What is recalcitrant soil organic matter?
Markus KleberDepartment of Crop and Soil Science, Oregon State University, 3017 Agriculture and Life Sciences Building, Corvallis, OR 97331, USA. Email: markus.kleber@oregonstate.edu
Environmental Chemistry 7(4) 320-332 https://doi.org/10.1071/EN10006
Submitted: 20 January 2010 Accepted: 1 June 2010 Published: 20 August 2010
Environmental context. On a global scale, soils store more carbon than plants or the atmosphere. The cycling of this vast reservoir of reduced carbon is closely tied to variations in environmental conditions, but robust predictions of climate–carbon cycle feedbacks are hampered by a lack of mechanistic knowledge regarding the sensitivity of organic matter decomposition to rising temperatures. This text provides a critical discussion of the practice to conceptualise parts of soil organic matter as intrinsically resistant to decomposition or ‘recalcitrant’.
Abstract. The understanding that some natural organic molecules can resist microbial decomposition because of certain molecular properties forms the basis of the biogeochemical paradigm of ‘intrinsic recalcitrance’. In this concept paper I argue that recalcitrance is an indeterminate abstraction whose semantic vagueness encumbers research on terrestrial carbon cycling. Consequently, it appears to be advantageous to view the perceived ‘inherent resistance’ to decomposition of some forms of organic matter not as a material property, but as a logistical problem constrained by (i) microbial ecology; (ii) enzyme kinetics; (iii) environmental drivers; and (iv) matrix protection. A consequence of this view would be that the frequently observed temperature sensitivity of the decomposition of organic matter must result from factors other than intrinsic molecular recalcitrance.
Introduction
Estimates for the amount of global soil carbon (0–300 cm) have recently been raised from a previous 2344 pg[1] to a new datum of >3300 pg.[2] It is not well understood how this vast amount of carbon will respond to environmental change. Models that have coupled climate and carbon cycles[3] show a large divergence in the size of the predicted feedback[4–6] between enhanced decomposition of biospheric carbon pools and the heat content of the atmosphere. One of the issues that remains to be resolved for a more mechanistic understanding of carbon-cycle climate feedbacks is the intensely disputed[7–12] temperature sensitivity of soil organic matter decomposition. Of particular concern is the sensitivity of the resistant pools of organic matter,[13] which are often synonymously referred to as stable, recalcitrant or ‘old’.[9]
Compounds that are classified as recalcitrant or ‘stable’ are assumed to comprise a much greater proportion of the total soil carbon pool than those that are classified as labile.[14] Theoretical models predict greater sensitivity of recalcitrant compounds to rising temperatures,[14,15] but empirical studies do not equivocally support this prediction.[7,16] Laboratory incubations tend to show a strong temperature response of recalcitrant organic matter, whereas field studies show either a minor temperature response or one that quickly re-equilibrates at a low level.[17] Part of the problem may be that a large variety of mechanistically independent interpretations of the terms ‘recalcitrance’ and ‘inherent stability’ can be encountered in the literature (Table 1). Some authors, for example, understand recalcitrance as a material property[15,18–21] based on molecular structure (Table 1, item I1); another approach (Table 1, item I2) considers ‘old’ organic compounds that have achieved long mean residence time as recalcitrant[14,16,22] and a third view defines recalcitrant organic matter operationally as such that is decomposed during the later stages of an incubation experiment[23,24] (Table 1, item III1).
![]() |
It is widely accepted within the scientific community that there is an easy logic that ties these three examples of recalcitrance together in a convincing causal relationship. This logic assumes that (i) if a compound had a certain molecular property like very low aqueous solubility or a high proportion of polycondensed aromatic moieties, then the compound would not be decomposed during the early stages of a hypothetical incubation experiment (ii) and would as a consequence achieve a long residence time (iii) in the system.
But there are issues with this easy logic. For example, age is a property that is physically independent of molecular composition. This can be illustrated by putting a perishable food item like ice cream in a freezer. Freezing does not change the molecular structure of ice cream, so when the ice cream is taken out of the freezer on day zero + X, it has aged but it still has the same disposition of becoming decomposed as on day zero. Similarly, the fact that OM persists in the presence of decomposer organisms does not automatically mean that it has resisted decomposition. It can, at least theoretically, have been left behind because the decomposers deliberately choose not to decompose it. A potato left on the plate after a family dinner is not left uneaten because it has been able to resist the onslaught of the hungry dinner participants, its persistence is the result of a decision triggered by food-saturation, which was independent of the molecular structure of the potato.
The scientific community is aware of the existence of mechanisms other than recalcitrance that can protect organic matter against decomposition.[25] Mechanisms of organic matter protection like sorptive interactions with minerals and physical separation from enzymes and decomposers[26] are treated as independent of the intrinsic chemical properties of organic carbon and are not considered here. Within the context of this paper, the term ‘recalcitrance’ and its many synonyms such as ‘resistant carbon’ or ‘refractory organic matter’ are understood in a material property sense and do not refer to carbon that has achieved long turnover time for reasons that are not related to its chemical composition, that is, because it was protected against decomposition by physical isolation from or through interactions with its environmental matrix.
There appear to be serious concerns that a large proportion of organic matter in soils may have chemical properties, operationally termed recalcitrance, which render it particularly sensitive to feedbacks initiated by the expected rise of heat content within the atmosphere. The carbon quality-temperature (CQT) theory of the temperature sensitivity of organic matter decomposition links the temperature sensitivity of ‘recalcitrant’ organic carbon to the increase in activation energy that can theoretically be expected when molecular structures become more complex.[14,15] The classical Humic Polymer Model of soil organic matter[27] explains recalcitrance as the consequence of humification processes, which are thought to create large, covalently bonded humic polymers.[28,29] But if soil organic matter fragments are not large, complex, polymeric humic macromolecules[30,31] and are therefore not intrinsically recalcitrant, there is no longer a molecular justifcation for the assumption of complexity needed by the CQT theory to explain higher activation energies and the resulting greater temperature sensitivity of recalcitrant materials.
Attempts to clarify the issue and to predict the response of recalcitrant organic matter to climate change are confounded by a large uncertainty about the precise physical and chemical determinants for the phenomenon of recalcitrance and by the semantic indeterminacy of the category ‘recalcitrance’. It recently has been suggested that contradictory evidence may result, in part, from a large ambiguity and a lack of conceptual rigidity within the complex problem space of organic matter decomposition.[17] It appears therefore that continuing the practice of classifying organic matter as recalcitrant may adversely affect progress in many areas, including the temperature sensitivity of decomposition, carbon-cycle climate feedbacks, carbon turnover models and agricultural soil management. For example, it has been suggested to sequester atmospheric CO2 in soils by increasing the proportion of recalcitrant aliphatic compounds in soil organic matter.[32] This would be a questionable strategy if aliphatic compounds cannot be classified as recalcitrant, as some recent experimental work suggests.[33,34] Consequently, the objective of this manuscript is to highlight the lack of mechanistic depth of the concept of recalcitrance, invite the reader to step outside the conceptual box of recalcitrance and to point at alternative ways of incorporating information about the molecular structure of organic matter into representations of soil carbon turnover dynamics.
Background and relevance
Terminology
The multitude of non-numerical expressions used to convey the presumed ability of organic materials to resist microbial decomposition illustrates the difficulty to constrain a characteristic, which traditionally is thought to be of great consequence for the fate of organic carbon in the environment. Historically, categories like ‘molecular recalcitrance’,[35] ‘intrinsic chemical recalcitrance’,[19] or simply ‘resistant’ v. ‘labile’ carbon[8] have been used to suggest that some organic materials in the biosphere may have a greater ability than do others to resist microbial attack.[21] The widespread use of these terms indicates a broad scientific consensus that one or more material properties of natural organic carbon compounds can prevent the compound from being decomposed. This assumption, the basis of general theories to explain the turnover of soil organic matter,[25,27,36] is used as a control on carbon turnover time in models of soil carbon turnover,[37,38] has been used to describe certain kinds of marine dissolved organic matter[39,40] and has informed research on mobile forms of refractory organic carbon in the terrestrial environment.[41]
When scanning the large amount of literature on ‘recalcitrance’, the first issue is the absence of standardised use of the concept. For example, the terms ‘recalcitrant organic matter’ and ‘refractory organic matter’ are often used synonymously in biogeochemistry. However, whereas there is semantic overlap between the two, the dictionary indicates an important difference in the conventions of use. The term recalcitrance has a more behavioural connotation (resistant in the sense of active opposition), whereas the term refractory implies an acquired material property (resistant in the sense of being immune, especially, being resistant to heat, see ‘recalcitrant’ at www.merriam-webster.com/dictionary/recalcitrant and ‘refractory’ at www.merriam-webster.com/dictionary/refractory, both accessed 28 June 2010). The latter convention has made its way into the Standard Terminology chosen by the American Society for Testing and Materials (ASTM). The ASTM C71 standard[42] defines refractory materials as ‘non-metallic materials having those chemical and physical properties that made them applicable for structures, or as components of systems, that are exposed to environments above 1000°F (811 K; 538°C)’.
The term ‘refractory’ appears to be favoured by that part of the community of biogeochemists who work with thermally altered forms of organic matter like charcoal, but it also is encountered in situations where plain resistance to microbial decomposition is discussed.[43] Recalcitrance has been made popular as a concept in soil microbiology in the early 1960s by Alexander,[35,44,45] who defined the term as ‘a stubbornness on the part of specific molecules to succumb to microbiological attack’.[35] This early view has been refined to ‘Recalcitrance comprises molecular-level characteristics of organic substances including elemental composition, presence of functional groups, and molecular conformation, that influence their degradation by microbes and enzymes’,[19] implying that there are certain material properties of organic molecules that allow them to actively resist microbial degradation. More recently it has been posited that recalcitrance mainly accounts for protection on shorter time scales[46] and that it may be necessary to distinguish between ‘primary recalcitrance of plant litter and rhizodeposits as a function of their indigenous molecular characteristics, and the secondary recalcitrance of microbial products, humic polymers and charred materials’.[26] Besides revealing the struggle to find an adequate denominator for an enigmatic phenomenon, the non-numerical character of this latter definition also illustrates the strong contrast between the ‘hard’ formalisms (known algorithms) of physical chemistry and related science disciplines[17] and the ‘soft’ semantic category of recalcitrance, which lacks specifity and cannot easily be expressed using numerical categories.
Recalcitrance as a mechanism of carbon stabilisation in soils
Recalcitrance has long been accepted as one of the major mechanisms involved in the persistence of organic matter in soils, next to interactions,[19] accessibility,[19] climatic stabilisation[25] and facultative non-utilisation by the decomposer community.[47,48] Some authors even determined that chemical recalcitrance of largely pyrogenic carbon was the only mechanism in their soils that could account for long turnover times of organic matter.[36] This understanding is reflected in current soil carbon turnover models[38,49] in which organic matter has been conceptualised as consisting of several functionally homogeneous compartments decomposing at different rates following first-order kinetics. Decomposition rates decrease continuously with time, with ∼10% of organic matter remaining after a decade while some organic matter remains in soil for greater than millennia.[50] The supposition that the more carbon ages in a soil, the more resistant against decomposition it becomes (‘new humus decomposes faster than old humus’[51]) is an important characteristic of carbon turnover modelling concepts. A decrease in decomposition rate with time is accounted for in some models by the depletion of fast-decomposing pools and transfer of organic matter into pools with lower rate constants.[38,49] Other modelling approaches allow the rate of decomposition to decrease with time as a function of decreasing substrate quality[52] or as an empirical relationship.[53]
The decrease in rate of decomposition over time is frequently viewed as resulting from either (i) the combined effects of the gradual incorporation of carbon into the protective structural fabric of soils and sediments (interactions and accessibility in the model of Sollins et al.[19]); from (ii) biotic and abiotic syntheses processes that fuse decomposition products into more recalcitrant organic materials (humification or biochemical protection in the model of Six et al.[54]) or as being caused by (iii) preferential decomposition of more palatable compounds (‘selective degradation’ in the model of Sollins et al.[19]). Whereas mechanism (i) is independent of the molecular properties of organic matter, mechanisms (ii) and (iii) rely on the supposition that some forms of organic matter are able to withstand decomposition based on their chemical structure.
Recalcitrance and litter quality
The plant litter available to the decomposer community encompasses a broad range of tissues that differ in chemical and physical properties.[55] Decay rates of different plant organs reflect this diversity: fruits decompose faster than leaves, which in turn decompose much faster than woody plant parts.[55,56] The reader is referred to the review of Sanderman and Amundson[57] for references to a wealth of studies illustrating the point that the chemical composition of plants plays a role in determining the decomposition rate. This observation is often articulated by calling woody plant parts more recalcitrant, following the notion that materials with slow turnover behave in this way because they are able to resist decomposition.
Generally, tissues with higher lignin, polyphenol and wax contents and higher lignin : N and C : N ratios decompose more slowly. Aber et al.[58] found that the ratio of lignin : cellulose (lignin–cellulose index, LCI) was a good predictor of the decomposition rate k of plant litter, and substrate quality measured as lignin content was the primary control on the decomposition rate of plant litter in coniferous forests.[59] But lignin content was not a major factor in a study of global patterns of root decomposition, where Silver and Miya[60] found that calcium concentrations and C : N ratios of root materials together explained 89% of the variability of decomposition rates in a multiple linear regression. It is not plausible that lignin should be a control on the aboveground decomposition of plant litters but have no influence on the belowground decomposition of roots. If methodological difficulties of lignin analysis[61] are ruled out as an explanation for contradictions of this kind, then there must be other system properties with the ability to override lignin content as a ‘quality control’ on decomposition. It can be summarised that the chemical composition of litter unquestionably influences its decomposition rate; it does not do so because it renders the substrate chemically invulnerable, it merely determines the complexity of the decomposition operation. To an extent this was recognised in a recent analyses of the relevance of recalcitrance for carbon turnover in soils, which posited that ‘molecular recalcitrance of natural OM is not absolute, but relative’.[26] The same study proposed to differentiate between primary and secondary recalcitrance – the former refering to the influence of indigeneous molecular characteristics on variations in turnover time of plant litter and rhizodeposits, the latter comprises products of secondary microbial syntheses, humification processes and thermally altered materials.[26]
Recalcitrance and molecular complexity
The idea that some molecular characteristics of organic matter, especially its molecular complexity and degree of polymerisation, should be linked to its susceptibility to decomposition can be traced back to the early decades of the 20th century[62] and received strong support from the work of Martin et al.[63–66] These scientists introduced 14C into the molecular structure of phenolic acids and artificially prepared phenolic polymers. This allowed them to identify the molecular origin of the carbon in the 14CO2 that evolved during decomposition of these molecules. In a seminal publication, Haider and Martin[65] showed how linkage into polymeric structures was able to prolong the residence time of organic carbon (Fig. 1) in 12-week incubation experiments.
![]() |
The evidence obtained by Martin and co-workers can be interpreted to indicate that labile carbon may become stabilised through abiotic or biotic syntheses into polymeric molecules that are necessarily more complex than the monomers from which they originate. The syntheses of polymeric molecules – assuming this to be a quantitatively significant process in soils and sediments – would necessarily take some time. This in turn would suggest that the longer an organic matter fragment persists in soil, the more likely it is to become resistant to decomposition as a consequence of being involved in some polymerisation reaction incorporating it into a more complex molecule. This idea was paraphrased by Stott and Martin[51] as the paradigm of ‘old humus is more stable than young humus’. Classical humification theories[67] can be seen as attempts to propose potential chemical pathways that would create such complex polymeric structures in soils.
The carbon quality-temperature (CQT) theory
Because complex geopolymers decompose at a slower rate than the monomers from which they originate, they can be perceived as having a lower quality as a substrate for microbial decomposition than the monomers. The term ‘quality’ is rather general and not easily parameterised, which is an obstacle to its incorporation into numerical models. To alleviate this disadvantage, Bosatta and Ågren[15] proposed to represent quality as ‘the number of enzymatic steps required to release as carbon dioxide a carbon from an organic compound. The larger the number of steps the lower is the quality of the carbon atom’. It can be shown that decomposing a complex molecule requires more enzymatic steps. Since each of these enzymatic steps has a characteristic, reaction-specific, temperature dependent activation energy (Ea), the total activation energy required to decompose a complex molecule is higher than that for one of its monomers. Thus the carbon-quality-temperature hypothesis assumes that the enzymatic reactions required to metabolise structurally complex, low-quality C substrates should have a higher net activation energy than reactions metabolising C substrates that are structurally simpler.[68] As the net activation energy increases, the temperature sensitivity as expressed by the Q10 value should also increase (Eqn 3).
There are three important corollaries of this approach: it (a) allows the general term ‘quality’ to be expressed in a numerical fashion by connecting it to the thermodynamics of an enzyme catalysed reaction, it (b) provides a mechanistic explanation for the observed decrease in decomposition rate (Fig. 1) with decreasing substrate quality, and most importantly, it (c) suggests that the decomposition rate of low quality substrates (= complex polymeric macromolecules) should have a stronger temperature dependence than that of high-quality substrates (= simple monomers) – always assuming quality to be a function of complexity, and complexity to result from the polymerisation of simple, labile compounds into more complex and more stable compounds.
According to the Arrhenius-theory,[69] the relation between the sensitivity of a reaction to a temperature increase (Q10) and the activation energy for this reaction (Ea) can be obtained by combining Eqns 1
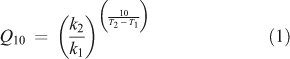
and 2

to define

with Q10 = a dimensionless number representing temperature sensitivity (= the change in decomposition rate k for a temperature difference of 10 K under otherwise constant conditions[70]) and k1 = reaction rate (often expressed as mol CO2 evolved per time unit) at temperature T1 (K), k2 = reaction rate at temperature T2 = T1 + 10 K; R = the gas constant (8.314 J mol–1 K–1) and Ea = activation energy.
Varying Ea in Eqn 3 illustrates that a compound with low Ea will necessarily exhibit a lesser response to a temperature increase than will a compound with a high Ea. With more heat in the atmosphere, more energy will be available to overcome activation energy barriers for decomposition reactions with high activation energies. Since the increase in reaction rate (= the Q10 value) is larger for reactions with high activation energies (that is, for reactions that require a large energy investment before they can proceed), materials requiring high Ea will show a more pronounced response to rising temperatures (= will decompose disproportionately faster) than materials requiring low Ea.[14]
Factors like substrate availability,[71] interactions of organic compounds with the abiotic matrix,[7] and freezing, drought, or flooding[14] have been shown to affect apparent temperature sensitivities of organic compounds. Yet it has become quite common to equate high Ea values of organic materials with ‘chemical recalcitrance’,[14,72,73] and to divide soil organic matter into labile and ‘more resistant’ fractions based on observed Q10 values.[23]
Challenging the concept of recalcitrance
Basic thermodynamics
The second law of thermodynamics commands that energy spontaneously flows only from being concentrated in one place to becoming diffused or spread out, not vice versa. Consequently, reviews[74] and textbook chapters[75] addressing the cycling of organic matter in the environment typically introduce natural organic matter as a thermodynamic anomaly dependent on life processes. Life is recognised as a situation where matter is in a state of particularly high order and thus, low entropy. The 2nd law prevents this state from becoming permanent; it forces organisms to continuously process energy to escape disintegration into disordered systems.[75] To counteract their inevitable energy losses, living organisms are forced to procure highly ordered molecules that have low entropy, high energy content and high free energy and to convert them into disordered molecules with high entropy, low energy content and low free energy.[76] Once life terminates, the 2nd law demands that energy stored in the reduced carbon constituents of dead organisms be dissipated throughout the system. Dead reduced carbon is forced to react with suitable electron acceptors (like O2, NO3–, Fe3+ and others) to form molecules with higher entropy and lower free energy. The 2nd law of thermodynamics thus creates a boundary condition for the assumption of inherent stability by determining that no carbon will stay in soil forever[25,50] – unless it is protected against decomposition. Such protection can be very powerful, as illustrated by reports of charcoal in Silurian sediments.[77]
Activation energy as a measure for recalcitrance
Todays atmosphere contains ∼21 wt% of oxygen, which is the second most powerful electron attractor in the periodic table. This fact brings up the question: why is all the electron-rich reduced carbon in the biosphere not spontaneously reacting with this abundance of oxygen to satisfy the 2nd law of thermodynamics and achieve greater entropy by converting few highly organised, energy rich, condensed organic biomolecules into many energy-poor, gaseous and thus more disordered CO2 molecules? The transformation of organic macromolecules into carbon dioxide requires that the chemical bonds of the former be broken first, a process that requires an initial input of activation energy (Ea). As long as this energy investment is not made, no reaction will take place and the compound will appear stable or ‘recalcitrant’. Activation energies are specific for given combinations of reactants and catalysts, but they can be modified by the response of the catalyst to environmental conditions such as substrate availability[71] and variations in pH. Experiments carried out on the trypsin-catalysed hydrolysis of amino acid esters illustrate this point. A histidine in the basic form is an essential part of the catalytic site of this enzyme. The activation energy at pH 6.0 is 76 kJ mol–1 and at pH 7.5 (when the histidine is predominantly in the basic form) it is 46 kJ mol–1.[69] Activation energy requirements for enzyme-catalysed decomposition reactions will therefore change as a function of the susceptibility of the catalyst towards variations in environmental conditions like pH.
Recalcitrance an inherent property?
If recalcitrance is defined as inherent biochemical stability,[54] that is if it is seen as a molecular property that operates independent from interactions with the abiotic environment,[19] then the ability of an organic compound to resist microbial decomposition is conceptualised as a characteristic that rests completely with the organic compound in question (= an ‘intrinsic’ or ‘material’ property). The implication is that the persistence of an intrinsically recalcitrant compound would not be affected by changes in environmental conditions, whereas a protected compound becomes a ready substrate as soon as the protective mechanism ceases to operate. By definition, an inherently stable compound must exhibit resistance to decomposition regardless of the environment in which it is placed. However, abundant observational data for the biodegradation of pesticides in soils have long shown that degradation rates of the same chemical can vary greatly depending on extrinsic factors like clay content, pH and microbial community composition that are not at all related to the molecular composition of the compound in question. Recently, Feng and Simpson[72] showed that activation energies and Q10 values of lignin Vanillyl, Syringyl and Cinnamyl units varied when they were incubated in two different grassland soils from the Canadian prairie. The same lignin unit qualified as relatively recalcitrant in one soil (Ea = 88.3 kJ mol–1 and corresponding temperature sensitivity of Q10 = 3.5 at 15°C) but showed a decidedly more labile character in another (Ea = 49.4 kJ mol–1 and Q10 = 2.0, also at 15°C). Significantly, the magnitude of change in activation energy was not constant for the three compounds, but amounted to a factor of 1.8, 2.3 and 1.4 for lignin Vanillyl, Syringyl and Cinnamyl units respectively. Clearly, the decomposition of these lignin fragments was, to a large extent, controlled by other factors than their ‘intrinsic molecular properties’.
Resistance to chemical degradation and decomposability
The issue of the decomposability of seemingly refractory materials was discussed in a review by Derenne and Largeau,[78] who defined the adjective ‘refractory’ to mean being ‘insoluble and nonhydrolysable in a laboratory procedure’. This approach assumes that an organic compound that can resist rather violent chemical treatment (involving, for example, boiling in 6 M HCl as in acid hydrolyses) should be expected to be able to resist the supposedly much less aggressive natural decomposition process as well. Derenne and Largeau[78] found that of the seven major classes of supposedly refractory biopolymers investigated (Table 2), only thermally altered carbon showed the presumed relationship between being refractory (= insoluble and nonhydrolysable) in the laboratory and being able to persist in a soil environment.
![]() |
Complexity and recalcitrance as the result of humification
Classical carbon turnover models assume that the component molecules of soil organic matter are produced from degradation products by secondary syntheses reactions or so-called humification processes.[79] This assumption has led to the Humic Polymer Model of soil organic matter,[80] in which the component molecules are depicted as large, covalently bonded (‘humic’) polymers with unique chemical structures that are different from those of the starting materials. Consequently, the Humic Polymer Model implies inherent resistance of so-called humic substances to decomposition.[81] This model forms the basis of a huge literature (see reviews by Haider et al.,[82] Stott and Martin,[51] Huang and Hardie[79]) on methods to synthesise model humic substances like the ones that Haider and Martin[65] used to demonstrate how carbon in polymers decomposes more slowly than carbon in monomers (Fig. 1). The fact that scientists managed to reproduce some of the characteristics that they observed in alkaline humic extracts in laboratory settings was taken as evidence that they had been successful in reproducing the mechanisms operating in nature. The competing Molecular Aggregate Model[83] assumes that organic materials in soils are formed mainly by enzymatic depolymerisation and oxidation of plant biopolymers. These reactions are thought to transform the originally nonpolar aromatic and lipid plant components into amphiphilic molecules. These amphiphiles form membrane-like aggregates on mineral surfaces and micelle-like aggregates in solution. Individual molecules within supramolecular aggregates are pushed in place by entropic interactions with the polar solvent water and are not covalently bonded. Since the Molecular Aggregate Model does not provide a structural reason for inherent stability against decomposition, the fundamental difference between the ‘Humic Polymer’ concept and the ‘Molecular Aggregate’ model is that the latter does not invoke the creation of refractory phases with extended turnover time.[81] The experimental evidence from the recent past fails to identify distinct humic molecules in soils[84] and in alkali extracted humic substances.[85] In addition, alkaline extracts recently have been found to decompose on an annual time scale.[86] In combination with the Molecular Aggregate Model, these indications challenge the scientific bases of the CQT theory, where the temperature sensitivity of ‘recalcitrant’ organic carbon is explained through an increase in molecular complexity with progressing decomposition state.[14,15]
Slow turnover without recalcitrance
Old organic matter may contain microbial polysaccharides and proteins,[87–89] indicating that chemically labile organic matter can persist for long times in soils.[26] This observation is often explained by matrix protection through sorptive interactions and reduced accessibility,[25] but recent investigations have revealed that significant quantities of organic material in soil may persist in spite of being chemically labile, unprotected, accessible and decomposable.[48] Using modified Michaelis–Menten kinetics, Schimel and Weintraub[90] showed that the reactivity constant of a substrate (= its decomposability) cannot by itself induce limitation of the decomposition rate as long as enzyme concentration is a term in the reaction rate equation. Above a given enzyme concentration, enzymatic products from substrate decomposition are insufficient to meet the cell energy demands associated with enzyme syntheses. This means that microbial substrate decomposition can be strongly limited even if substrate availability is unlimited. In such a case the decomposability of a substrate does not determine its fate: labile organic matter may well be left behind. This is only one of many mechanisms with the potential to preserve organic carbon without recalcitrance, more possibilities are discussed in mechanistic detail elsewhere.[47,91,92]
Thermally altered organic matter
Pyrogenic carbon differs from other natural organic matter in that it has gone through a thermal transformation process after its precursor materials were assembled by enzymatic processes. In uncontrolled natural fires, the outcome is a stochastic mix of products with properties that are novel to the environment. Thus the decompository challenge associated with charcoal originates from the fact that it does not represent a well defined chemical compound or structure; rather, it represents a dynamic molecular property space within which molecular structure and crystallinity vary with the conditions under which the char was created. Depending on charring intensity, charcoal may contain individual polycondensate aromatic elements of increasing size, which eventually grow into graphene sheets that are then arranged to form increasingly complex turbostratic crystallites (Fig. 2).
Since natural fires differ in intensity, duration, precursor materials and many other factors,[93–96] the resulting palette of often highly alkaline chars and ashes will typically be rather diverse as well.[97] These considerations suggest that the event-specific combination of charring products is likely to be somewhat distant from the mainstream of catabolic pathways for common microorganisms even in fire-prone ecosystems. In other words, the likelihood that the decomposer community has the full suite of required enzymes available to decompose the multitude of thermally altered phases produced by any fire event is rather low, a fact that has been experimentally verified by demonstrating decreasing decomposition rates with increasing charring temperatures.[98] On the other hand, there can be no doubt that charcoal has been around long enough in earth history[77] to give the decomposer community ample chance to develop enzymatic pathways that can decompose even highly altered pyrogenic carbon. Observational evidence for the decomposability of charcoal has accumulated over the last years[99–104] and found its most recent confirmation in a study from Scandinavia showing a much lesser mean age of charcoal in forest soils than would have been expected based on fire frequency since the onset of the Holocene.[105]
How molecular characteristics affect decomposition of organic compounds
Several molecular features have been proposed as having the ability to render natural organic carbon compounds refractory, including molecular size,[18] aqueous solubility or polarity,[26] aromaticity,[106] aliphaticity expressed as alkyl : O-alkyl ratio,[107] molecular complexity,[15] certain N-containing substituents and functional groups[26] and many others. Fierer et al.[68] published the results of an incubation experiment that allows testing the effect of variations in some of these features (Table 3). To do so, respiration rate constants (B-values) from Fierer et al.[68] were plotted as a function of aqueous solubility (Fig. 3a), molecular mass (Fig. 3b), carbon oxidation state (Fig. 3c) and atomic oxygen:carbon ratio (Fig. 3d).
![]() |
Aqueous solubility and molecular mass did not explain any variability of decomposition rate-constants. In addition, they varied independent of each other: in the case of tannic acid, solubility was high (this should, in theory, facilitate decomposition) when molecular size was large (this should, in theory, make a molecule more recalcitrant). Thus neither parameter appeared to be suitable as a predictor for the decomposability of the organic compounds in this experimental setup. The molecular complexity of the compounds, approximated as the number of different bonds within the molecule (Table 3), varies within the limited range of 3 and 5, with the compound that had the highest Q10/Ea (catechol) being the least complex in terms of diversity of bonds. Catechol also has the lowest molecular mass of the seven compounds. Thus, an examination of what can be considered as classic molecular-level characteristics for recalcitrance reveals that they are not predictors for the decomposability of the substances examined here.
The picture changes when parameters are considered that link the chemical composition of the organic compounds to an outside resource: oxygen. The oxidation state of organic carbon (Cox) can vary between –4 (dominance of energy rich C–H bonds as in CH4) and +4 (dominance of low energy C=O bonds as in CO2). The range for oxidation states in different soil environments has been estimated between –0.45 and 0.3, with a mean of –0.26.[108,109] The ability to undergo further oxidation will depend on the availability of oxygen as a resource, and in this sense the parameter Cox integrates a molecular potential with a logistical constraint, that is, oxygen availability. Fig. 3c shows a clear trend of increasing decomposition rate with decreasing electron richness of the substrate. If universally applicable, such a trend should be reflected in the relative proportions of oxygen that is already incorporated in organic matter, because energy-poor bonds are made when organic matter is oxidised, and oxidation involves the introduction of oxygen-containing functional groups. Fig. 3d shows that B-values are lower (and activation energies higher, since B, Q10 and Ea are mathematically linked with each other, Eqn 1–3) for energy-rich compounds that contain less oxygen than do compounds of comparable size, similar solubility and similar molecular complexity. It can be deduced that even mild oxygen deprivation should have greater consequences for the decomposition of energy-rich substrates with low oxygen content and high activation energies than for substrates that already are partially oxidised. This inference is supported by observations of increasing relative proportions of aliphatic compounds with increasing soil depth and thus increasing distance from the atmospheric oxygen source.[110,111] Oxygen depleted microenvironments at the centre of aggregates were reported to persist for extended times after soils have been allowed to drain,[112] indicating that limitations in O2 supply may be common to many soil environments[113] and may explain long turnover times that were previously thought to be the result of ‘recalcitrance’. The length of time that organic carbon is exposed to molecular oxygen (oxygen exposure time, OET) at the location of deposition has long been identified as an important control on organic carbon preservation in continental margin sediments,[114–116] further corroborating the importance of oxygen limitation for organic matter preservation.
But do these findings not suggest that an energy-rich lipid with low molecular oxygen content and high activation energies should be viewed as being able to resist decomposition, and classified as ‘recalcitrant’?[32] I advise the reader to resist the temptation to do so. Lipids turned over on decadal time scales in well aerated topsoils[33] and selective preservation of any kind of organic material, including lipids, was not found to occur in a topsoil studied by Bol et al.[34] Thus a classification of a lipid as ‘recalcitrant’ would only be valid for a very closely constrained, oxygen depleted subsoil microenvironment. But it may not apply to the overlying topsoil or even the outside of an aggregate, creating the paradoxon that the same organic compound could be labile and recalcitrant at the same time and in the same soil, simply as a function of oxygen availability within the given microenvironment. Dependence of recalcitrance on environmental conditions, however, is logically incompatible with a definition of recalcitrance as ‘intrinsic molecular property’.
Conclusions
Classifications determine our attitudes and behaviour towards the object or phenomenon classified. For example, ‘when lightning was classified as “evidence of divine wrath”, no courses of action other than prayer were suggested to prevent one’s being struck by lightning. As soon, however, as it was classified as “electricity”, Benjamin Franklin achieved a measure of control over it by his invention of the lightning rod’.[117] One can make a similar point for the widely used classification of soil organic matter as either labile or recalcitrant: research will have to explore new avenues if the category of intrinsic recalcitrance is abandoned.
The evidence suggests that the persistence of reduced organic carbon in the environment is co-determined by the interaction between substrates, microbial actors and abiotic conditions. Organic matter turnover should thus be seen as dependent on microbial ecology and the logistic state of a specific environment, not on the ill-defined ‘recalcitrance’ of an organic compound. Varying degrees of structural organisation, microbial readiness and resource limitation within a given environment (soil aggregate, soil horizon) render it likely that identical organic compounds may turn over with different velocities as a result of variations in driving variables. Much confusion may be due to the fact that ‘recalcitrant carbon’ is often used as a synonym for ‘old’ carbon, neglecting that old age can be achieved through many other mechanisms than ‘inherent chemical recalcitrance’.
What is recalcitrant soil organic matter? In the opinion of this author, ‘recalcitrance’ is a category of semantic convenience and not a useful classification of material properties. Conceptual progress appears to be certain if soil organic matter is reclassified as a reservoir of reduced carbon in different states of protection against decomposition. If such a conceptual step is taken, the enigma behind the temperature sensitivity of organic matter decomposition may be a step closer to a solution, and robust strategies of soil carbon sequestration can be based on mechanisms of protection.
Acknowledgements
I am indebted to Noah Fierer for sharing the data for Fig. 3 and to Joan Sandeno for language edits to the final version of the manuscript. Marco Keiluweit, Peter S. Nico and Dave D. Myrold provided helpful comments on earlier versions of the manuscript. Three anonymous reviewers are acknowledged for their constructive suggestions to improve the manuscript.
[1]
E. G. Jobbágy ,
R. B. Jackson ,
The vertical distribution of soil organic carbon and its relation to climate and vegetation.
Ecol. Appl. 2000
, 10, 423.
| Crossref | GoogleScholarGoogle Scholar |
[2]
C. Tarnocai ,
J. G. Canadell ,
E. A. G. Schuur ,
P. Kuhry ,
G. Mazhitova ,
S. Zimov ,
Soil organic carbon pools in the northern circumpolar permafrost region.
Global Biogeochem. Cy. 2009
, 23, GB2023.
| Crossref | GoogleScholarGoogle Scholar |
[3]
M. Heimann ,
M. Reichstein ,
Terrestrial ecosystem carbon dynamics and climate feedbacks.
Nature 2008
, 451, 289.
| Crossref | GoogleScholarGoogle Scholar | PubMed |
[4]
M. S. Torn ,
J. Harte ,
Missing feedbacks, asymmetric uncertainties, and the underestimation of future warming.
Geophys. Res. Lett. 2006
, 33, L10703.
| Crossref | GoogleScholarGoogle Scholar |
[5]
M. Scheffer ,
V. Brovkin ,
P. M. Cox ,
Positive feedback between global warming and atmospheric CO2 concentration inferred from past climate change.
Geophys. Res. Lett. 2006
, 33, L10702.
| Crossref | GoogleScholarGoogle Scholar |
[6]
E. Dorrepaal ,
S. Toet ,
R. S. P. van Logtestijn ,
E. Swart ,
M. J. van de Weg ,
T. V. Callaghan ,
R. Aerts ,
Carbon respiration from subsurface peat accelerated by climate warming in the subarctic.
Nature 2009
, 460, 616.
| Crossref | GoogleScholarGoogle Scholar |
[7]
M. von Lützow ,
I. Kögel-Knabner ,
Temperature sensitivity of soil organic matter decomposition-what do we know?
Biol. Fertil. Soils 2009
, 46, 1.
| Crossref | GoogleScholarGoogle Scholar |
[8]
C. M. Fang ,
P. Smith ,
J. B. Moncrieff ,
J. U. Smith ,
Similar response of labile and resistant soil organic matter pools to changes in temperature.
Nature 2005
, 433, 57.
| Crossref | GoogleScholarGoogle Scholar | PubMed |
[9]
C. Fang ,
P. Smith ,
J. U. Smith ,
Is resistant soil organic matter more sensitive to temperature than the labile organic matter?
Biogeosciences 2006
, 3, 65.
| Crossref | GoogleScholarGoogle Scholar |
[10]
M. Reichstein ,
T. Katterer ,
O. Andren ,
P. Ciais ,
E. D. Schulze ,
W. Cramer ,
D. Papale ,
R. Valentini ,
Temperature sensitivity of decomposition in relation to soil organic matter pools: critique and outlook.
Biogeosciences 2005
, 2, 317.
| Crossref | GoogleScholarGoogle Scholar |
[11]
M. Reichstein ,
J. A. Subke ,
A. C. Angeli ,
J. D. Tenhunen ,
Does the temperature sensitivity of decomposition of soil organic matter depend upon water content, soil horizon, or incubation time?
Glob. Change Biol. 2005
, 11, 1754.
| Crossref | GoogleScholarGoogle Scholar |
[12]
F. Conen ,
J. Leifeld ,
B. Seth ,
C. Alewell ,
Warming mineralises young and old soil carbon equally.
Biogeosciences 2006
, 3, 515.
| Crossref | GoogleScholarGoogle Scholar |
[13]
[14]
E. A. Davidson ,
I. A. Janssens ,
Temperature sensitivity of soil carbon decomposition and feedbacks to climate change.
Nature 2006
, 440, 165.
| Crossref | GoogleScholarGoogle Scholar | PubMed |
[15]
E. Bosatta ,
G. I. Agren ,
Soil organic matter quality interpreted thermodynamically.
Soil Biol. Biochem. 1999
, 31, 1889.
| Crossref | GoogleScholarGoogle Scholar |
[16]
B. Marschner ,
S. Brodowski ,
A. Dreves ,
G. Gleixner ,
A. Gude ,
P. M. Grootes ,
U. Hamer ,
A. Heim ,
et al. How relevant is recalcitrance for the stabilization of organic matter in soils?
J. Plant Nutr. Soil Sc. 2008
, 171, 91.
| Crossref | GoogleScholarGoogle Scholar |
[17]
D. L. Wixon ,
T. C. Balser ,
Complexity, climate change and soil carbon: a systems approach to microbial temperature response.
Syst. Res. Behav. Sci. 2009
, 26, 601.
| Crossref | GoogleScholarGoogle Scholar |
[18]
M. Alexander ,
Biodegradation of chemicals of environmental concern.
Science 1981
, 211, 132.
| Crossref | GoogleScholarGoogle Scholar | PubMed |
[19]
P. Sollins ,
P. Homann ,
B. A. Caldwell ,
Stabilization and destabilization of soil organic matter: Mechanisms and controls.
Geoderma 1996
, 74, 65.
| Crossref | GoogleScholarGoogle Scholar |
[20]
J. A. Baldock ,
J. O. Skjemstad ,
Role of the soil matrix and minerals in protecting natural organic materials against biological attack.
Org. Geochem. 2000
, 31, 697.
| Crossref | GoogleScholarGoogle Scholar |
[21]
[22]
J. Balesdent ,
S. Recous ,
The residence times of C, and the potential for C storage in some French cultivated soils.
Can. J. Soil Sci. 1997
, 77, 187.
[23]
R. T. Conant ,
R. A. Drijber ,
M. L. Haddix ,
W. J. Parton ,
E. A. Paul ,
A. F. Plante ,
J. Six ,
J. M. Steinweg ,
Sensitivity of organic matter decomposition to warming varies with its quality.
Glob. Change Biol. 2008
, 14, 868.
| Crossref | GoogleScholarGoogle Scholar |
[24]
R. T. Conant ,
J. M. Steinweg ,
M. L. Haddix ,
E. A. Paul ,
A. F. Plante ,
J. Six ,
Experimental warming shows that decomposition temperature sensitivity increases with soil organic matter recalcitrance.
Ecology 2008
, 89, 2384.
| Crossref | GoogleScholarGoogle Scholar | PubMed |
[25]
S. Trumbore ,
Radiocarbon and soil carbon dynamics.
Annu. Rev. Earth Planet. Sci. 2009
, 37, 47.
| Crossref | GoogleScholarGoogle Scholar |
[26]
M. von Lützow ,
I. Kögel-Knabner ,
K. Ekschmitt ,
E. Matzner ,
G. Guggenberger ,
B. Marschner ,
H. Flessa ,
Stabilization of organic matter in temperate soils: mechanisms and their relevance under different soil conditions – a review.
Eur. J. Soil Sci. 2006
, 57, 426.
| Crossref | GoogleScholarGoogle Scholar |
[27]
[28]
H.-R. Schulten ,
M. Schnitzer ,
Chemical model structures for soil organic matter and soils.
Soil Sci. 1997
, 162, 115.
| Crossref | GoogleScholarGoogle Scholar |
[29]
R. Swift ,
Macromolecular properties of soil humic substances: fact, fiction and opinion.
Soil Sci. 1999
, 164, 790.
| Crossref | GoogleScholarGoogle Scholar |
[30]
A. Piccolo ,
The supramolecular structure of humic substances.
Soil Sci. 2001
, 166, 810.
| Crossref | GoogleScholarGoogle Scholar |
[31]
R. Sutton ,
G. Sposito ,
Molecular structure in soil humic substances: the new view.
Environ. Sci. Technol. 2005
, 39, 9009.
| Crossref | GoogleScholarGoogle Scholar | PubMed |
[32]
K. Lorenz ,
R. Lal ,
C. M. Preston ,
K. G. J. Nierop ,
Strengthening the soil organic carbon pool by increasing contributions from recalcitrant aliphatic bio(macro)molecules.
Geoderma 2007
, 142, 1.
| Crossref | GoogleScholarGoogle Scholar |
[33]
G. L. B. Wiesenberg ,
J. Schwarzbauer ,
M. W. I. Schmidt ,
L. Schwark ,
Source and turnover of organic matter in agricultural soils derived from n-alkane/n-carboxylic acid compositions and C-isotope signatures.
Org. Geochem. 2004
, 35, 1371.
[34]
R. Bol ,
N. Poirier ,
J. Balesdent ,
G. Gleixner ,
Molecular turnover time of soil organic matter in particle-size fractions of an arable soil.
Rapid Commun. Mass Spectrom. 2009
, 23, 2551.
| Crossref | GoogleScholarGoogle Scholar | PubMed |
[35]
M. Alexander ,
Biodegradation: problems of molecular recalcitrance and microbial fallibility.
Adv. Appl. Microbiol. 1965
, 7, 35.
| Crossref | GoogleScholarGoogle Scholar | PubMed |
[36]
E. S. Krull ,
J. A. Baldock ,
J. O. Skjemstad ,
Importance of mechanisms and processes of the stabilization of soil organic matter for modelling carbon turnover.
Funct. Plant Biol. 2003
, 30, 207.
| Crossref | GoogleScholarGoogle Scholar |
[37]
[38]
D. S. Jenkinson ,
The turnover of organic carbon and nitrogen in soil.
Philos. Trans. R. Soc. Lond. B Biol. Sci. 1990
, 329, 361.
| Crossref | GoogleScholarGoogle Scholar |
[39]
T. Dittmar ,
G. Kattner ,
Recalcitrant dissolved organic matter in the ocean: major contribution of small amphiphilics.
Mar. Chem. 2003
, 82, 115.
| Crossref | GoogleScholarGoogle Scholar |
[40]
T. Dittmar ,
J. Paeng ,
A heat-induced molecular signature in marine dissolved organic matter.
Nature Geosci. 2009
, 2, 175.
| Crossref | GoogleScholarGoogle Scholar |
[41]
[42]
[43]
A. Tietema ,
D. VanDam ,
Calculating microbial carbon and nitrogen transformations in acid forest litter with 15N enrichment and dynamic simulation modelling.
Soil Biol. Biochem. 1996
, 28, 953.
| Crossref | GoogleScholarGoogle Scholar |
[44]
[45]
M. Alexander ,
Biochemical ecology of soil microorganisms.
Annu. Rev. Microbiol. 1964
, 18, 217.
| Crossref | GoogleScholarGoogle Scholar | PubMed |
[46]
L. M. Mayer ,
The inertness of being organic.
Mar. Chem. 2004
, 92, 135.
| Crossref | GoogleScholarGoogle Scholar |
[47]
K. Ekschmitt ,
E. Kandeler ,
C. Poll ,
A. Brune ,
F. Buscot ,
M. Friedrich ,
G. Gleixner ,
A. Hartmann ,
et al. Soil-carbon preservation through habitat constraints and biological limitations on decomposer activity.
J. Plant Nutr. Soil Sc. 2008
, 171, 27.
| Crossref | GoogleScholarGoogle Scholar |
[48]
K. Ekschmitt ,
M. Liu ,
S. Vetter ,
O. Fox ,
V. Wolters ,
Strategies used by soil biota to overcome soil organic matter stability – why is dead organic matter left over in the soil?
Geoderma 2005
, 128, 167.
| Crossref | GoogleScholarGoogle Scholar |
[49]
W. J. Parton ,
D. S. Schimel ,
C. V. Cole ,
D. S. Ojima ,
Analysis of factors controlling soil organic matter levels in Great Plains grasslands.
Soil Sci. Soc. Am. J. 1987
, 51, 1173.
| Crossref |
[50]
Y. S. Feng ,
Fundamental considerations of soil organic carbon dynamics: a new theoretical framework.
Soil Sci. 2009
, 174, 467.
| Crossref | GoogleScholarGoogle Scholar |
[51]
[52]
G. I. Ågren ,
E. Bosatta ,
Quality: A bridge between theory and experiment in soil organic matter studies.
Oikos 1996
, 76, 522.
| Crossref | GoogleScholarGoogle Scholar |
[53]
C. Fang ,
P. Smith ,
J. U. Smith ,
A simple equation for simulating C decomposition in a multi-component pool of soil organic matter.
Eur. J. Soil Sci. 2005
, 56, 815.
[54]
J. Six ,
R. T. Conant ,
E. A. Paul ,
K. Paustian ,
Stabilization mechanisms of soil organic matter: Implications for C-saturation of soils.
Plant Soil 2002
, 241, 155.
| Crossref | GoogleScholarGoogle Scholar |
[55]
[56]
I. Kögel-Knabner ,
The macromolecular organic composition of plant and microbial residues as inputs to soil organic matter.
Soil Biol. Biochem. 2002
, 34, 139.
| Crossref | GoogleScholarGoogle Scholar |
[57]
[58]
J. D. Aber ,
J. M. Melillo ,
C. A. McClaugherty ,
Predicting long-term patterns of mass-loss, nitrogen dynamics, and soil organic matter formation from initial litter chemistry in temperate forest ecosystems.
Can. J. Bot. 1990
, 68, 2201.
| Crossref | GoogleScholarGoogle Scholar |
[59]
B. R. Taylor ,
C. E. Prescott ,
W. J. F. Parsons ,
D. Parkinson ,
Substrate control of litter decomposition in four Rocky Mountain coniferous forests.
Can. J. Bot. 1991
, 69, 2242.
| Crossref | GoogleScholarGoogle Scholar |
[60]
W. L. Silver ,
R. K. Miya ,
Global patterns in root decomposition: comparisons of climate and litter quality effects.
Oecologia 2001
, 129, 407.
[61]
R. Hatfield ,
R. S. Fukushima ,
Can lignin be accurately measured?
Crop Sci. 2005
, 45, 832.
| Crossref | GoogleScholarGoogle Scholar |
[62]
[63]
J. P. Martin ,
K. Haider ,
Microbial activity in relation to soil humus formation.
Soil Sci. 1971
, 111, 54.
| Crossref | GoogleScholarGoogle Scholar |
[64]
J. P. Martin ,
H. Zunino ,
P. Peirano ,
M. Caiozzi ,
K. Haider ,
Decomposition of 14C-labeled lignins, model humic acid polymers, and funal melanins in allophanic soils.
Soil Biol. Biochem. 1982
, 14, 289.
| Crossref | GoogleScholarGoogle Scholar |
[65]
K. Haider ,
J. P. Martin ,
Decomposition of specifically C14 labeled benzoic and cinnamic acid derivatives in soil.
Soil Sci. Soc. Am. J. 1975
, 39, 657.
| Crossref |
[66]
K. Haider ,
J. Trojanowski ,
Decomposition of specifically C-14-labeled phenols and dehydropolymers of coniferyl alcohol as models for lignin degradation by soft and white rot fungi.
Arch. Microbiol. 1975
, 105, 33.
| Crossref | GoogleScholarGoogle Scholar |
[67]
[68]
N. Fierer ,
J. M. Craine ,
K. McLauchlan ,
J. P. Schimel ,
Litter quality and the temperature sensitivity of decomposition.
Ecology 2005
, 86, 320.
| Crossref | GoogleScholarGoogle Scholar |
[69]
[70]
C. M. Fang ,
P. Smith ,
J. B. Moncrieff ,
J. U. Smith ,
Corrigendum: Similar response of labile and resistant soil organic matter pools to changes in temperature.
Nature 2005
, 436, 881.
| Crossref | GoogleScholarGoogle Scholar |
[71]
E. A. Davidson ,
I. A. Janssens ,
Y. Q. Luo ,
On the variability of respiration in terrestrial ecosystems: moving beyond Q10.
Glob. Change Biol. 2006
, 12, 154.
| Crossref | GoogleScholarGoogle Scholar |
[72]
X. J. Feng ,
M. J. Simpson ,
Temperature responses of individual soil organic matter components.
J. Geophys. Res. 2008
, 113, G03036.
| Crossref | GoogleScholarGoogle Scholar |
[73]
N. Fierer ,
B. P. Colman ,
J. P. Schimel ,
R. B. Jackson ,
Predicting the temperature dependence of microbial respiration in soil: a continental-scale analysis.
Global Biogeochem. Cy. 2006
, 20, GB3026.
| Crossref | GoogleScholarGoogle Scholar |
[74]
J. I. Hedges ,
G. Eglinton ,
P. G. Hatcher ,
D. L. Kirchman ,
C. Arnosti ,
S. Derenne ,
R. P. Evershed ,
I. Kögel-Knabner ,
et al. The molecularly-uncharacterized component of nonliving organic matter in natural environments.
Org. Geochem. 2000
, 31, 945.
| Crossref | GoogleScholarGoogle Scholar |
[75]
[76]
[77]
I. J. Glasspool ,
D. Edwards ,
L. Axe ,
Charcoal in the Silurian as evidence for the earliest wildfire.
Geology 2004
, 32, 381.
| Crossref | GoogleScholarGoogle Scholar |
[78]
S. Derenne ,
C. Largeau ,
A review of some important families of refractory macromolecules: composition, origin and fate in soils and sediments.
Soil Sci. 2001
, 166, 833.
| Crossref | GoogleScholarGoogle Scholar |
[79]
[80]
[81]
M. Kleber ,
M. G. Johnson ,
Advances in understanding the molecular structure of soil organic matter: implications for interactions in the environment.
Adv. Agron. 2010
, 106, 77.
| Crossref | GoogleScholarGoogle Scholar |
[82]
[83]
[84]
J. Lehmann ,
D. Solomon ,
J. Kinyangi ,
L. Dathe ,
S. Wirick ,
C. Jacobsen ,
Spatial complexity of soil organic matter forms at nanometre scales.
Nature Geosci. 2008
, 1, 238.
| Crossref | GoogleScholarGoogle Scholar |
[85]
B. P. Kelleher ,
A. J. Simpson ,
Humic substances in soils: are they really chemically distinct?
Environ. Sci. Technol. 2006
, 40, 4605.
| Crossref | GoogleScholarGoogle Scholar | PubMed |
[86]
M. Tatzber ,
M. Stemmer ,
H. Spiegel ,
C. Katzlberger ,
F. Zehetner ,
G. Haberhauer ,
K. Roth ,
E. Garcia-Garcia ,
M. H. Gerzabek ,
Decomposition of carbon-14-labeled organic amendments and humic acids in a long-term field experiment.
Soil Sci. Soc. Am. J. 2009
, 73, 744.
| Crossref | GoogleScholarGoogle Scholar |
[87]
W. Amelung ,
I. Lobe ,
C. C. Du Preez ,
Fate of microbial residues in sandy soils of the South African Highveld as influenced by prolonged arable cropping.
Eur. J. Soil Sci. 2002
, 53, 29.
| Crossref | GoogleScholarGoogle Scholar |
[88]
R. Kiem ,
I. Koegel-Knabner ,
Contribution of lignin and polysaccharides to the refractory carbon pool in C-depleted arable soils.
Soil Biol. Biochem. 2003
, 35, 101.
| Crossref | GoogleScholarGoogle Scholar |
[89]
H. Knicker ,
Stabilization of N-compounds in soil and organic-matter-rich sediments – what is the difference?
Mar. Chem. 2004
, 92, 167.
| Crossref | GoogleScholarGoogle Scholar |
[90]
J. P. Schimel ,
M. N. Weintraub ,
The implications of exoenzyme activity on microbial carbon and nitrogen limitation in soil: a theoretical model.
Soil Biol. Biochem. 2003
, 35, 549.
| Crossref | GoogleScholarGoogle Scholar |
[91]
T. Wutzler ,
M. Reichstein ,
Colimitation of decomposition by substrate and decomposers – a comparison of model formulations.
Biogeosciences 2008
, 5, 749.
| Crossref | GoogleScholarGoogle Scholar |
[92]
S. Manzoni ,
A. Porporato ,
Soil carbon and nitrogen mineralization: theory and models across scales.
Soil Biol. Biochem. 2009
, 41, 1355.
| Crossref | GoogleScholarGoogle Scholar |
[93]
D. G. Neary ,
C. C. Klopatek ,
L. F. DeBano ,
P. F. Ffolliott ,
Fire effects on belowground sustainability: a review and synthesis.
For. Ecol. Manage. 1999
, 122, 51.
| Crossref | GoogleScholarGoogle Scholar |
[94]
M. I. Bird ,
Fire in the earth sciences.
Episodes 1997
, 20, 223.
[95]
H. Knicker ,
How does fire affect the nature and stability of soil organic nitrogen and carbon? A review.
Biogeochemistry 2007
, 85, 91.
| Crossref | GoogleScholarGoogle Scholar |
[96]
A. Miltner ,
W. Zech ,
Effects of minerals on the transformation of organic matter during simulated fire-induced pyrolysis.
Org. Geochem. 1997
, 26, 175.
| Crossref | GoogleScholarGoogle Scholar |
[97]
C. I. Czimczik ,
C. M. Preston ,
M. W. I. Schmidt ,
E. D. Schulze ,
How surface fire in Siberian Scots pine forests affects soil organic carbon in the forest floor: stocks, molecular structure, and conversion to black carbon (charcoal).
Global Biogeochem. Cy. 2003
, 17, 1020.
| Crossref | GoogleScholarGoogle Scholar |
[98]
J. A. Baldock ,
R. J. Smernik ,
Chemical composition and bioavailability of thermally altered Pinus resinosa (Red pine) wood.
Org. Geochem. 2002
, 33, 1093.
| Crossref | GoogleScholarGoogle Scholar |
[99]
U. Hamer ,
B. Marschner ,
S. Brodowski ,
W. Amelung ,
Interactive priming of black carbon and glucose mineralisation.
Org. Geochem. 2004
, 35, 823.
| Crossref | GoogleScholarGoogle Scholar |
[100]
K. Hammes ,
M. S. Torn ,
A. G. Lapenas ,
M. W. I. Schmidt ,
Centennial black carbon turnover observed in a Russian steppe soil.
Biogeosciences 2008
, 5, 1339.
| Crossref | GoogleScholarGoogle Scholar |
[101]
C. H. Cheng ,
J. Lehmann ,
M. H. Engelhard ,
Natural oxidation of black carbon in soils: changes in molecular form and surface charge along a climosequence.
Geochim. Cosmochim. Acta 2008
, 72, 1598.
| Crossref | GoogleScholarGoogle Scholar |
[102]
C.-H. Cheng ,
J. Lehmann ,
J. E. Thies ,
S. D. Burton ,
Stability of black carbon in soils across a climatic gradient.
J. Geophys. Res. 2008
, 113, G02027.
| Crossref | GoogleScholarGoogle Scholar |
[103]
C.-H. Cheng ,
J. Lehmann ,
J. E. Thies ,
S. D. Burton ,
M. H. Engelhard ,
Oxidation of black carbon by biotic and abiotic processes.
Org. Geochem. 2006
, 37, 1477.
| Crossref | GoogleScholarGoogle Scholar |
[104]
M. I. Bird ,
C. Moyo ,
E. M. Veenendaal ,
J. Lloyd ,
P. Frost ,
Stability of elemental carbon in a savanna soil.
Global Biogeochem. Cy. 1999
, 13, 923.
| Crossref | GoogleScholarGoogle Scholar |
[105]
M. Ohlson ,
B. Dahlberg ,
T. Okland ,
K. J. Brown ,
R. Halvorsen ,
The charcoal carbon pool in boreal forest soils.
Nature Geosci. 2009
, 2, 692.
| Crossref | GoogleScholarGoogle Scholar |
[106]
C. A. Campbell ,
E. A. Paul ,
D. A. Rennie ,
K. J. McCallum ,
Applicability of the carbon-dating method of analysis to soil humus studies.
Soil Sci. 1967
, 104, 217.
| Crossref | GoogleScholarGoogle Scholar |
[107]
J. A. Baldock ,
J. M. Oades ,
P. N. Nelson ,
T. M. Skene ,
A. Golchin ,
P. Clarke ,
Assessing the extent of decomposition of natural organic materials using solid-state 13C NMR spectroscopy.
Aust. J. Soil Res. 1997
, 35, 1061.
| Crossref | GoogleScholarGoogle Scholar |
[108]
C. A. Masiello ,
W. C. Hockaday ,
M. E. Gallagher ,
Organic carbon oxidation state (C-ox): a new proxy for the Earth’s C and O cycles.
Geochimica Et Cosmochimica Acta 2007
, 71, A633.
[109]
W. C. Hockaday ,
C. A. Masiello ,
J. T. Randerson ,
R. J. Smernik ,
J. A. Baldock ,
O. A. Chadwick ,
J. W. Harden ,
Measurement of soil carbon oxidation state and oxidative ratio by 13C nuclear magnetic resonance.
J. Geophys. Res. 2009
, 114, G02014.
| Crossref | GoogleScholarGoogle Scholar |
[110]
C. Rumpel ,
K. Eusterhues ,
I. Kogel-Knabner ,
Location and chemical composition of stabilized organic carbon in topsoil and subsoil horizons of two acid forest soils.
Soil Biol. Biochem. 2004
, 36, 177.
| Crossref | GoogleScholarGoogle Scholar |
[111]
C. Rumpel ,
A. Seraphin ,
M. O. Goebel ,
G. Wiesenberg ,
F. Gonzales-Vila ,
J. Bachmann ,
L. Schwark ,
W. Michaelis ,
A. Mariotti ,
I. Kögel-Knabner ,
Alkyl C and hydrophobicity in B and C horizons of an acid forest soil.
J. Plant Nutr. Soil Sc. 2004
, 167, 685.
| Crossref | GoogleScholarGoogle Scholar |
[112]
A. J. Sexstone ,
N. P. Revsbech ,
T. B. Parkin ,
J. M. Tiedje ,
Direct measurement of oxygen profiles and denitrification rates in soil aggregates.
Soil Sci. Soc. Am. J. 1985
, 49, 645.
| Crossref |
[113]
G. E. M. van der Lee ,
B. de Winder ,
W. Bouten ,
A. Tietema ,
Anoxic microsites in Douglas fir litter.
Soil Biol. Biochem. 1999
, 31, 1295.
| Crossref | GoogleScholarGoogle Scholar |
[114]
H. E. Hartnett ,
R. G. Keil ,
J. I. Hedges ,
A. H. Devol ,
Influence of oxygen exposure time on organic carbon preservation in continental margin sediments.
Nature 1998
, 391, 572.
| Crossref | GoogleScholarGoogle Scholar |
[115]
R. G. Keil ,
A. F. Dickens ,
T. Arnarson ,
B. L. Nunn ,
A. H. Devol ,
What is the oxygen exposure time of laterally transported organic matter along the Washington margin?
Mar. Chem. 2004
, 92, 157.
| Crossref | GoogleScholarGoogle Scholar |
[116]
J. I. Hedges ,
R. G. Keil ,
Sedimentary organic matter preservation: an assessment and speculative synthesis.
Mar. Chem. 1995
, 49, 81.
| Crossref | GoogleScholarGoogle Scholar |
[117]
[118]
[119]
P. D. Falloon ,
P. Smith ,
Modelling refractory soil organic matter.
Biol. Fertil. Soils 2000
, 30, 388.
| Crossref | GoogleScholarGoogle Scholar |
[120]
J. M. Talbot ,
S. D. Allison ,
K. K. Treseder ,
Decomposers in disguise: mycorrhizal fungi as regulators of soil C dynamics in ecosystems under global change.
Funct. Ecol. 2008
, 22, 955.
| Crossref | GoogleScholarGoogle Scholar |
[121]
C. A. Masiello ,
M. E. Gallagher ,
J. T. Randerson ,
R. M. Deco ,
O. A. Chadwick ,
Evaluating two experimental approaches for measuring ecosystem carbon oxidation state and oxidative ratio.
J. Geophys. Res. – Biogeosciences 2008
, 113, G03010.
| Crossref | GoogleScholarGoogle Scholar |
[122]
S. E. Trumbore ,
S. H. Zheng ,
Comparison of fractionation methods for soil organic matter C-14 analysis.
Radiocarbon 1996
, 38, 219.
[123]
H. Knicker ,
P. G. Hatcher ,
Sequestration of organic nitrogen in the sapropel from Mangrove Lake, Bermuda.
Org. Geochem. 2001
, 32, 733.
| Crossref | GoogleScholarGoogle Scholar |
[124]
K. Eusterhues ,
C. Rumpel ,
M. Kleber ,
I. Kogel-Knabner ,
Stabilisation of soil organic matter by interactions with minerals as revealed by mineral dissolution and oxidative degradation.
Org. Geochem. 2003
, 34, 1591.
| Crossref | GoogleScholarGoogle Scholar |
[125]
K. Haase ,
K. Wantzen ,
Analysis and decomposition of condensed tannins in tree leaves.
Environ. Chem. Lett. 2008
, 6, 71.
| Crossref | GoogleScholarGoogle Scholar |
[126]
N. Poirier ,
S. Derenne ,
J. N. Rouzaud ,
C. Largeau ,
A. Mariotti ,
J. Balesdent ,
J. Maquet ,
Chemical structure and sources of the macromolecular, resistant, organic fraction isolated from a forest soil (Lacadee, south-west France).
Org. Geochem. 2000
, 31, 813.
| Crossref | GoogleScholarGoogle Scholar |
[127]
O. E. Craig ,
M. J. Collins ,
The removal of protein from mineral surfaces: implications for residue analysis of archaeological materials.
J. Archaeol. Sci. 2002
, 29, 1077.
| Crossref | GoogleScholarGoogle Scholar |
[128]
M. Keiluweit ,
P. S. Nico ,
M. G. Johnson ,
M. Kleber ,
Dynamic molecular structure of plant biomass-derived black carbon (Biochar).
Environ. Sci. Technol. 2010
, 44, 1247.
| Crossref | GoogleScholarGoogle Scholar | PubMed |