual of the largest rodent species present on Norfolk Island (i.e. the ‘core home range’ of R. rattus; Shiels 2010). To ensure sampling independence, the buffer area for each site did not overlap with that of any other site, which equates to a minimum distance of 180 m between site mid-points.
Three monitoring stations were established at the centre of each site (transect mid-point), one for each focal stratum: ground, mid-storey and canopy spaced 5–10 m from any other station (Fig. 3). The mid-storey was defined as approximately half the height of the canopy, as measured by a laser range finder (Opti-Logic, model: 800LH). Each monitoring station comprised three commonly used passive monitoring devices: a motion-triggered infrared camera trap (Reconyx HyperFire HC600), a chew card baited with a non-toxic peanut butter lure (Connovation GC136), and a tracking tunnel with a non-toxic peanut butter lure (Gotcha Traps, Black Trakka standard size) (Blackwell et al. 2002; Sweetapple and Nugent 2011; Rendall et al. 2014). We deployed the three devices at each monitoring station as a cluster, with each device situated within 1 m of each other. A small number of cameras were pointed at another monitoring device, however all cameras were considered to be baited due to their proximity to baited chew cards and tunnels.
Deployment heights of invasive rodent monitoring stations by forest type across 30 sites in Norfolk Island National Park. Mean canopy height for each forest type is represented by the maximum height of trees. Mean deployment heights of monitoring stations per stratum (ground, mid-storey and canopy) are represented by the heights of monitoring station images. Box and whisker plots indicate variation in deployment heights of mid-storey (grey boxes) and canopy (white boxes) stations. All ground stations were deployed on the ground (i.e. 0 m) and did not vary in height. Lines inside boxes indicate median heights, lower and upper box boundaries indicate 25th and 75th percentiles respectively, lower and upper whiskers indicate 95% confidence intervals. Filled circles indicate outliers.
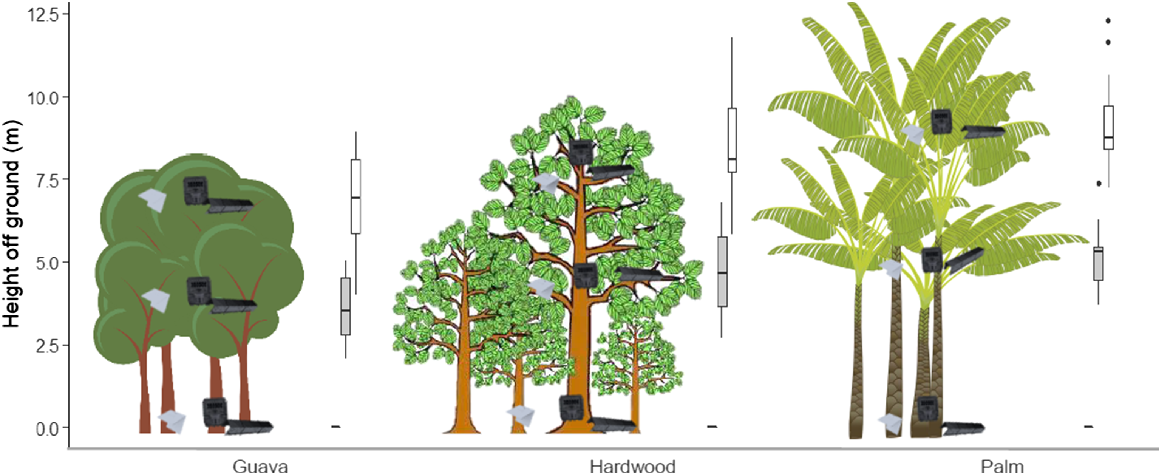
Monitoring equipment was deployed at each station across two seasons: austral spring 2019 (6 November – 14 December) and austral autumn 2020 (27 February – 1 April), resulting in 180 monitoring stations (three stations per site per season). Guava trees began fruiting during the second data collection period (autumn 2020), however fruits largely remained green (and therefore unpalatable to rodents) for the duration of the observation period. Equipment at each station was deployed for a continuous 29- to 30-day period resulting in 5379 trap nights. A handheld infrared thermal scanner (ThermoGEAR G100EX, Nippon Avionics Co., Ltd) was used to conduct two to three night-time surveys per site per season to independently estimate rodent density in the immediate vicinity of all fixed monitoring stations. Monitoring stations were checked opportunistically during thermal surveys (two to three times during the observation period) or after adverse weather (one to two times during the observation period) for compromised cameras, cards or tunnels. Parks staff refreshed bait stations with diphacinone- or coumatetralyl-based feed blocks on a monthly or bi-monthly basis in the months leading up to and during both monitoring seasons as part of routine rodent control activities.
Rodent monitoring
Camera traps (Reconyx Hyperfire HC600) were mounted on tree trunks or branches using cable ties, with the camera lens in the horizontal plane. Due to the clustered format of devices, chew cards or tunnels were visible in the field of vision for a small proportion of cameras (n = 31). To ensure cameras could be triggered by rodent movement we aimed mid-storey and canopy cameras at vegetation structures that rodents could reasonably traverse. Cameras were programmed to take a series of three ‘rapid fire’ photographs (i.e. with no delay between triggers) upon motion detection. Rodent presence was defined as one or more rodent detections made during the month-long observation period. We used a subset of camera trap images to identify between rodent species (see ‘Species Composition’). We removed eight of the 180 cameras from the dataset due to technical malfunction.
Chew cards consist of 9 × 18 cm pieces of 3 mm corflute (Connovation GC136). We filled internal flutes with a 60:40 mixture of smooth peanut butter and water prior to deployment. Chew cards were then nailed flat against a tree trunk or large branch at the beginning of the observation period. Any cards that remained on the nail at the end of the observation period were collected. We determined rodent presence for each chew card based on the presence of rodent chew marks. One chew card was removed from the dataset as a member of the public tampered with it during the observation period (i.e. n = 179). We did not differentiate species based on chew marks.
We used lightweight, rectangular black plastic tunnels (10 × 10 × 50 cm) containing a white cardboard tracking card (Gotcha Traps, Black Trakka standard size). The 50 × 10 cm tracking card consists of a central black inkpad adjoined on either side by two white cardboard panels. A lure is placed at the centre, and as animals move through the tunnel their prints (i.e. presence) are recorded on the white card. Lure consisted of a small amount of store-bought smooth peanut butter placed on the centre of the tracking card before securing it inside the tracking tunnel. Ground-level tunnels were secured using a large metal U-pin, and above-ground tunnels were secured to tree branches using two cable ties. To ensure rodents could reasonably access and pass through above-ground tunnels we attached them to horizontal or near-horizontal branches. At the end of the observation period rodent presence was determined based on the presence of rodent footprints on each tracking card. Five cards were excluded because they were dislodged from their tunnels during the observation period. We did not differentiate species based on foot print markings.
Species were identified exclusively through the use of a subset of camera trap images. Due to high levels of rodent activity (and high insect activity in some instances) a large proportion of chew card and tracking tunnels reached saturation (see results; Burge et al. 2017). Because of this, differentiation between species was not possible in many instances. It was possible, however, to identify to genus and often to species using high-quality camera trap images (see Burns et al. 2018). To generate the initial dataset, we removed detections made within 1 min of a previous or subsequent detection to ensure each image represented a temporally explicit rodent detection. Individual rodents could not be distinguished, thus it is likely that single individuals were captured across multiple detection events. Quality control checks were performed so as to obtain a pool of high-quality images by removing those that were blurry, and those that did not capture all or most of a rodent’s body. An assessor of intermediate experience (AN) identified these high-quality detections as either Rattus or Mus based on overall body size and comparative tail to body ratio. An expert assessor (PB) then reviewed images and confirmed genus-level identifications based on overall size, head and body shape (see Supplementary Material).
To determine rat species composition (R. exulans or R. rattus) an expert assessor highly experienced in rodent identification from camera trap imagery (PB) reviewed a random subset of all identified Rattus images (15%) stratified across strata and forest type. Rat species were differentiated based on overall size, ear, head and body shape, and tail morphology (Burns et al. 2018; see Supplementary Material for identification guidelines). The initial assessor (AN) then validated identifications before calculating proportions. Any images that could not be identified to genus or species by either assessor were removed from the dataset. We fitted a log-linear model to test the relationship between detection frequencies of Rattus sp. (black rat, Pacific rat) at each forest stratum (ground, mid-storey, canopy).
In combination with distance sampling, thermal surveys have been shown to yield accurate density estimates for small nocturnal endotherms (Bedson et al. 2021; Clarke, unpublished data). Therefore, to quantify rodent density for each forest type we employed distance sampling (Buckland et al. 2001) in conjunction with thermal surveys along 100 m transects. A single 100 m transect was established at each of the 30 sites. All surveys were undertaken at least 1.5 h after sunset and involved a single observer slowly scanning the vegetation for rodents using a thermal scanner (ThermoGEAR G100EX, Nippon Avionics Co., Ltd), while a second team member acted as scribe. To minimise bias, both sides of the transect and all forest strata were evenly sampled over a similar period of time (mean survey time per transect = 21 min). Upon detection of a rodent, the observer recorded the distance perpendicular to the transect (m) and height off the ground (m). Distances and heights of detections were verified in situ with a laser rangefinder where possible, otherwise visual estimates were made. We calibrated observers’ visual estimates at the beginning of each survey night on mock transects using the same laser rangefinder. To obtain an adequate minimum number of rodent detections in each forest type for distance sampling purposes (Buckland et al. 2001), two surveys per site per season were conducted for guava (n = 40) and palm forests (n = 40), and three surveys per site per season for hardwood forests (n = 60).
As managers are often time and resource-limited, we were interested in determining whether continuous measures of activity could be used as a simple proxy for rodent density. In addition to analysing rodent presence/absence, we therefore also developed a continuous rodent activity index (RAI) for each of the three monitoring methods as a potential proxy for relative density (Whisson et al. 2005). For all cameras, the number of rodent-active nights per camera (out of 29 or 30 nights) was used as the basis of the index, where a rodent-active night was defined as one or more rodent detections made between local sunset and sunrise across the 29- or 30- night sampling period. Eight cameras were removed due to malfunction (see Supplementary Material for sample sizes).
Chew card RAI was based on the surface area of each chew card consumed or chewed by rodents. This was calculated by converting digital images of each card to a binary image and calculating the proportion of black (chewed) and white (unchewed) pixels using freeware ImageJ1 Ver. 1.52a (Schneider et al. 2012) (protocol available from FigShare Repository: https://doi.org/10.26180/c.5758037). During opportunistic checks of the monitoring stations (at least once per week), we collected any large sections (up to 50%) of chew cards that had fallen from above-ground stations and excluded them from consideration to avoid over-estimating activity (32 of 180 cards or 18%, see Supplementary Material for sample sizes).
For tracking tunnels, RAI was based on the area of each tracking card that displayed rodent footprints. Using Adobe PhotoShop (Ver. 21.1.1) we overlaid a standardised 8 × 12 cell grid on digital images of each tracking card and calculated the proportion of undamaged cells that contained at least half a rodent footprint (protocol available from FigShare Repository: https://doi.org/10.26180/c.5758037). Seven tracking cards were excluded due to short deployment periods or excessive physical damage to the card caused by weather or rodent chewing (see Supplementary Material for sample sizes).
Statistical analysis
A binomial generalised linear mixed-effects model (GLMM) was used to test the effects of strata and forest type on rodent presence, using R package lme4 (Bates et al. 2015). Covariates in the model included strata (ground, mid-storey, canopy) and forest type (guava, hardwood, palm) as fixed factors, with ground-level stations in guava forest assigned as the reference level. An interaction between the two fixed terms was not included in the final model due to convergence issues. Detection method (camera trap, chew card, tracking tunnel), site ID (30 unique sites), and season (spring, autumn) were included as random effects. Residual diagnostics and dispersion tests were performed using R package DHARMa (Hartig 2021) to ensure the model did not violate underlying assumptions. We conducted Tukey pairwise comparisons for the final model using R package emmeans (Lenth 2021).
To account for differences in transect width (e.g. 5 m-wide 4WD tracks versus <1 m-wide non-public bait-lines), we left-truncated raw detection distances by half the track width for all transects >1 m-wide. Imperfect detection was then modelled using the hazard-rate key function in R package Distance (Miller et al. 2019). The dataset was right-truncated (95th percentile; Buckland et al. 2001), resulting in 349 observations contributing to all tested models. Model performance was assessed using Akaike Information Criterion (AIC) and goodness of fit determined via the package’s inbuilt function (unweighted Cramer-von Mises test). The final model included season as a covariate (Supplementary Material).
To test the effects of forest strata and forest type on RAI we implemented additive GLMMs for each detection method using R package glmmTMB (Brooks et al. 2017). All models included strata (ground, mid-storey, canopy) and forest type (guava, hardwood, palm) as fixed factors, with ground-level stations in guava forest assigned as the reference level. All models also included site ID and season as random effects. We conducted Tukey pairwise comparisons for all final models using R package emmeans (Lenth 2021). To ensure underlying assumptions were met, residual diagnostics and dispersion tests were conducted for all models using R package DHARMa (Hartig 2021).
Using camera trap RAI (count of rodent-active nights) we built a zero-inflated Poisson GLMM to correct for highly zero-inflated simulated values generated using a binomial GLMM. Beta GLMMs were used for assessing chew card RAI and tracking tunnel RAI. As these metrics are continuous proportions as opposed to count-based proportions, beta regression is preferable to standard logistic regression (Douma and Weedon 2019). Beta regression requires there to be no values of exactly 0 or 1 in the response, therefore we transformed all such values by adding and subtracting small amounts to and from 0 and 1, respectively (Smithson and Verkuilen 2006). We considered non-overlapping 95% confidence intervals to infer statistical significance for all models. All statistical analyses were performed in R ver. 4.0.0 (R Core Team 2021).
Results
Rodent presence
Above-ground stations were deployed at heights ranging from 2.1 m to 12.3 m (Fig. 3) and we observed rodents across this vertical range. Rodent presence was uniformly distributed across strata: of the 169 stations (camera, tracking tunnel, and chew cards combined) that detected rodent presence (94% of all stations), 32% were ground-based, 34% were situated in the mid-storey and 34% were situated in the canopy. All passive monitoring methods detected high levels of rodent presence; 73.8% of camera traps, 84.9% of chew cards and 71.4% of tracking tunnels detected at least one rodent during the 29–30-day observation periods. Forest strata had a negligible effect on rodent presence (Table 1, Fig. 4), however, there was evidence of an effect of forest type where we observed a trend of decreased rodent presence in hardwood forest (probability of presence [‘prob’ henceforth] = 0.78; 95% CI = 0.50–0.93), compared to guava (prob = 0.91; 95% CI = 0.72–0.98) and palm forest (prob = 0.88; 95% CI = 0.65–0.97) though this difference was not statistically significant (Table 1). We did not detect any statistically significant difference in rodent presence across forest strata (Table 1), although chew cards tended to record higher proportions of rodent presence, which was consistent across all forest types (Fig. 4). We also recorded anecdotal evidence of arboreal rodent foraging and dwelling at mid-storey and canopy level monitoring stations, including presence of rodent scats, food caches and denning materials (Supplementary Material).
Term | Estimate | s.e. | z statistic | 2.5% | 97.5% | |
---|---|---|---|---|---|---|
(Intercept) | 2.10 | 0.72 | 2.90 | 0.68 | 3.52 | |
StrataMid-storey | 0.38 | 0.29 | 1.27 | −0.20 | 0.95 | |
StrataCanopy | 0.25 | 0.29 | 0.86 | −0.32 | 0.82 | |
VegHardwood | −1.00 | 0.82 | −1.22 | −2.62 | 0.61 | |
VegPalm | −0.31 | 0.84 | −0.38 | −1.95 | 1.32 |
Proportion of monitoring devices that detected rodent presence over a 30-day period separated by forest strata and forest type in Norfolk Island National Park. Rodent presence was recorded by camera traps, chew cards, and tracking tunnels, and was collected across three forest types: invasive red cherry guava (Psidium cattleyanum) thickets, native hardwood forests and native palm forests, and three forest strata: ground (dark grey), mid-storey (light grey) and canopy (white). The number of samples retained per treatment after removing failed cameras, chew cards and tracking tunnels is reported below each bar. Error bars indicate ± s.e. of the sample proportion.
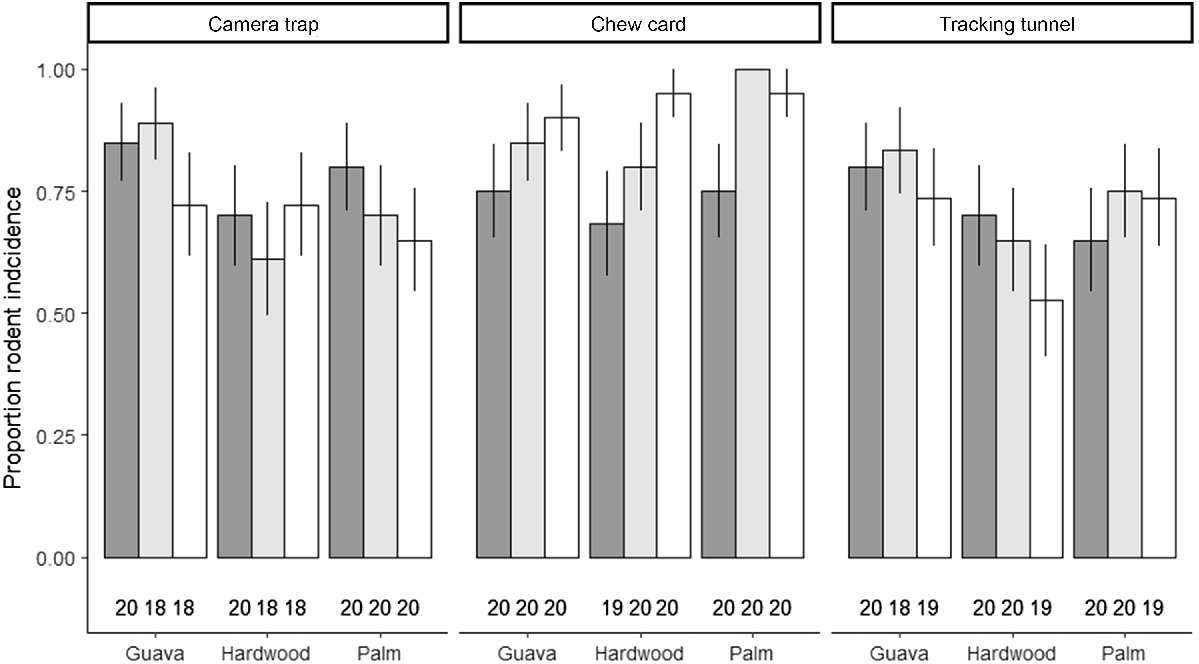
Species composition
Rattus sp. (cf. Mus musculus) represented most camera trap detections, and there was evidence of vertical niche partitioning (Table 2). We detected differences in detection rates across forest strata for both black rats and Pacific rats; black rats were more commonly detected in the mid-storey (predicted detections = 105; 95% CI = 86.7–127.1) compared to the ground (predicted detections = 57; 95% CI = 52.7–85.1) and canopy (predicted detections = 69; 95% CI = 54.5–87.4), and Pacific rats were more commonly detected on the ground (Table 2). House mice contributed 0.3% of assessed camera trap detections (7 of 2355), and were only detected at ground stations (n = 6) barring a single mouse detected at a mid-storey station 3.95 m above ground. All other detections (n = 2348) were identified as Rattus sp. Of the subset of images analysed for Rattus species composition (n = 350), 12% (42 images) could not be identified to species. Black rats were three times more common than Pacific rats (66% and 22% of total detections respectively) and were present in every stratum and forest type (Table 2). The black rat was the dominant arboreal species across all forest types (detection proportions between 0.48 and 0.96), whereas the Pacific rat was the most common ground-active species in hardwood and palm sites (detection proportions = 0.67 and 0.61 respectively; Table 2).
Guava | Hardwood | Palm | |||||||||||
---|---|---|---|---|---|---|---|---|---|---|---|---|---|
All strata | Ground | Mid-storey | Canopy | All strata | Ground | Mid-storey | Canopy | All strata | Ground | Mid-storey | Canopy | ||
Prop. R rattus | 0.82 | 0.62 | 0.93 | 0.89 | 0.59 | 0.22 | 0.85 | 0.48 | 0.57 | 0.34 | 0.96 | 0.74 | |
Prop. R exulans | 0.04 | 0.11 | 0.02 | 0.00 | 0.22 | 0.67 | – | 0.27 | 0.36 | 0.61 | – | – | |
Prop. Rattus sp. | 0.14 | 0.27 | 0.05 | 0.11 | 0.19 | 0.11 | 0.15 | 0.24 | 0.08 | 0.05 | 0.04 | 0.26 | |
Number of images analysed | 122 | 37 | 41 | 44 | 69 | 9 | 27 | 33 | 159 | 94 | 46 | 19 |
Proportions were derived from 350 analysed images stratified across forest type and strata. Sample sizes (images analysed) represent randomly sampled subsets of total detections made in each habitat niche (15% of detections made per habitat niche). Detections that could not be identified to species level were pooled as ‘Rattus sp.’ (i.e. the detection could be either R. rattus or R. exulans). House mice (Mus musculus) were not included in calculations due to being detected in <1% of images.
Rodent density
The majority of rodent detections (80%) during thermal surveys occurred above ground at a maximum height of 15 m (mean height = 5.1 m ± 0.17 m). Rodent density derived from distance sampling was 14.3 rodents/ha (95% CI = 12.3–16.6) across the entire surveyed area, though density varied with forest type (Supplementary Material). Mean density in hardwood forest was lowest (9.9 rodents/ha; 95% CI = 7.4–13.3), and statistically significantly lower compared to guava forests, which supported the highest densities (19.1 rodents/ha; 95% CI = 15.8–23.1). Palm forest supported intermediate densities (16.1 rodents/ha; 95% CI = 12.5–20.6) and was not statistically significantly different from densities observed in hardwood or guava forests. Detectability was lower in spring (2019) and higher in autumn (2020) when compared to the overall detection probability (Supplementary Material).
Rodent activity indices
High levels of variation in RAI were observed across strata and forest types for all monitoring methods resulting in wide confidence intervals (Supplementary Material). The median camera trap RAI was four (range = 0–30), median chew card RAI was 0.58 (range = 0–1), and median tracking tunnel RAI 0.54 (range = 0–0.95). For all indices, we observed a trend of lower activity in hardwood sites compared to other forest types though these differences were not statistically significant (Supplementary Material). Strata had a negligible effect on all rodent activity indices (Supplementary Material). Plotted residuals for all RAI models exhibited significant deviation from normality, suggesting that there are additional undescribed variables that would improve model fit and performance.
Discussion
In a recent review, Taggart et al. (2023) called for a better understanding of how invasive animals use and move through their environment in order to design more targeted and effective conservation baiting strategies; our study directly addresses this by assessing invasive rodent activity in different forest strata. Previous studies have demonstrated that invasive rodents are capable climbers (Innes and Skipworth 1983; Shiels 2010; Foster et al. 2011; King et al. 2011), however this study provides a more comprehensive and species-specific assessment of this behaviour, in a system where long-term ground-based baiting has occurred (~1800 bait stations in the study system). These results provide compelling evidence that rats frequently use all vertical strata across structurally variable forest types, with most of this activity attributable to black rats and, to a lesser extent, Pacific rats. Further, we demonstrated that vertical activity patterns across forest strata are similar despite different physical forest structures and observed differences in rodent density. In systems where the effectiveness of long-term control strategies is declining or where eradication programs have failed, a mixed strategy of baiting across different strata (both on the ground and above) could lead to improved outcomes, particularly where black rats are present.
Black rats were the most common species overall and the dominant arboreal species, whereas most of the ground-level activity was attributable to Pacific rats. This observed dominance of black rats is consistent with previous studies (Harper 2006; Rendall et al. 2014), as is the evidence for vertical niche partitioning between black and Pacific rats (Shiels 2010; Witmer and Shiels 2018). Our findings also mirror those of a study conducted on Dekehtik Island, which found that although Pacific rats occupied the canopy during the day, they spent most of their active nocturnal hours on the ground (Wegmann et al. 2011). As such, the activity observed in the present study needs to be considered in the context of species-specific behaviour and composition. Except in guava sites, black rats only made up between one-fifth and one-third of rat detections at the ground level. Although studies have shown that arboreal rodents do come to ground (e.g. Dowding and Murphy 1994; Key and Woods 1996; Pryde et al. 2005; Shiels 2010; Loveridge et al. 2016), probability theory dictates that species that spend less time on the ground are likely to encounter ground-based devices less often. It then follows that ground-based rodent monitoring in this and similar systems has the potential to under-estimate the extent of black rat activity. As the black rat is considered one of the most harmful invasive rodent species globally (Banks and Hughes 2012), under-estimating their activity has substantial implications for the efficacy of subsequent control efforts.
There are various explanations for the distribution of rodent activity across strata observed in this study. It is possible that the high levels of arboreal activity could be a result of the long-term ground-based control program selecting for such behaviour. This is a plausible scenario considering that the rodent population in the Norfolk Island National Park has experienced extensive ground-based control for three decades. Long-term control programs are already known to impose substantial selective pressures on target species, creating populations that exhibit genetic resistance to toxins and behavioural resistance to bait stations (Allsop et al. 2017). Therefore, multi-decadal ground-based programs could impose an additional selective pressure on ground-based foraging. However, black rats are morphologically adapted to arboreal movement (Alpin et al. 2003; Loveridge et al. 2016; Smith et al. 2016), and arboreal Rattus behaviour was observed in the Norfolk Island National Park prior to any intensive baiting (Robinson 1988). The presence of free-roaming cats as predators may also drive arboreal behaviour, though rodents on Norfolk Island are also subject to predation from an aerial predator, the native owl (Sperring et al. 2021). Predation pressure from above and below could explain the significantly higher presence of black rats observed in the mid-storey compared to the ground and canopy. Variation in food sources across strata may also influence rodent activity, however, known and potential food sources are distributed across all strata: both tree-nesting and ground-nesting birds are present in the National Park, as are ground-dwelling and arboreal invertebrates such as snails, insects and other arthropods (Director of National Parks 2023). Seeds and fruits are also present across all strata, though mass seeding and fruiting events may lead to a higher abundance of resources in the canopy during certain times of the year, which could drive more arboreal activity. Whatever the drivers, arboreal activity is a key aspect of Rattus ecology on Norfolk Island, and exploiting this part of their niche through the incorporation of above-ground control may improve outcomes. This is one of numerous ways in which managers can exploit pest animal behaviour to improve control, thus other avenues should not be overlooked (see Garvey et al. 2020).
Implementation of above-ground control and monitoring will likely increase costs and effort compared to a solely ground-based program, however, an improvement in efficacy may offset those costs. In systems like Norfolk Island that experience high levels of rodent activity despite intensive and ongoing ground-based control, the long-term environmental and economic costs associated without such implementation would be much higher. By deploying control mechanisms above-ground in conjunction with ground-based control, our results suggest that land managers would likely increase encounter rates and subsequently, mortality rates of invasive rodents, particularly black rats (Tobin et al. 1997; Wegmann et al. 2014; Ringler et al. 2021). Raising bait stations off the ground can also mitigate interference from non-target species (Keitt et al. 2015). Preliminary results from small-scale trials in NINP undertaken in 2021 have produced promising results; a pilot field trial of lethal traps (Goodnature A24) deployed across three forest strata showed that canopy traps (6–10 m above-ground) were responsible for nearly 80% of rodent kills, all of which were identified as R. rattus (Nance et al. 2022). A subsequent camera-monitored trial conducted within the NINP found that by deploying rodenticide-baited tunnels ~1.5 m above-ground, bait uptake by rodents increased by 60%, and non-target bait uptake by feral chickens ceased (M. Wilson, unpublished data). Although these trials spanned relatively short sampling periods, results to date are encouraging. To determine if such gains can be maintained over time and across systems, longer-term and larger-scale trials that directly assess the efficacy of above-ground lethal trapping are needed. Additionally, assessing the lowest heights at which bait stations can be deployed while delivering meaningful rodent suppression outcomes will support managers in developing resource-efficient control programs. Finally, understanding the level of rodent control (at or above-ground) required to benefit native species, such as through the implementation of density-impact functions (Norbury et al. 2015), will be critical to the ongoing development of any rodent control program.
Although all activity indices developed in this study demonstrated similar trends for density estimates, high site-level variation suggests that a continuous measure of activity for chew cards and tracking tunnels is unlikely to yield reliable measures of relative density. Conversely, the activity index developed for camera trap detections (rodent-active nights) may be more informative, with the camera trap RAI aligning closely with the more robust thermal survey-based density estimates. Unlike camera traps, activity indices derived from chew cards and tracking tunnels lack temporal resolution, therefore it is not possible to know if high RAI (i.e. heavily chewed cards or heavily tracked tunnels) were produced by one very active rodent or many rodents. On the other hand, the use of camera traps for estimating densities of ground-dwelling mammals has been validated previously using theoretical and empirical evidence (Rowcliffe et al. 2008). Camera trapping to monitor for arboreal mammals is in its infancy (Haysom et al. 2021; Moore et al. 2021), however practical outputs such as that produced by Moore et al. (2021) will undoubtedly lead to improved utility of camera traps in above-ground strata over time. The use of a camera trap index to infer relative density of arboreal invasive rodents is therefore likely to become more precise, and could become a valuable alternative to more effort-intensive survey methods.
When comparing ground-based and above-ground devices, the potential for detectability biases should be considered. For example, the detection zone of a ground-based camera trap will be larger than that of an above-ground camera trap, as above-ground detection zones would be reduced by the empty space that a rodent could not traverse. Conversely, above-ground monitoring may be susceptible to the ‘funnelling’ of rodents near monitoring devices as rodents move to and from the ground along trunks and branches. This has the potential to inflate above-ground activity indices compared to ground-based indices where such funnelling would not occur. This could explain why black rats were more commonly detected in the mid-storey than the canopy. It is worth noting that in the context of rodent control, funnelling arboreal rodents past control devices may actually confer a benefit, as higher encounter rates may lead to higher mortality rates. When monitoring is the primary objective however, programs should consider such differences in detectability when comparing rodent activity across strata.
It is possible that rodents in this system have developed resistance to control (Allsop et al. 2017), though we believe the frequent rotation of rodenticide used in the study system would reduce the likelihood or prevalence of genetic resistance (see Materials and methods: Long-term rodent control). We did not test for this phenomenon in the present study, thus a clearer understanding of the prevalence of resistance, genetic or otherwise, among the rodent population would provide useful additional insight. However, since incorporating tree-based baiting in the NINP rodent control program, practitioners are recording measurable declines in rat activity through independent monitoring (M. Wilson, unpublished data). It is likely therefore that there are multiple causal factors, including arboreal behaviour, that impact the effectiveness of long-term ground-based rodent control programs.
Invasive arboreal rodents are a global threat to native arboreal species. This study highlights the need to understand the dynamics of species-specific rodent activity to design ecologically informed control in systems where long-term programs are experiencing a decline in efficacy, or when planning eradication campaigns for target species that exhibit arboreal behaviour. We provide evidence that neither height off the ground nor vegetation structure limit invasive rat movement above-ground, and suggest that ground-based control alone may not take full advantage of species’ behaviour when targeting the black rat. We argue that a misalignment between arboreal black rat activity and traditional control measures should be considered when assessing the risks to biodiversity, and when reviewing the performance of long-term rodent control programs. These results provide novel insights that can improve the long-term control of invasive rodents, recognising that replication across systems will further our understanding. In systems where black rats persist and the risk for non-target mortality of native species is low, it may be beneficial for practitioners to trial rodent baiting and trapping above-ground. This approach may be particularly beneficial where native species are threatened with imminent extinction and long-term rodent control programs are failing. Understanding the behavioural ecology of invasive species is necessary if we are to successfully minimise and mitigate their environmental and socioeconomic impacts. Research that seeks to optimise the efficacy of above-ground rodent control will be an important step to address the global conservation threat posed by invasive rodents.
Data availability
Rodent activity and thermal survey data, and protocols for processing chew cards and tracking tunnels are published and publicly available through Monash FigShare collection: https://doi.org/10.26180/c.5758037.
Declaration of funding
This research was funded by Parks Australia [grant number 281969256] under a collaborative agreement between the Director of National Parks and Monash University. AHN was supported by an Australian Government Research Training Program Scholarship.
Acknowledgements
We thank the management team of Norfolk Island National Park for their knowledge contributions and dedicated support throughout this project: Nigel Greenup, Joel Christian, Ken Christian and Ross Quintal. Nicholas Macgregor at Parks Australia is also thanked for his support. We are grateful to field volunteers Kathleen Garland, Nicholas Wiggins, Mark Hallam and Judith Andersen for their generous contributions. We thank Benjamin Viola for his administrative support throughout this project, and Timothy Jones for advice on statistical analysis. All research was conducted under an approved Parks Australia Management Plan, and Monash University Animal Ethics approval 2021-14636-59388.
References
Allsop SE, Dundas SJ, Adams PJ, Kreplins TL, Bateman PW, Fleming PA (2017) Reduced efficacy of baiting programs for invasive species: some mechanisms and management implications. Pacific Conservation Biology 23, 240-257.
| Crossref | Google Scholar |
Australian Bureau of Statistics (2021) Norfolk Island. 2021 census all persons QuickStats: area code SAL90004. Available at https://www.abs.gov.au/census/find-census-data/quickstats/2021/SAL90004
Banko PC, Jaenecke KA, Peck RW, Brinck KW (2019) Increased nesting success of Hawaii Elepaio in response to the removal of invasive black rats. The Condor 121, duz003.
| Crossref | Google Scholar |
Banks PB, Hughes NK (2012) A review of the evidence for potential impacts of black rats (Rattus rattus) on wildlife and humans in Australia. Wildlife Research 39, 78-88.
| Crossref | Google Scholar |
Bates D, Mächler M, Bolker B, Walker S (2015) Fitting linear mixed-effects models using lme4. Journal of Statistical Software 67, 1-48.
| Crossref | Google Scholar |
Bedson CPE, Thomas L, Wheeler PM, Reid N, Harris WE, Lloyd H, Mallon D, Preziosi R (2021) Estimating density of mountain hares using distance sampling: a comparison of daylight visual surveys, night-time thermal imaging and camera traps. Wildlife Biology 2021, wlb.00802.
| Crossref | Google Scholar |
Bellard C, Cassey P, Blackburn TM (2016) Alien species as a driver of recent extinctions. Biology Letters 12, 20150623.
| Crossref | Google Scholar | PubMed |
Blackwell GL, Potter MA, McLennan JA (2002) Rodent density indices from tracking tunnels, snap-traps and Fenn traps: do they tell the same story? New Zealand Journal of Ecology 26, 43-51.
| Google Scholar |
Brooks ME, Kristensen K, van Benthem KJ, Magnusson A, Berg CW, Nielsen A, Skaug HJ, Mächler M, Bolker BM (2017) glmmTMB balances speed and flexibility among packages for zero-inflated generalized linear mixed modeling. The R Journal 9, 378-400.
| Crossref | Google Scholar |
Burge OR, Kelly D, Wilmshurst JM (2017) Interspecies interference and monitoring duration affect detection rates in chew cards. Austral Ecology 42, 522-532.
| Crossref | Google Scholar |
Burns PA, Parrott ML, Rowe KC, Phillips BL (2018) Identification of threatened rodent species using infrared and white-flash camera traps. Australian Mammalogy 40, 188-197.
| Crossref | Google Scholar |
Doherty TS, Glen AS, Nimmo DG, Ritchie EG, Dickman CR (2016) Invasive predators and global biodiversity loss. Proceedings of the National Academy of Sciences 113, 11261-11265.
| Crossref | Google Scholar |
Douma JC, Weedon JT (2019) Analysing continuous proportions in ecology and evolution: a practical introduction to beta and Dirichlet regression. Methods in Ecology and Evolution 10, 1412-1430.
| Crossref | Google Scholar |
Dowding JE, Murphy EC (1994) Ecology of ship rats (Rattus rattus) in a Kauri (Agathis australis) forest in Northland, New Zealand. New Zealand Journal of Ecology 18, 19-27.
| Google Scholar |
Dueñas M-A, Hemming DJ, Roberts A, Diaz-Soltero H (2021) The threat of invasive species to IUCN-listed critically endangered species: a systematic review. Global Ecology and Conservation 26, e01476.
| Crossref | Google Scholar |
Duron Q, Shiels AB, Vidal E (2017) Control of invasive rats on islands and priorities for future action. Conservation Biology 31, 761-771.
| Crossref | Google Scholar | PubMed |
Engeman RM, Avery ML, Shiels AB, Berentsen AR, VerCauteren KC, Sugihara RT, Duffiney AG, Clark CS, Eisemann JD (2018) Diverse examples from managing invasive vertebrate species on inhabited islands of the United States. Australasian Journal of Environmental Management 25, 43-61.
| Crossref | Google Scholar |
Foster S, King C, Patty B, Miller S (2011) Tree-climbing capabilities of Norway and ship rats. New Zealand Journal of Zoology 38, 285-296.
| Crossref | Google Scholar |
Garvey PM, Banks PB, Suraci JP, Bodey TW, Glen AS, Jones CJ, McArthur C, Norbury GL, Price CJ, Russell JC, Sih A (2020) Leveraging motivations, personality, and sensory cues for vertebrate pest management. Trends in Ecology & Evolution 35, 990-1000.
| Crossref | Google Scholar | PubMed |
Geosciences Australia (2021) Norfolk Island. Available at https://www.ga.gov.au/scientific-topics/national-location-information/dimensions/remote-offshore-territories/norfolk-island
Gronwald M, Russell JC (2022) Behaviour of invasive ship rats, Rattus rattus, around Goodnature A24 self-resetting traps. Management of Biological Invasions 13, 479-493.
| Crossref | Google Scholar |
Hadfield MG, Saufler JE (2009) The demographics of destruction: isolated populations of arboreal snails and sustained predation by rats on the island of Moloka’i 1982-2006. Biological Invasions 11, 1595-1609.
| Crossref | Google Scholar |
Harper GA (2006) Habitat use by three rat species (Rattus spp.) on an island without other mammalian predators. New Zealand Journal of Ecology 30, 321-333.
| Google Scholar |
Harper GA, Bunbury N (2015) Invasive rats on tropical islands: their population biology and impacts on native species. Global Ecology and Conservation 3, 607-627.
| Crossref | Google Scholar |
Hartig F (2021) Dharma: residual diagnostics for heirarchical (multi-level/mixed) regression models: R package version 0.4.3. Available at http://florianhartig.github.io/DHARMa/
Haysom JK, Deere NJ, Wearn OR, Mahyudin A, Jami J, Reynolds G, Struebig MJ (2021) Life in the canopy: using camera-traps to inventory arboreal rainforest mammals in Borneo. Frontiers in Forests and Global Change 4, 673071.
| Crossref | Google Scholar |
Howald G, Donlan CJ, Galván JP, Russell JC, Parkes J, Samaniego A, Wang Y, Veitch D, Genovesi P, Pascal M, Saunders A, Tershy B (2007) Invasive rodent eradication on islands. Conservation Biology 21, 1258-1268.
| Crossref | Google Scholar | PubMed |
Innes JG, Skipworth JP (1983) Home ranges of ship rats in a small New Zealand forest as revealed by trapping and tracking. New Zealand Journal of Zoology 10, 99-110.
| Crossref | Google Scholar |
Keitt B, Griffiths R, Boudjelas S, Broome K, Cranwell S, Millett J, Pitt W, Samaniego-Herrera A (2015) Best practice guidelines for rat eradication on tropical islands. Biological Conservation 185, 17-26.
| Crossref | Google Scholar |
Key GE, Woods RD (1996) Spool-and-line studies on the behavioural ecology of rats (Rattus spp.) in the Galápagos Islands. Canadian Journal of Zoology 74, 733-737.
| Crossref | Google Scholar |
Kier G, Kreft H, Lee TM, Jetz W, Ibisch PL, Nowicki C, Mutke J, Barthlott W (2009) A global assessment of endemism and species richness across island and mainland regions. Proceedings of the National Academy of Sciences 106, 9322-9327.
| Crossref | Google Scholar |
King CM, Foster S, Miller S (2011) Invasive European rats in Britain and New Zealand: same species, different outcomes. Journal of Zoology 285, 172-179.
| Crossref | Google Scholar |
Krijger IM, Belmain SR, Singleton GR, Groot Koerkamp PWG, Meerburg BG (2017) The need to implement the landscape of fear within rodent pest management strategies. Pest Management Science 73, 2397-2402.
| Crossref | Google Scholar | PubMed |
Lenth RV (2021) Emmeans: estimated marginal means, aka least-squares means: R package version 1.6.3. Available at https://github.com/rvlenth/emmeans
Lindsey GD, Mosher SM, Fancy SG, Smucker TD (1999) Population structure and movements of introduced rats in an Hawaiian rainforest. Pacific Conservation Biology 5, 94-102.
| Crossref | Google Scholar |
Loveridge R, Wearn OR, Vieira M, Bernard H, Ewers RM (2016) Movement behavior of native and invasive small mammals shows logging may facilitate invasion in a tropical rain forest. Biotropica 48, 373-380.
| Crossref | Google Scholar |
Miller DL, Rexstad E, Thomas L, Marshall L, Laake JL (2019) Distance sampling in R. Journal of Statistical Software 89, 1-28.
| Crossref | Google Scholar |
Moore JF, Soanes K, Balbuena D, Beirne C, Bowler M, Carrasco-Rueda F, Cheyne SM, Coutant O, Forget P-M, Haysom JK, Houlihan PR, Olson ER, Lindshield S, Martin J, Tobler M, Whitworth A, Gregory T (2021) The potential and practice of arboreal camera trapping. Methods in Ecology and Evolution 12, 1768-1779.
| Crossref | Google Scholar |
Nance AH, Mitchell WF, Dawlings F, Cook CN, Clarke RH (2023) Rodent predation and specialised avian habitat requirements drive extinction risk for endemic island songbirds in the south-west Pacific. Emu – Austral Ornithology 123, 217-231.
| Crossref | Google Scholar |
Nelson JT, Woodworth BL, Fancy SG, Lindsey GD, Tweed EJ (2002) Effectiveness of rodent control and monitoring techniques for a montane rainforest. Wildlife Society Bulletin 30, 82-92.
| Google Scholar |
Norbury GL, Pech RP, Byrom AE, Innes J (2015) Density-impact functions for terrestrial vertebrate pests and indigenous biota: guidelines for conservation managers. Biological Conservation 191, 409-420.
| Crossref | Google Scholar |
Pryde M, Dilks P, Fraser I (2005) The home range of ship rats (Rattus rattus) in beech forest in the Eglinton Valley, Fiordland, New Zealand: a pilot study. New Zealand Journal of Zoology 32, 139-142.
| Crossref | Google Scholar |
Rendall AR, Sutherland DR, Cooke R, White J (2014) Camera trapping: a contemporary approach to monitoring invasive rodents in high conservation priority ecosystems. PLoS ONE 9, e86592.
| Crossref | Google Scholar | PubMed |
Ringler D, Guillerault N, Baumann M, Cagnato M, Russell JC (2021) Rodenticide baiting black rats (Rattus rattus) in mangrove habitats. Wildlife Research 48, 554-560.
| Crossref | Google Scholar |
Rowcliffe JM, Field J, Turvey ST, Carbone C (2008) Estimating animal density using camera traps without the need for individual recognition. Journal of Applied Ecology 45, 1228-1236.
| Crossref | Google Scholar |
Ruffino L, Zarzoso-Lacoste D, Vidal E (2015) Assessment of invasive rodent impacts on island avifauna: methods, limitations and the way forward. Wildlife Research 42, 185-195.
| Crossref | Google Scholar |
Schneider CA, Rasband WS, Eliceiri KW (2012) NIH Image to ImageJ: 25 years of image analysis. Nature Methods 9, 671-675.
| Crossref | Google Scholar | PubMed |
Shiels AB, Ramírez de Arellano GE (2019) Habitat use and seed removal by invasive rats (Rattus rattus) in disturbed and undisturbed rain forest, Puerto Rico. Biotropica 51, 378-386.
| Crossref | Google Scholar |
Shiels AB, Pitt WC, Sugihara RT, Witmer GW (2014) Biology and impacts of Pacific island invasive species. 11. Rattus rattus, the black rat (Rodentia: Muridae). Pacific Science 68, 145-184.
| Crossref | Google Scholar |
Smith HM, Dickman CR, Banks PB (2016) Nest predation by commensal rodents in urban bushland remnants. PLoS ONE 11, e0156180.
| Crossref | Google Scholar | PubMed |
Smithson M, Verkuilen J (2006) A better lemon squeezer? Maximum-likelihood regression with beta-distributed dependent variables. Psychological Methods 11, 54-71.
| Crossref | Google Scholar | PubMed |
Spatz DR, Zilliacus KM, Holmes ND, Butchart SHM, Genovesi P, Ceballos G, Tershy BR, Croll DA (2017) Globally threatened vertebrates on islands with invasive species. Science Advances 3, e1603080.
| Crossref | Google Scholar |
Sweetapple P, Nugent G (2011) Chew-track-cards: a multiple-species small mammal detection device. New Zealand Journal of Ecology 35, 153-162.
| Google Scholar |
Taggart PL, Taylor P, Patel KK, Noble DWA (2023) Baiting in conservation and pest management: a systematic review of its global applications in a changing world. Biological Conservation 284, 110214.
| Crossref | Google Scholar |
Tng DYP, Goosem MW, Paz CP, Preece ND, Goosem S, Fensham RJ, Laurance SGW (2016) Characteristics of the Psidium cattleianum invasion of secondary rainforests. Austral Ecology 41, 344-354.
| Crossref | Google Scholar |
Tobin ME, Sugihara RT, Koehler AE (1997) Bait placement and acceptance by rats in macadamia orchards. Crop Protection 16, 507-510.
| Crossref | Google Scholar |
Whisson DA, Engeman RM, Collins K (2005) Developing relative abundance techniques (RATs) for monitoring rodent populations. Wildlife Research 32, 239-244.
| Crossref | Google Scholar |
Williams BK, Brown ED (2014) Adaptive management: from more talk to real action. Environmental Management 53, 465-479.
| Crossref | Google Scholar | PubMed |