Increased stratification intensifies surface marine heatwaves north-east of Aotearoa New Zealand in New Zealand’s Earth System model
Liv Cornelissen
A
B
Abstract
The Western Boundary Current system in the South Pacific is an important element of the climate system as it carries heat from the tropical regions poleward. The East Auckland Current (EAUC) flows along the continental shelf break of Aotearoa New Zealand’s North Island, transporting heat into this region. Sea surface temperatures (SSTs) increase ~0.15–0.2°C per decade in this region, just above the global average, and marine heatwaves (MHWs) are projected to intensify despite a predicted decline in oceanic volume transport in this region. This study investigates the possible drivers of the extreme oceanic warming in a low (SSP1–2.6), medium (SSP2–4.5) and high (SSP3–7.0) emission scenario using New Zealand’s Earth System model. Our projections suggest a mean decline of heat transport in the East Auckland Current of 5.3% in SSP1–2.6, 22% in SSP2–4.5 and 46% in SSP3–7.0. Although net heat transport (top 1000 m) within the East Auckland Current is projected to decline, the heat near the surface intensifies. This in turn leads to an increase in stratification, shallower mixed layers, by 5 m in SSP1–2.6, 15 m in SSP2–4.5 and 30 m in SSP3–7.0, and more intense surface MHWs, despite a net decline in heat transport into this region. Increased stratification in the top 250 m contributes to the surface warming of the SSTs in all SSPs, which reach ~2°C in SSP1–2.6 to 4°C warming in SSP3–7.0. Despite an overall decline in oceanic heat transport into this region, MHWs are projected to further intensify owing to sustained surface warming and reduced wind-induced vertical mixing.
Keywords: earth system model, future prediction, heat transport, marine heatwaves, New Zealand, sea surface temperature, stratification, trends, western boundary current.
1.Introduction
Increasing anthropogenic greenhouse gases concentrations cause the earth to warm (e.g. Bodeker et al. 2022), with a global heat gain of ~0.67 W m–2 (Storto et al. 2017). Of this excess heat, between 89 and 93% (Cheng et al. 2019; Von Schuckmann et al. 2020) is taken up by the ocean (Johnson and Lyman 2020), with global sea surface temperatures (SSTs) increasing by ~0.13°C per decade (Huang et al. 2020). The rate of warming varies substantially between ocean basins and regions (Wu et al. 2012; Yang et al. 2016; Johnson and Lyman 2020). Most of New Zealand’s ocean experiences warming trends of between 0.2 and 0.35°C per decade (1981–2023). The Tasman Sea and the east of New Zealand’s North Island warm particularly fast (Sutton and Bowen 2019; Sutton et al. 2024). This temperature rise increases the likelihood for heat extremes, such as marine heatwaves (MHWs; Hobday et al. 2016, 2018; Holbrook et al. 2020), which affect marine ecosystems (Holbrook et al. 2020). Island nations in particularly are vulnerable to these events as their economies often rely on fisheries and marine tourism (Smith et al. 2021).
The New Zealand region has experienced an elevated number of MHWs over the past decade (Behrens et al. 2019; Salinger et al. 2019, 2020, 2023, 2024; Montie et al. 2023) and climate projections suggest that MHW intensity and frequency will further increase in parallel with increasing greenhouse gas emissions (Behrens et al. 2022). MHWs are predominantly driven by elevated surface heat fluxes (e.g. blocking high pressure systems, which are associated with low winds that reduce mixing and with higher surface exposure to solar radiation due to reduced cloud cover) or by changes in oceanic heat advection, with a nearly even split between both drivers (Gregory et al. 2023) identified around New Zealand. Atmospheric drivers predominantly control the intensity whereas oceanic heat advection affects the duration of MHWs (Kerry et al. 2022; Gregory et al. 2023).
A main oceanic heat pathway across the Tasman Sea towards New Zealand is the Tasman Front (TF). This warm surface intensified eddy field (Oke et al. 2019) originates from the separation of the East Australian Current (EAC) at ~31–34°S (Ridgway and Dunn 2003), with the remainder of the current flowing south along the continental shelf slope as the East Australian Current extension (EAC ext.), which partly exits the Tasman Sea through the Tasman Leakage (TL). Future climate projections suggest little or no change to volume transports of the EAC but an increase in the TL, and a reduction of the Tasman Front (Hill et al. 2011; Sen Gupta et al. 2021). The TF is an eastward-flowing band of subtropical water that connects the East Australian Current with the North Cape, New Zealand, and the East Auckland Current (EAUC). The EAUC flows south along the continental shelf to East Cape. South of East Cape, the East Cape Current (ECC) flows south along the east coast, turning eastward when it reaches Chatham Rise, and then exits the region into the South Pacific (Chiswell et al. 2015). Changes in heat transport towards New Zealand will affect the habitat of several marine ecosystems (Smale et al. 2019). Despite the projected decline in volume transport towards New Zealand (Sen Gupta et al. 2021) and associated changes in heat transport, climate projections suggest an enhanced surface warming and further increase in MHW intensities around northern New Zealand in the future, with MHWs being projected to intensify at a higher rate compared with the subantarctic waters (Sung et al. 2021; Behrens et al. 2022; Rickard et al. 2023).
In this study, we explore the causes of the ongoing surface warming and the drivers of intensifying MHWs in future projections for the region north-east of New Zealand using the New Zealand Earth System Model (Williams et al. 2016; Behrens et al. 2020). We aim to understand why marine heatwaves are projected to intensify north-east of New Zealand despite a decline in oceanic heat transport from the tropics. Our study examines oceanic heat transport and atmospheric fluxes in the region to identify the primary source for surface heat and its dissipation into the deeper ocean and how this helps to explain the expected intensification of MHWs in the 2050–2099 period. This work builds on the Bogers-Cornelissen (2022) study.
This paper is structured as follows: Section 2 describes the model used to compute the heat budget for the study region; the results of the heat budget analysis are described in Section 3, and possible drivers of the intensification of the MHWs, including ocean heat advection, ocean–atmosphere fluxes, the mixed layer depth and wind stress over the area are analysed. The conclusion and discussion are presented in Section 4.
2.Methods
2.1. Model description
The New Zealand Earth System Model (NZESM; Williams et al. 2016) is a fully coupled Earth System model based on its parent model, UK Earth System model (UKESM) (Kuhlbrodt et al. 2018; Storkey et al. 2018; Sellar et al. 2019). Ocean physics is simulated through Nucleus for European Modelling of the Ocean (NEMO) (Madec et al. 2017), ocean biogeochemistry through Model of Ecosystem Dynamics, nutrient Utilisation, Sequestration and Acidification (MEDUSA) (Yool et al. 2013), sea-ice through The Los Alamos sea ice model CICE (Rae et al. 2015; Hunke et al. 2017) and atmospheric physics and chemistry, including aerosols, through Met Office Unified Model (UM) (Walters et al. 2019). The main difference between NZESM and UKESM is the dynamical oceanic downscaling that has been embedded in NZESM around New Zealand to improve the representation of the oceanic circulation (Behrens et al. 2020). The dynamical downscaling has been applied from 132.7°E to 143.7°W and 60.17 to 10.75°S, with a nominal resolution of 1/5° using Adaptive Grid Refinement in Fortran (AGRIF; Debreu et al. 2008). Outside the nested region, a global tripolar 1 × 1 model grid has been used to simulate ocean properties. Further NZESM model details, validation and impacts of the dynamical downscaling in NZESM can be found in Behrens et al. (2020).
2.2. Model simulations
For this study, we use four NZESM simulations, consisting of a historical simulation from 1950 to 2014 and three future projections with different greenhouse gas emissions shared socioeconomic scenarios (SSP1–2.6, SSP2–4.5, SSP3–7.0; Meinshausen et al. 2020) following the widely used Coupled Modelling Intercomparison Project 6 protocol. To reduce computational demand and allow a direct comparison with UKESM, initial conditions from related historical simulations of UKESM in 1950 were used to initialise the historical simulations of NZESM. The future projections cover the period 2015–2100, which represent low (SSP1–2.6), moderate (SSP2–4.5) and high (SSP3–7.0) future greenhouse gas emission scenarios. These simulations have been analysed for marine heatwaves around New Zealand (Behrens et al. 2022) and used to predict deep sea coral distributions (Anderson et al. 2020, 2022; Stephenson et al. 2023).
2.3. Diagnostics
In this study, we conduct a heat budget analysis, similarly to Behrens et al. (2019), over our target region to investigate why MHW intensities continue to increase in the future, despite the projected decrease in volume and associated heat transport into the region. We compute the oceanic heat transport over the study area (Fig. 1a and Table 1) with corner points (A–E), hereafter referred to as the target region. The enclosed region is dominated by two oceanic currents, the EAUC, which is partly sourced from the TF through the section (A–B), and the ECC, which carries water southward through section (D–E) (Fig. 1a). Flow across the other boundaries does not include any major oceanic currents but net heat transport is not negligible, as specified in the results section. There is net heat transport into the region across the western section and out of the region across the northern, eastern and southern section. Modelled meridional and zonal velocities in combination with potential temperature were used to quantify the oceanic heat transport in and out of our target region, using Eqn 1:
where HT is heat advection (kg·m2 s−3), ρ is the density of ocean water (kg m−3) (set to be constant at 1025 kg m−3), cp is the specific heat (kg·m2 s−2·kg−1·K−1), T is the temperature (K), v is the velocity (m s−1), dl is the distance north or south depending on the section (m). The sum of the oceanic heat fluxes over the boundaries was then compared with oceanic heat content (OHC, kg·m2 s−2) anomalies of the top 1000 m over the target region by integrating potential temperature over the enclosed volume:
The anomalies are the difference between the mean over 2050 and 2099 of each future pathway and the historical mean (1995–2014). Furthermore, the ocean–atmosphere surface heat flux over the region and heat transported to below 1000 m were diagnosed to close the budget and identify future drivers for MHWs in this region. This allowed us to identify the heat sources and sinks of the region. Volume transports across the sections were also computed to quantify changes to the oceanic circulation.
(a) Schematic of surface ocean currents in our study area (cyan box): East Australian Current (EAC), East Auckland Current (EAUC) and East Cape Current (ECC). Grey contour lines show ocean bathymetry (1000 and 3000 m). Table 1 provides the locations of the boundary for our study area indicated by the corner points A–E. Median marine heatwave intensity anomalies relative to 1995–2014 as projected from New Zealand Earth System Model by 2080–2099 for (b) low (SSP1–26), (c) moderate (SSP2–4.5), and (d) high (SSP3–7.0) greenhouse gas emission scenarios. The blue hatched regions indicate areas where the change in median MHW intensity is not statistically significant (P > 0.01) compared to the historical reference period. (c) and (d) adapted from Behrens et al. (2022).
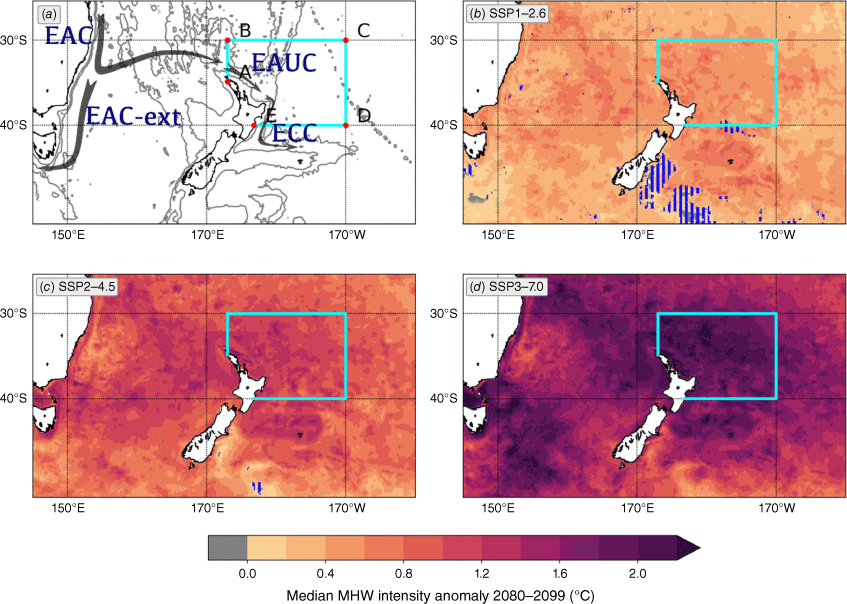
Historical (TW) | SSP1–2.6 (TW) | SSP2–4.5 (TW) | SSP3–7.0 (TW) | ||
---|---|---|---|---|---|
Western section (AB) | 556.47 | 700.79 (+144.32) | 509.32(−47.15) | 355.48 (−200.99) | |
EAUC | 756.00 | 892.42 (+136.42) | 725.88 (−30.12) | 496.15 (−259.85) | |
Northern section (BC) | −241.39 | −345.87 (−104.48) | −263.61 (−22.22) | −217.19 (+24.20) | |
Eastern section (CD) | 2212137.60 | −155.61 (−18.01) | −75.86 (+61.74) | 23.97 (+161.57) | |
Southern section (DE) | −163.92 | −181.02 (−17.10) | −152.23 (+11.69) | −148.58 (+15.34) | |
ECC | 388.61 | 383.40(−5.21) | 383.23 (−5.38) | 372.22 (−16.39) | |
Oceanic heat transport convergence | 13.56 | 18.29 (+4.73) | 17.62 (+4.06) | 13.67 (+0.11) |
Historical values are averaged over 1995–2014, whereas future projections are averaged over 2050–2099. Numbers in parentheses show differences in values from the historical simulation. Positive (negative) values mean there is a net incoming (outgoing) heat transport over the section. Despite the projected future heat transport decline of the EAUC with increasing greenhouse gas emission, all simulations show oceanic heat transport convergence over the target region (bottom row Table 1). This is also clear in the increasing cumulative oceanic heat fluxes over time (solid lines in Fig. 4a).
Area averages over the target region were calculated to diagnose changes to the temperature, salinity profile and water column stratification using mixed layer depths and Brunt–Väisälä frequency. Mixed layer depths were diagnosed as the depth where the potential density is 0.01 kg m–3 greater than the density at the surface. Lastly, model wind stress was assessed to quantify changes to wind-induced mixing over our target region.
The net heat and volume transport of the EAUC and ECC were determined by the maximum transport from integration of the heat and volume transport from the coast outwards for each time step. This approach accounts for the geographic shift of the current over time. As both currents are generally adjacent to the continental shelf, we assume the absence of a strong recirculation between the coast and the current.
Future changes to the above diagnostics were quantified over the period between 2050 and 2099 in comparison with the last 20 years of the historical simulation (1995–2014). Future MHW intensities (Fig. 1b–d and Fig. 2) were diagnosed using 5-day averaged SST data following the approach outlined in Behrens et al. (2022) by using a fixed baseline from 1982 to 2012. To demonstrate that MHW intensities are projected to become more severe with climate change and that this intensification is not solely driven by the long-term warming trend, a comparison was conducted where local linear trends were removed from the 5-daily averaged SST data for the individual analysed periods while keeping the fixed baseline period unchanged. Probability distributions were constructed for our target region and normalised so that the area under the curve equated to 1 for each probability distribution (Fig. 2). The probability distribution for MHW intensities with a fixed baseline and warming trend included (Fig. 2a) substantially changes shape in the future scenarios and implies a higher likelihood for more severe MHWs compared with the historical period. Even when the local linear temperature trends are removed, the probability of more severe MHWs is projected to increase in the future as indicated by the shift towards higher MHW intensities compared with the historical period (Fig. 2b). This MHW intensification provides the motivation for this study to further investigate the drivers for MHWs and how they change north-east of New Zealand.
3.Results
3.1. Heat sources north-east of NZ
The heat from subtropical waters that is transported to the EAUC through the TF is the main source of heat into the target region, shown by the large positive heat transport between 34 and 33°S over the western section (Fig. 3a and Table 1). Investigating changes to the inflow of heat helps us understand the projected changes in oceanic heat and MHWs in the region. Along the southern section, the southward heat transport associated with the ECC west of 180°E is apparent. The northern and eastern sections in Fig. 3 have been scaled by a factor of three so their weaker variability is visible. They show some interannual variability, but the persistent heat transport is not associated with any permanent oceanic currents. The volume and associated heat transport of the TF, which feeds into the EAUC, is projected to decrease owing to the spin-up and extension of the South Pacific gyre (Sen Gupta et al. 2021). Over the historical simulation of NZESM between 1950 and 2014, the net heat transport of the EAUC shows a long-term decline by ~8% per decade and the net heat transport of the ECC a decline by ~15% per decade. The trends of the heat and volume transport for the EAUC and the ECC are shown in Fig. 4a, b respectively. Overall, the future projections suggest an overall weaker volume and heat transport relative to the historical period, but that the historical strong trends ease. However, only the high emission scenario (SSP3–7.0) shows a further declining trend in both quantities for the EAUC, whereas the other scenarios suggest a positive trend from 2015 onward. For the EEC, all scenarios suggest a positive trend in volume and heat transport beyond 2015.
(a–d) Historical simulations of the top 1000 m oceanic heat transport over the four boundaries of the target region; (e–h) interannual oceanic heat transport anomalies for SSP3–7.0 relative to 1995–2014 of the historical simulation. (i–l) Mean oceanic heat transport anomalies for SSP1–2.6, SSP2–4.5, SSP3–7.0 for 2050–2099 relative to the historical simulation (1995–2014). Positive (negative) values in the heat transport in the historical simulation represent heat transported into (out of) the region. Positive (negative) anomalies represent an increase (decrease) in heat transport. Note the scaling factors for the northern and eastern boundary.
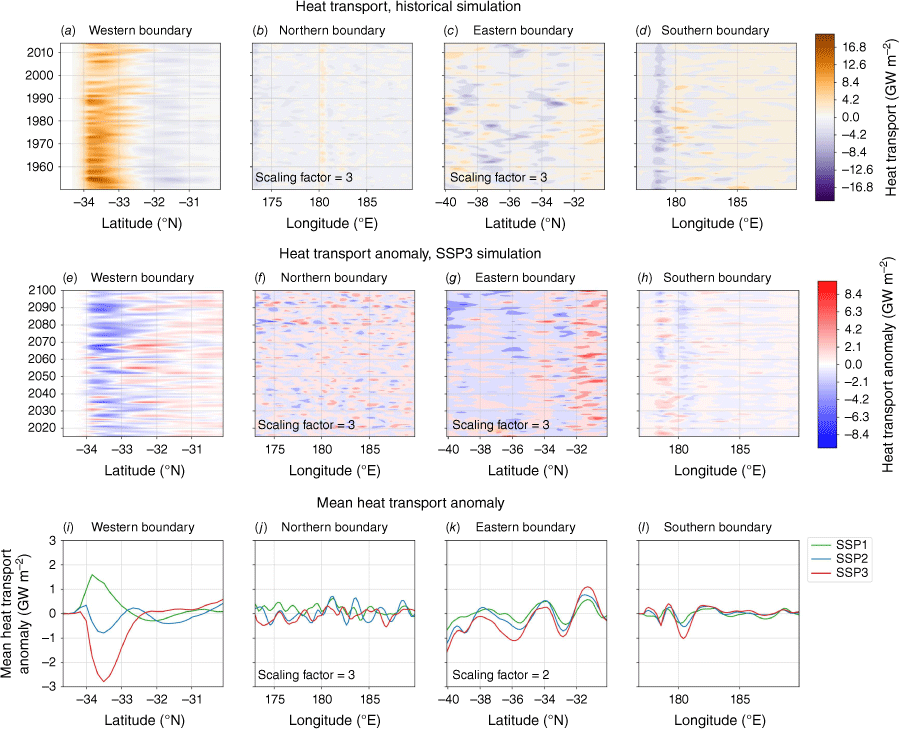
The annual mean heat transport and volume transport in Sverdrups (1 Sv = 106 m3 s–1) of (a), (c) the EAUC, and (b), (d) the ECC; the solid line represents the trend of heat transport in the second half of the century for the future scenarios and the dashed line over the period 2015–2099.
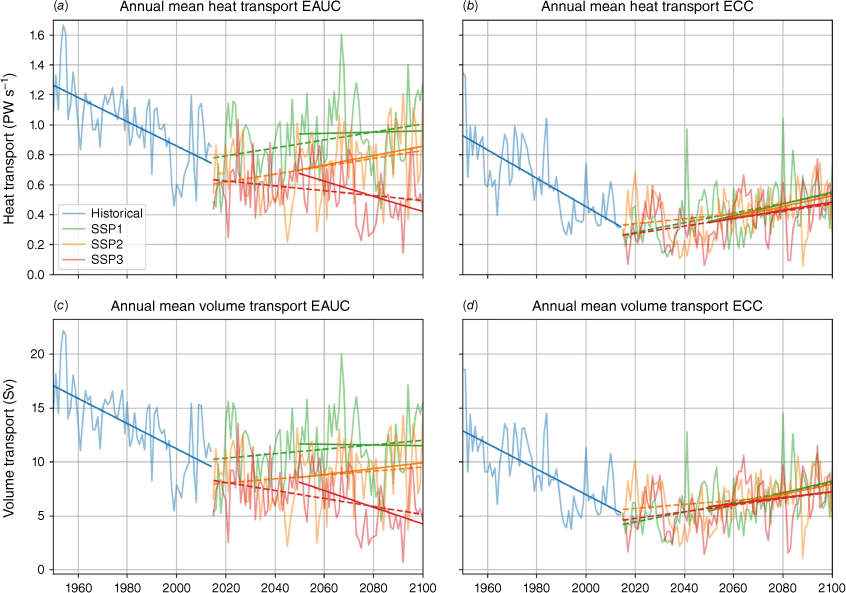
The mean historic (1995–2014) net heat transport (top 1000 m) across the four sections, West, South, East and North, is 556.4, −241.4, −137.6, −163.9 × 1012 W respectively (see Table 1), which indicates an oceanic heat convergence of 13.6 × 1012 W. This heat convergence has flow-on effects on the heat content, heat fluxes to the atmosphere and fluxes to the deep ocean. The heat loss into the deep ocean, below 1000 m, calculated with NZESM, is six orders of magnitude smaller than the oceanic heat transport across the boundaries and atmospheric heat losses. Hence, the heat flux to the deep ocean is considered negligible for the heat budget shown in Fig. 5b. In the SSP1–2.6, SSP2–4.5 and SSP3–7.0 scenarios, 72.9, 45.2 and 42.1% respectively of the heat transport convergence leads through the warming ocean to increased heat fluxes to the atmosphere, while the remaining 27.1, 54.8 and 57.8% in SSP1–2.6, SSP2–4.5 and SSP3–7.0 maintains the ongoing warming of this region. Less than 0.003% of the heat transport convergence gets transported to the deep ocean below 1000 m. The cumulative heat fluxes of SSP2–4.5 and SSP3–7.0 do not suggest a dependency on the climate changes scenario. Therefore, the heat fluxes, atmospheric and top 1000 m ocean heat transports in and out of the region alone cannot explain the different rates at which MHWs are projected to intensify.
(a) Top 1000-m cumulative heat fluxes (Φ) for future projections based on oceanic heat advection (solid), atmospheric fluxes (dashed) and advection into the deep ocean below 1000 m (dot–dash) for SSP1–2.6 (green), SSP2–4.5 (blue) and SSP3–7.0 (red). (b) Net heat content changes derived from the difference between oceanic and atmospheric heat fluxes from (a). Positive heat flux anomalies indicate oceanic heat transport convergence and an increase of ocean heat content over the target region.
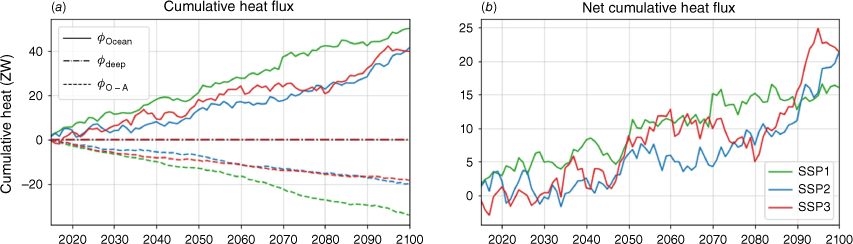
3.2. Heat transport changes
In this section, we examine how heat transport changes through the water column. Surface intensified warming has implications for temperature extremes, such as MHWs at the surface of the ocean in the euphotic zone. We focus on the primary inflow of heat across the western section (Fig. 1). Heat transports from the historical period show the surface-intensified flow of the EAUC next to the shelf of the North Island of New Zealand (Fig. 6a). The largest heat transport anomalies are observed within the top 250 m for all four sections.
Heat transport over all four sections surrounding the target region for mean historical (1995–2014) and future changes in SSP1–2.6, SSP2–4.5 and SSP3–7.0 (2050–2099) relative to the historical period: (a, e, i, m) Western; (b, f, j, n) Northern; (c, g, k, o) Eastern; and (d, h, l, p) Southern respectively. In the colour bar for the historical period, orange represents eastward and northward transport and purple represents westward and southward heat transport. The positive anomalies are increases in heat transport and negative anomalies decreases into and out of the study volume.
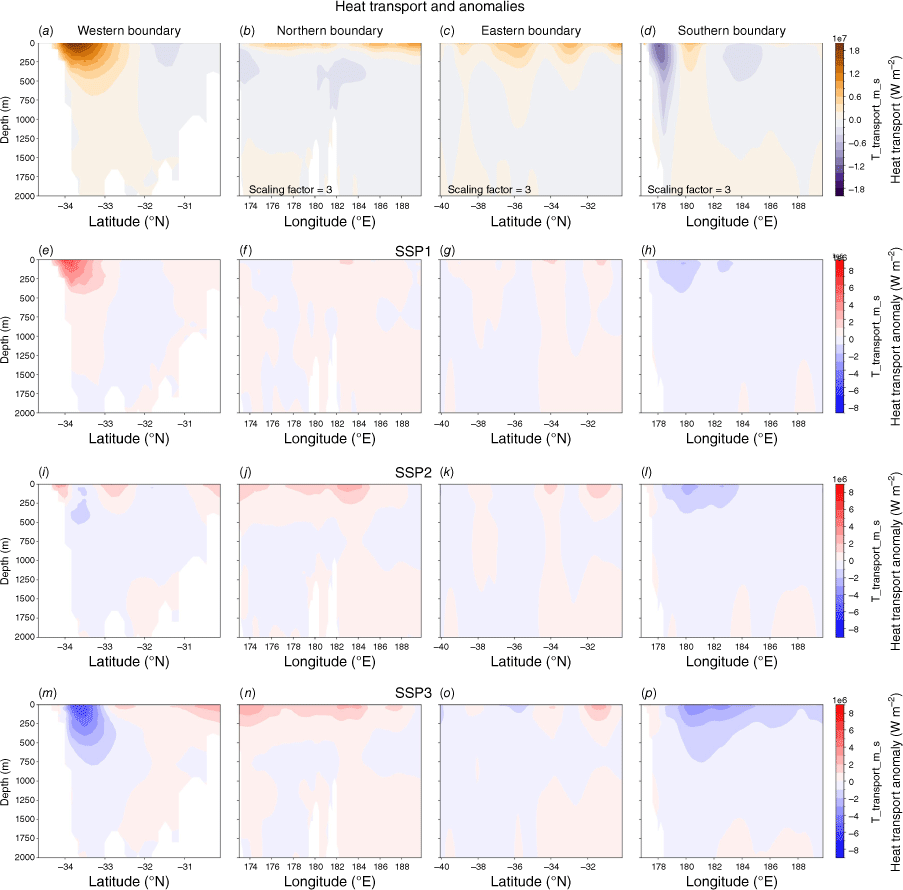
With the stronger greenhouse gas emission scenarios, the heat transport associated with the EAUC is projected to decline (Fig. 6e, i, m). Note in the SSP1–2.6 heat transports associated with the EAUC would be higher than the historical reference. The heat transport across the southern and eastern boundaries also weakens with increasing greenhouse gas emissions, while transport through the northern section increases. Integration of these anomalies reveals that declining EAUC heat transports are overcompensated by increased heat transport across the northern section with only mild declines across the southern section, leading to a heat transport convergence over this region in the top ~250 m. Please note that heat transports and projected changes are closely coupled to volume transports, with an r value between 0.98 and 0.99 and P values in the order of 10−70 for each region and future scenario.
After investigating the spatial variations of the lateral heat fluxes into the target region, we turn our attention to the area-averaged temperature profile to investigate the cumulative impact of changes in these lateral heat fluxes (Fig. 7). We observe an ongoing warming of the top 250 m in all SSPs, reaching ~2°C in SSP1–2.6 and ~4°C in SSP3–7.0 by the end of the century. This depth range represents surface and subtropical mode waters in this region (Fernandez et al. 2018). Below this layer, between 250 and 700 m, a cooling signal emerges, which is stronger in high emission scenarios.
Area-averaged temperature anomaly profiles over the target region for (a) SSP1–2.6, (b) SSP2–4.5, and (c) SSP3–7.0 (2050–2099) relative to the historical period (1995–2014). Stratification changes over the target region quantified by the Brunt–Väisälä frequency for (d) SSP1–2.6, (e) SSP2–4.5, and (f) SSP3–7.0 relative to the historical period (1995–2014).
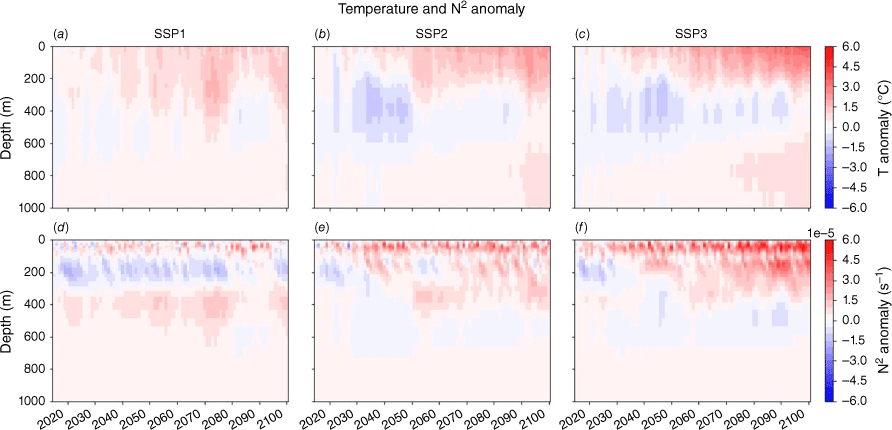
Changes in the temperature profile have impacts on the overall stratification in this region, which is shown in Fig. 7d–f. The stratification of the top 1000 m of the ocean is described by the Brunt–Väisälä frequency, which is a measure of vertical stability. The SSP1–2.6 scenario shows a weakening of the stratification over most of the century between 100 and 250 m, with positive anomalies above and below this depth. The stratification increases in the SSP2–4.5 scenario in the top 50 m in the first half of this century and down to 400 m in the second half of the century. The SSP3–7.0 scenario shows larger stratification changes than the lower emission scenarios; the stratification increases from 2030 in the top 100 m and extends down to 400 m in the second half of the century. Increased stratification in the upper layer of the ocean can trap heat near the surface, thereby intensifying the strength, duration and occurrence of MHWs (Oliver et al. 2021).
The historical simulation suggests annual mean mixed layer depths between 45 and 75 m over our target region for the historical simulation from 1995 to 2014, with deeper mixed layers to the south (Fig. 8a). The future projections show in Fig. 8b–d, these mixed layers becoming shallower by the end of the century by up to 15 m in the SSP2–4.5 scenario and up to 30 m in the SSP3–7.0 scenario. Negative anomalies mean the mixed layer depth is becoming shallower.
Mixed layer depths for (a) historical simulation (1995–2014), and anomalies in (b) SSP1–2.6, (c) SSP2–4.5, and (d) SSP3–7.0 over the period 2050–2099 relative to the historical period (1995–2014). Negative anomalies mean the mixed layer depth is becoming more shallow.
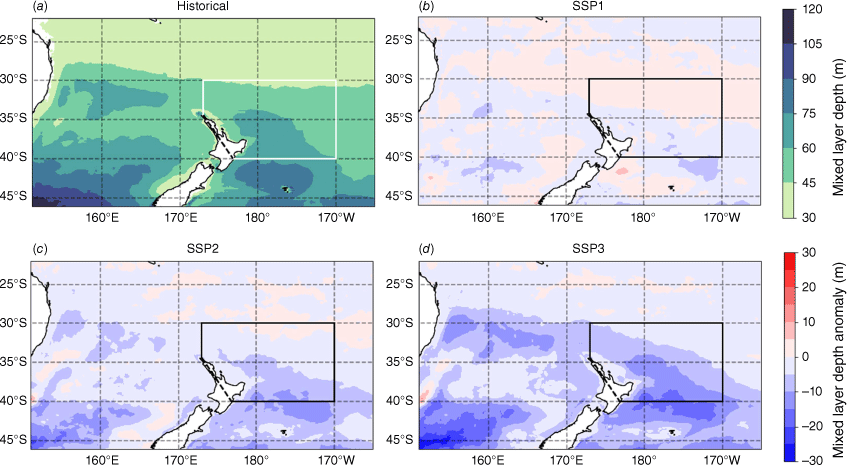
Finally, we investigate changes in wind stress because winds also influence oceanic stratification through turbulent mixing (Fig. 9). Stronger winds induce stronger mixing, which dissipates heat to deeper depths and prevents heat building up at the ocean surface. In the historical mean (Fig. 9a), we observe a band of low wind stress reaching from Australia to the north of New Zealand between 35 and 30°S. South of this region, wind stress increases. Future projections suggest a further decline in wind stress to the north and east of New Zealand, including over our target region. The pattern and magnitude of these anomalies is very similar between all three SSPs, which implies little sensitivity to greenhouse gas emissions. Ozone recovery is a possible cause for the changing wind pattern and is identical in each future scenario in NZESM. The projected wind decreases are consistent with the predicted future shoaling of the mixed layer (Fig. 8).
4.Discussion and summary
This study investigates the causes of increased MHW intensities north-east of New Zealand in future projections by the NZESM under rising greenhouse gas emissions. For the two stronger scenarios, there is agreement with other climate change projection studies (e.g. Sen Gupta et al. 2021); the volume and therefore the oceanic heat transport of the TF and EAUC are reduced in these NZESM SSP2–4.5 and SSP3–7.0 simulations, decreasing a major heat source to this region. A heat content budget was conducted to disentangle the various heat sources to this region and gain insight as to why MHWs are projected to intensify. These analyses revealed that the top 1000-m cumulative heat content will further increase with increasing greenhouse gas emissions, despite a decline in the heat transport associated with the EAUC in the SSP2–4.5 and SSP3–7.0 scenarios compared with the historical mean. The reduced heat transport from the tropics, through the Tasman Front and EAUC, is overcompensated by a reduced outflow of meridional heat transports through the northern and southern sections.
Our results reveal that the heat content convergence, shown in Fig. 5, due to ocean advection is not fully compensated by elevated atmospheric heat fluxes, which leads to an overall increase in heat content over this region. In Fig. 7, we show that the largest heat content increase is concentrated in the top 250 m and cause SSTs to warm in agreement with climate projections from other Earth System models (Rickard et al. 2023). The layer from 250 to 700 m is projected to show a decline in heat content due to reduced heat transport through the EAUC. The consequence of heat content increase in the top 250 m is a projected increase in stratification over the top 400 m of the water column and for mixed layer depths to decline by up to 30 m over the second half of the century in high greenhouse gas emission scenarios. Shallower future mixed layers have also been reported in this region from other climate models (Rickard et al. 2023).
A stronger surface stratification is conducive to the development of MHWs at the surface, as surface heating is distributed over a smaller volume, leading to greater warming. In addition to the oceanic heat transport changes, the future projections also indicate a reduction of surface wind stress north of New Zealand, reducing mechanical mixing, which again increases the likelihood of MHWs as vertical heat transport is reduced with slower winds. Interestingly, the projected reductions in surface wind stresses show little sensitivity to greenhouse gas emissions. The very similar decline in wind stress between all SSPs potentially suggests that the frequency of blocking high pressure systems will not change in the future and therefore not substantially alter characteristics of MHWs. Based on the presented results, we can conclude that with climate change, MHWs projected in NZESM will generally intensify owing to an increase in stratification. The decrease in wind and surface intensified heating were found to be key elements in maintaining MHWs.
Although NZESM, owing to its fine model mesh around New Zealand, shows smaller model biases in temperatures and salinity compared with UKESM (Behrens et al. 2020), the remaining model biases are not zero and affect MHWs metrics in historical and future projections. Previous research has shown that NZESM overestimates MHWs intensities by ~0.5°C around the North Island, whereas MHW days show a negative bias of ~25 days per year in this region (Behrens et al. 2022). Ongoing research has also identified shortcomings in NZESM in the placement of the Subtropical Front, which affects MHWs in historical and future simulations around the South Island (Roach et al. 2024). Although biases in temperature have a direct effect on MHWs, our research has demonstrated that the underlying shape of probability distributions for MHW intensities is projected to change in the future and cannot be explained just by a constant warming, which would result in a shift of the probability distribution to higher intensities while the shape of the distribution remained the same, as shown in Fig. 2. In fact, in future projections of NZESM, the probability distribution widens and shifts, implying that the likelihood for more intense MHWs increases disproportionally. This suggests changes to the drivers of MHWs beyond an overall warming due to climate change.
Data availability
The raw data supporting the conclusions of this article will be made available by the authors, without undue reservation.
Declaration of funding
All authors obtained funding from New Zealand Ministry for Business Innovation and Employment by CO1X1902 Deep South National Science Challenge and the New Zealand Ministry of Business, Innovation and Employment NIWA Strategic Investment Funding OCOC2504 to conduct this research.
Acknowledgements
We acknowledge the support of NeSI High Performance Computing Facility and its team. In addition, we acknowledge those involved in the development of UKESM. We also thank Graham Rickard and Craig Stevens for their support to make this project possible. Finally, L. Cornelissen acknowledges her partner Russell and dog Tasman. We also thank both reviewers for their constructive feedback during the review process, which helped us improve this manuscript.
Author contributions
L. Cornelissen performed the analysis. E. Behrens and L. Cornelissen compiled the paper draft. D. Fernandez and P. Sutton provided feedback on the paper draft and diagnostics. All authors contributed to and approved the submitted version.
References
Anderson O, Stephenson F, Behrens E (2020) Updated habitat suitability modelling for protected corals in New Zealand waters. NIWA client report 2020174WN, prepared for Department of Conservation (DOC). (National Institute of Water & Atmospheric Research: Wellington, New Zealand) Available at https://www.doc.govt.nz/globalassets/documents/conservation/marine-and-coastal/marine-conservation-services/reports/pre-2019-annual-plans/pop2018-01-coral-habitat-suitability-final-report.pdf
Anderson OF, Stephenson F, Behrens E, Rowden AA (2022) Predicting the effects of climate change on deep‐water coral distribution around New Zealand – will there be suitable refuges for protection at the end of the 21st Century? Global Change Biology 28(22), 6556-6576.
| Crossref | Google Scholar | PubMed |
Behrens E, Fernandez D, Sutton P (2019) Meridional oceanic heat transport influences marine heatwaves in the Tasman Sea on interannual to decadal timescales. Frontiers in Marine Science 6, 228.
| Crossref | Google Scholar |
Behrens E, Williams J, Morgenstern O, Sutton P, Rickard G, Williams MJM (2020) Local grid refinement in New Zealand’s Earth System Model: Tasman Sea ocean circulation improvements and super-gyre circulation implications. Journal of Advances in Modelling Earth Systems 12(7), e2019MS001996.
| Crossref | Google Scholar |
Behrens E, Rickard G, Rosier S, Williams J, Morgenstern O, Stone D (2022) Projections of future marine heatwaves for the oceans around New Zealand using New Zealand’s Earth System Model. Frontiers in Climate 4, 798287.
| Crossref | Google Scholar |
Bodeker G, Tait A, Morgenstern O, Noone D, Revell L, McDonald A, et al. (2022) Aotearoa New Zealand climate change projections guidance: interpreting the latest IPCC WG1 report findings. Prepared for the Ministry for the Environment, Report number CR 501. (University of Canterbury) Available at https://ir.canterbury.ac.nz/server/api/core/bitstreams/294aea35-9bc1-4a4f-ba4a-2667e31ef3dc/content
Bogers-Cornelissen L (2022) Currents in the Tasman Sea in a changing climate. MClimatePhys thesis, Utrecht University, Utrecht, Netherlands. Available at https://studenttheses.uu.nl/handle/20.500.12932/43304
Cheng L, Zhu J, Abraham J, Trenberth KE, Fasullo JT, Zhang B, Yu F, Wan L, Chen X, Song X (2019) 2018 Continues record global ocean warming. Advances in Atmospheric Sciences 36(3), 249-252.
| Crossref | Google Scholar |
Chiswell SM, Bostock HC, Sutton PJ, Williams MJ (2015) Physical oceanography of the deep seas around New Zealand: a review. New Zealand Journal of Marine and Freshwater Research 49(2), 286-317.
| Crossref | Google Scholar |
Debreu L, Vouland C, Blayo E (2008) AGRIF: adaptive grid refinement in Fortran. Computers & Geosciences 34(1), 8-13.
| Crossref | Google Scholar |
Gregory CH, Holbrook NJ, Marshall AG, Spillman CM (2023) Atmospheric drivers of Tasman Sea marine heatwaves. Journal of Climate 36, 5197-5214.
| Crossref | Google Scholar |
Fernandez D, Bowen M, Sutton P (2018) Variability, coherence and forcing mechanisms in the New Zealand ocean boundary currents. Progress in Oceanography 165, 168-188.
| Crossref | Google Scholar |
Hill KL, Rintoul SR, Ridgway KR, Oke PR (2011) Decadal changes in the South Pacific western boundary current system revealed in observations and ocean state estimates. Journal of Geophysical Research Atmospheres 116(C1), C01009.
| Crossref | Google Scholar |
Hobday AJ, Alexander LV, Perkins SE, Smale DA, Straub SC, Oliver ECJ, et al. (2016) A hierarchical approach to defining marine heatwaves. Progress in Oceanography 141, 227-238.
| Crossref | Google Scholar |
Hobday AJ, Oliver EC, Gupta AS, Benthuysen JA, Burrows MT, Donat MG, et al. (2018) Categorizing and naming marine heatwaves. Oceanography 31(2), 162-173.
| Crossref | Google Scholar |
Holbrook NJ, Sen Gupta A, Oliver ECJ, Hobday AJ, Benthuysen JA, Scannell HA, et al. (2020) Keeping pace with marine heatwaves. Nature Reviews Earth & Environment 1(9), 482-493.
| Crossref | Google Scholar |
Huang B, Menne MJ, Boyer T, Freeman E, Gleason BE, Lawrimore JH, et al. (2020) Uncertainty estimates for sea surface temperature and land surface air temperature in NOAAGlobalTemp version 5. Journal of Climate 33(4), 1351-1379.
| Crossref | Google Scholar |
Hunke E, Lipscomb W, Jones P, Turner A, Jeffery N, Elliott S (2017) CICE, The Los Alamos Sea Ice Model. CICE: 005315WKSTN00. (US Department of Energy, Office of Scientific and Technical Information) Available at https://www.osti.gov/biblio/1364126
Johnson GC, Lyman JM (2020) Warming trends increasingly dominate global ocean. Nature Climate Change 10(8), 757-761.
| Crossref | Google Scholar |
Kerry C, Roughan M, Azevedo Correia de Souza JM (2022) Drivers of upper ocean heat content extremes around New Zealand revealed by Adjoint Sensitivity Analysis. Frontiers in Climate 4, 980990.
| Crossref | Google Scholar |
Kuhlbrodt T, Jones CG, Sellar A, Storkey D, Blockley E, Stringer M, et al. (2018) The low‐resolution version of HadGEM3 GC3.1: development and evaluation for global climate. Journal of Advances in Modeling Earth Systems 10(11), 2865-2888.
| Crossref | Google Scholar | PubMed |
Madec G, Bourdallé-Badie R, Bouttier P-A, Bricaud C, Bruciaferri D, Calvert D, et al. (2024) NEMO ocean engine. Zenodo 2017, v3.6-patch, number 27 [Software documentation].
| Crossref | Google Scholar |
Meinshausen M, Nicholls ZR, Lewis J, Gidden MJ, Vogel E, Freund M, et al. (2020) The shared socio-economic pathway (SSP) greenhouse gas concentrations and their extensions to 2500. Geoscientific Model Development 13(8), 3571-3605.
| Crossref | Google Scholar |
Montie S, Thoral F, Smith RO, Cook F, Tait LW, Pinkerton MH, et al. (2023) Seasonal trends in marine heatwaves highlight vulnerable coastal ecoregions and historic change points in New Zealand. New Zealand Journal of Marine and Freshwater Research 58, 276-299.
| Crossref | Google Scholar |
Oke PR, Pilo GS, Ridgway K, Kiss A, Rykova T (2019) A search for the Tasman Front. Journal of Marine Systems 199, 103217.
| Crossref | Google Scholar |
Oliver EC, Benthuysen JA, Darmaraki S, Donat MG, Hobday AJ, Holbrook NJ, Schlegel RW, Gupta AS (2021) Marine heatwaves. Annual Review of Marine Science 13(1), 313-342.
| Crossref | Google Scholar | PubMed |
Rae JGL, Hewitt HT, Keen AB, Ridley JK, West AE, Harris CM, et al. (2015) Development of the Global Sea Ice 6.0 CICE configuration for the Met Office Global Coupled model. Geoscientific Model Development 8(7), 2221-2230.
| Crossref | Google Scholar |
Rickard GJ, Behrens E, Chiswell S, Law CS, Pinkerton MH (2023) Biogeochemical and physical assessment of CMIP5 and CMIP6 Ocean Components for the southwest Pacific Ocean. Journal of Geophysical Research: Biogeosciences 128, e2022JG007123.
| Crossref | Google Scholar |
Ridgway K, Dunn J (2003) Mesoscale structure of the mean East Australian Current System and its relationship with topography. Progress in Oceanography 56(2), 189-222.
| Crossref | Google Scholar |
Roach CJ, de Souza JMAC, Behrens E, Stuart SJ (2024) Moana Ocean Future Climate V1.0: high resolution marine climate futures for the New Zealand Region. EGUsphere 2024, egusphere-2024-1962 [Preprint, published 30 October 2024].
| Crossref | Google Scholar |
Salinger J, Renwick J, Behrens E, Mullan B, Diamond HJ, Sirguey P, et al. (2019) The unprecedented coupled ocean–atmosphere summer heatwave in the New Zealand region 2017/18: drivers, mechanisms and impacts. Environmental Research Letters 14, 044023.
| Crossref | Google Scholar |
Salinger MJ, Diamond HJ, Behrens E, Fernandez D, Fitzharris BB, Herold N, et al. (2020) Unparalleled coupled ocean–atmosphere summer heatwaves in the New Zealand region: drivers, mechanisms and impacts. Climatic Change 162(2), 485-506.
| Crossref | Google Scholar |
Salinger MJ, Diamond HJ, Bell J, Behrens E, Fitzharris BB, Herod N, et al. (2023) Coupled ocean–atmosphere summer heatwaves in the New Zealand region: an update. Weather and Climate 42(1), 18-41.
| Crossref | Google Scholar |
Salinger MJ, Trenberth KE, Diamond HJ, Behrens E, Fitzharris BB, Herold N, Smith RO, Sutton PJ, Trought MCT (2024) Climate extremes in the New Zealand region: mechanisms, impacts and attribution. International Journal of Climatology 44, 5809-5824.
| Crossref | Google Scholar |
Sellar A, Jones C, Mulcahy J, Tang Y, Yool A, Wiltshire A, O’Connor FM, Stringer M, Hill RS, Palmiéri J, Woodward S, De Mora L, Kuhlbrodt T, Rumbold ST, Kelley DI, Ellis R, Johnson CE, Walton J, Abraham NL, et al. (2019) UKESM1: description and evaluation of the UK Earth System Model. Journal of Advances in Modeling Earth Systems 11(12), 4513-4558.
| Crossref | Google Scholar |
Sen Gupta A, Stellema A, Pontes GM, Taschetto AS, Vergés A, Rossi V (2021) Future changes to the upper ocean Western Boundary Currents across two generations of climate models. Scientific Reports 11(1), 9538.
| Crossref | Google Scholar | PubMed |
Smale DA, Wernberg T, Oliver EC, Thomsen M, Harvey BP, Straub SC, et al. (2019) Marine heatwaves threaten global biodiversity and the provision of ecosystem services. Nature Climate Change 9(4), 306-312.
| Crossref | Google Scholar |
Smith KE, Burrows MT, Hobday AJ, Sen Gupta A, Moore PJ, Thomsen M, et al. (2021) Socioeconomic impacts of marine heatwaves: global issues and opportunities. Science 374(6566), eabj3593.
| Crossref | Google Scholar | PubMed |
Stephenson F, Rowden AA, Anderson OF, Ellis JI, Geange SW, Brough T, Behrens E, Hewitt JE, Clark MR, Tracey DM, Goode SL, Petersen GL, Lundquist CJ (2023) Implications for the conservation of deep-water corals in the face of multiple stressors: a case study from the New Zealand region. Journal of Environmental Management 346, 118938.
| Crossref | Google Scholar | PubMed |
Storkey D, Blaker AT, Mathiot P, Megann A, Aksenov Y, Blockley EW, et al. (2018) UK Global Ocean GO6 and GO7: a traceable hierarchy of model resolutions. Geoscientific Model Development 11(8), 3187-3213.
| Crossref | Google Scholar |
Storto A, Yang C, Masina S (2017) Constraining the global ocean heat content through assimilation of CERES‐derived TOA energy imbalance estimates. Geophysical Research Letters 44(20), 10,520-10,529.
| Crossref | Google Scholar |
Sung HM, Kim J, Lee J-H, Shim S, Boo K-O, Ha J-C, Kim Y-H (2021) Future changes in the global and regional sea level rise and sea surface temperature based on CMIP6 models. Atmosphere 12(1), 90.
| Crossref | Google Scholar |
Sutton PJH, Bowen M (2019) Ocean temperature change around New Zealand over the last 36 years. New Zealand Journal of Marine and Freshwater Research 53(3), 305-326.
| Crossref | Google Scholar |
Sutton PJH, Rickard GJ, Roemmich DH (2024) Southwest Pacific Ocean warming driven by circulation changes. Geophysical Research Letters 51(13), e2024GL109174.
| Crossref | Google Scholar |
Von Schuckmann K, Cheng L, Palmer MD, Hansen JE, Tassone C, Aich V, Adusumilli S, Beltrami H, Boyer T, Cuesta‐Valero FJ, Desbruyères D, Domingues CM, García‐García A, Gentine P, Gilson J, Gorfer M, Haimberger L, Ishii M, Johnson GC, Wijffels S (2020) Heat stored in the Earth system: where does the energy go? Earth System Science Data 12(3), 2013-2041.
| Crossref | Google Scholar |
Walters D, Baran AJ, Boutle I, Brooks M, Earnshaw P, Edwards J, et al. (2019) The Met Office Unified Model Global Atmosphere 7.0/7.1 and JULES Global Land 7.0 configurations. Geoscientific Model Development 12(5), 1909-1963.
| Crossref | Google Scholar |
Williams J, Morgenstern O, Varma V, Behrens E, Hayek W, Oliver H, et al. (2016) Development of the New Zealand Earth System Model: NZESM. Weather and Climate 36, 25-44.
| Crossref | Google Scholar |
Wu L, Cai W, Zhang L, Nakamura H, Timmermann A, Joyce T, et al. (2012) Enhanced warming over the global subtropical western boundary currents. Nature Climate Change 2(3), 161-166.
| Crossref | Google Scholar |
Yang H, Lohmann G, Wei W, Dima M, Ionita M, Liu J (2016) Intensification and poleward shift of subtropical western boundary currents in a warming climate. Journal of Geophysical Research: Oceans 121(7), 4928-4945.
| Crossref | Google Scholar |
Yool A, Popova EE, Anderson TR (2013) MEDUSA-2.0: an intermediate complexity biogeochemical model of the marine carbon cycle for climate change and ocean acidification studies. Geoscientific Model Development 6(5), 1767-1811.
| Crossref | Google Scholar |