Subsoil testing required to detect the rundown of soil potassium to deficient levels for wheat production on loam-textured soils
Craig A. Scanlan

A
B
C
D
Abstract
Long-term negative potassium (K) balances in crop production have depleted soil K levels in Western Australia (WA). Previous research has focussed on sand-textured soils, but recently, monitoring of crops grown on loam-textured soils has shown deficient or marginal shoot K concentrations where Colwell K 0–10 cm is above current critical levels.
The aims were to examine whether grain yield responses to fertiliser K can be detected on loam-textured soils and if soil test calibration curves can be identified for these soils.
Eight field trials were conducted with wheat on loam-textured soils. The same experimental design was used at all sites; six levels of K applied at sowing, from 0 to 200 kg K ha−1 with one treatment including a split application. Soil and plant test calibration curves were modelled using measurements from the trials.
Grain yield responses of 0.69 to 1.37 t ha−1 to fertiliser K (P < 0.05) occurred in 4 of 8 trials. Relative yield was closely related to soil exchangeable K and the goodness of fit of the soil test calibration curves increased as the depth of sampling increased. The best soil test calibration curve was for sampling 0–40 cm.
This research confirms that on some loam-textured soils, yield loss is occurring to K deficiency if no K fertiliser is applied.
As soil K reserves are run down, soil sampling at 0–40 cm on loam-textured soils will provide the most accurate monitoring of soil K deficiency for wheat production.
Keywords: exchangeable potassium, plant analysis, potassium, soil analysis, soil test calibration, wheat.
Introduction
Long term rundown of soil potassium (K) reserves in agricultural soils is common globally (Brownlie et al. 2024; Wang et al. 2024). Negative K balances (removal in harvested products exceeds inputs) over decades have been reported for Australia, India, sub-Saharan Africa and the southern cone of Latin America (Majumdar et al. 2021; Das et al. 2022; Ma et al. 2022). Where plant-available K is above the level where deficiency occurs, negative K balances are an economically rational approach because there is no immediate economic benefit from applying fertiliser K. However, this approach relies on accurate predictions by empirical models to identify when plant-available K has been depleted to deficient levels and a profitable response to fertiliser K is likely.
Soil testing for plant-available K is an important tool for monitoring soil K rundown and predicting when K deficiency is limiting the growth of crops or pastures. Soil test calibration curves are used to define the relationship between a soil test level and relative yield, and to quantify critical levels (and critical ranges), typically the soil test level required for 90% of maximum yield (Brennan and Bell 2013). Soil test calibration curves can differ between crop species, soil test method and soil type (Siatwiinda et al. 2024). The development of these calibration curves needs to include regionally relevant combinations of the factors above and be reassessed over time as soil conditions and production systems change.
Plant testing is an also a valuable tool for monitoring the nutrient supply from soils used for crop production. Like soil test calibration curves, plant test calibration curves relate relative yield to shoot nutrient concentration in whole shoots or specific shoot parts (Murrell and Pitchay 2021; Bell 2023), and the critical level is usually defined as the shoot nutrient concentration that relates to a relative yield of 90%. Plant test calibrations differ from soil testing in that the critical level is dependent on growth stage because the concentration required for maximum yield decreases as the growth stage increases (Bell 2023). Current critical levels for K in use for the major grain crops in Australia are derived from glasshouse (Brennan and Bolland 2004; Brennan and Bolland 2007) and field experiments (Brennan and Bolland 2006). Plant testing complements soil testing because it is possible to respond tactically with fertiliser application in the growing season when deficiencies are detected.
The occurrence of K deficiency in soils used for crop production in Western Australia (WA) has correlated with the native K status of the major soil groups used for agricultural production. Potassium deficiency symptoms were first detected in pastures grown on deep sands in the 1950s (Fitzpatrick and Dunne 1956; Drover 1961; Toms and Fitzpatrick 1961) and in crops grown on duplex soils approximately four decades later (Wong and Witwer 1997; Wong et al. 2000). Based on these findings, research on K nutrition of grain crops has focussed on soils with a sand texture at the surface; 77% of trials in a national database were done on sandy soils in WA (Brennan and Bell 2013). Recently, our monitoring of shoot nutrient concentrations has revealed shoot K concentrations that are low, or marginal in comparison to critical shoot concentrations (Reuter and Robinson 1997) in crops grown on loam-textured soils where Colwell K for 0–10 cm depth was above the current critical soil test levels, for example 40 mg kg−1 in Chromosols (Brennan and Bell 2013). The aims of this study were to examine whether (1) K deficiency is emerging on loam-textured soils via grain yield or quality responses to fertiliser and (2) if a soil test calibration curve can be identified for these soils.
Methods
Growing environment
Eight field trials were located in the Avon sub-region of the Avon River basin (Galloway 2004) in Western Australia (WA). The Avon sub-region was chosen for this series of experiments because of the prevalence of loam-textured soils used for grain production in that region. The climate in that region is Mediterranean, characterised by hot, dry summers and cool, wet winter winters. In this region, sowing usually occurs in late April to mid-May (mid to late Autumn) and harvest in November (late spring) (Harries et al. 2020), with one crop grown per year. The field experiments were conducted in 2021 and 2022. Growing season rainfall (April to October) ranged from 289 to 475 mm at the sites (Table 1).
Location | GSRF (mm) | |
---|---|---|
York S 2022 | 289 | |
York N 2022 | 382 | |
Beverley E 2021 | 475 | |
York N 2021 | 445 | |
Quairading W 2022 | 404 | |
Beverley E 2022 | 399 | |
Greenhills S 2021 | 406 | |
Greenhills E 2021 | 436 |
Trial sites are ordered in this table to match ordering by Colwell K concentrations (0–50 cm depth) in Table 3.
Soils at the trial sites were classified as Chromosols, Kandosols or Dermosols (Table 2) in the Australian Soil Classification (ASC) system (Isbell and the National Committee on Soil and Terrain 2021). The corresponding classifications using the Western Australian Soil Group (WASG) system (Schoknecht and Pathan 2013) are also shown in Table 2. The texture of the A1 horizon was sandy loam or loamy sand at all sites (Table 2). Colwell K for 0–10 cm depth ranged 25 to 231 mg kg−1 (Table 3), and was mostly above the current critical ranges for Chromosols (35–45 mg kg−1) and Kandosols (45–52 mg kg−1) (Brennan and Bell 2013). Cation exchange capacity (CEC) for 0–10 cm depth ranged 3 to 15 molc+ kg−1. Sodium tetraphenylboron K (TPB-K) (0–10 cm) ranged 51 to 452 mg kg−1, which is at the lower end of the range of TPB-K values in a recent study of WA cropping soils (46 to 1582 mg kg−1) (Cheng et al. 2023). The sites were selected based on the detection of marginal or deficient shoot K concentrations (Reuter and Robinson 1997) in crops grown in those paddocks in the previous 2 years, on soil types that were loam textured.
Location | ASC | WASG | A1 texture | |
---|---|---|---|---|
York S 2022 | Reticulate, Dystrophic, Brown Chromosol | Reticulate deep sandy duplex | Loamy sand | |
York N 2022 | Ferric Mesotrophic Kandosol | Red loamy earth | Sandy loam | |
Beverley E 2021 | Ferric-sodic Mesotrophic Red Kandosol | Red loamy earth | Sandy loam | |
York N 2021 | Ferric Mesotrophic Kandosol | Red loamy earth | Sandy loam | |
Quairading W 2022 | Mottled, Subplastic, Brown Chromosol | Yellow/brown deep sandy duplex | Loamy sand | |
Beverley E 2022 | Ferric-sodic, Mesotropic, Red Chromosol | Red deep sandy duplex | Loamy sand | |
Greenhills E 2021 | Sodic Calcic Brown Dermosol | Brown loamy earth | Sandy loam | |
Greenhills S 2021 | Acid-mottled Dystrophic Kandosol | Red loamy earth | Sandy loam |
Hand texture of the A1 horizon was done by an experienced pedologist. Trial sites are ordered in this table to match ordering by Colwell K concentrations (0–50 cm depth) in Table 3.
York S 2022 | York N 2022 | Beverley E 2021 | York N 2021 | Quairading W 2022 | Beverley E 2022 | Greenhills S 2021 | Greenhills E 2021 | ||
---|---|---|---|---|---|---|---|---|---|
Col K (mg kg−1) | |||||||||
0–10 | 25 | 53 | 74 | 80 | 42 | 105 | 161 | 231 | |
10–20 | 21 | 31 | 41 | 41 | 32 | 63 | 62 | 117 | |
20–30 | 24 | 26 | 34 | 35 | 43 | 57 | 68 | 116 | |
30–40 | 30 | 20 | 31 | 33 | 64 | 36 | 41 | 113 | |
40–50 | 27 | 18 | 27 | 27 | 62 | 38 | 32 | 124 | |
TPB-K (mg kg−1) | |||||||||
0–10 | 51 | 85 | 115 | 115 | 52 | 197 | 184 | 452 | |
10–20 | 40 | 63 | 102 | 84 | 42 | 178 | 75 | 332 | |
20–30 | 43 | 64 | 98 | 91 | 57 | 186 | 83 | 373 | |
30–40 | 59 | 52 | 105 | 98 | 87 | 164 | 60 | 290 | |
40–50 | 70 | 44 | 102 | 96 | 80 | 126 | 42 | 392 | |
CEC (molc+ kg−1) | |||||||||
0–10 | 6 | 6 | 8 | 8 | 4 | 6 | 3 | 15 | |
10–20 | 2 | 6 | 5 | 7 | 1 | 5 | 3 | 15 | |
20–30 | 1 | 8 | 6 | 8 | 1 | 6 | 3 | 15 | |
30–40 | 1 | 7 | 6 | 7 | 2 | 5 | 3 | 19 | |
40–50 | 2 | 7 | 6 | 7 | 2 | 6 | 4 | 21 | |
pH (CaCl2) | |||||||||
0–10 | 5.9 | 5.1 | 6.0 | 5.7 | 6.0 | 5.6 | 4.5 | 6.0 | |
10–20 | 4.7 | 4.8 | 4.8 | 4.9 | 5.4 | 5.3 | 4.5 | 5.8 | |
20–30 | 4.6 | 5.4 | 5.8 | 5.4 | 5.3 | 6.0 | 4.8 | 6.0 | |
30–40 | 4.8 | 5.5 | 5.7 | 5.5 | 5.4 | 6.0 | 5.2 | 6.3 | |
40–50 | 5.1 | 5.8 | 6.0 | 5.6 | 5.2 | 6.3 | 5.6 | 6.0 | |
OC (%) | |||||||||
0–10 | 1.0 | 1.6 | 1.3 | 2.2 | 0.8 | 1.0 | 1.3 | 1.9 | |
10–20 | 0.5 | 1.1 | 0.6 | 1.2 | 0.4 | 0.7 | 0.6 | 1.3 | |
20–30 | 0.2 | 0.9 | 0.4 | 0.8 | 0.3 | 0.9 | 0.5 | 0.7 | |
30–40 | 0.3 | 0.6 | 0.2 | 0.6 | 0.3 | 0.3 | 0.4 | 0.5 | |
40–50 | 0.2 | 0.6 | 0.2 | 0.3 | 0.3 | 0.3 | 0.3 | 0.4 |
Values shown are the mean of six replicates. Sites are ordered left to right based on mean Colwell K concentration (0–50 cm depth).
Col K, Colwell K; TPB-K, sodium tetraphenylboron K; CEC, cation exchange capacity; OC, organic carbon.
Field experiments
The same design was used for each trial. Six K treatments were applied, supplying from 0 to 200 kg K ha−1 (Table 4). All treatments were applied as fertiliser grade potassium chloride (KCl, 50% K) and were broadcast in front of the tines while sowing with a cone seeder, incorporating it into the top few cm of soil due to lateral soil throw by the narrow point openers (Barr et al. 2019). The trials were arranged as randomised complete block designs with six replicates. Wheat (Triticum aestivum) cv. Chief was sown at Greenhills E 2021 at 80 kg ha−1 and wheat (Triticum aestivum) cv. Scepter was sown at 80 kg ha−1 at the other trials. The trials were sown with plot seeders fitted with tines and press wheels at 22 cm row spacing. Plots were 16 m in length at York N 2022 and 20 m in length for the other trials. All seven rows were harvested with a plot harvester at maturity and a subsample of grain was retained from each plot for grain quality analysis.
Treatment | K applied at sowing | K applied 8 weeks after sowing | |
---|---|---|---|
K0 | 0 | ||
K25 | 25 | ||
K50 | 50 | ||
K25 + 25 | 25 | 25 | |
K100 | 100 | ||
K200 | 200 |
All treatments were applied as muriate of potash (MOP) fertiliser (KCl, 50% K) and were applied to the soil surface. Values shown are kg K ha−1.
Agronomic management of the trials was aligned to current management by grain growers in WA. Weeds were controlled by knockdown herbicides prior to sowing and by post emergence herbicide applications (Harries et al. 2020). Monoammonium phosphate fertiliser was drilled below the seed at sowing at 68 kg ha−1 (15 kg P ha−1, 8 kg N ha−1). The target nitrogen (N) fertiliser rate (80 kg N ha−1) was slightly above the level applied in this region by grain producers (71 kg N ha−1) (Planfarm 2021), to provide K-response data that were representative for current cropping systems. Nitrogen was surface-applied at sowing (40 kg N ha−1 as urea), at 4 weeks after sowing (20 kg N ha−1 as urea–ammonium nitrate), and at 8 weeks after sowing (20 kg N ha−1 as urea). However, additional N fertiliser (20 kg N ha−1 as urea) was also applied at 10 weeks after sowing in 2021 because cumulative rainfall at that time was in decile 9 at all sites.
Soil and plant measurements
Soil samples were collected from each replicate for chemical analysis. Three cores were taken from each of the six replicates at 0–10, 10–20, 20–30, 30–40 and 40–50 cm depth and were combined into three replicates for measurement; Replicates 1 and 2 were combined, 3 and 4 were combined, and 5 and 6 were combined. The replicate data mentioned below are for the combined samples. Soil samples were dried at 40°C in a forced-draught oven before passing through a 2 mm sieve. Soil samples were analysed according to Rayment and Lyons (2011) for organic carbon [OC] (mg kg−1) [Method 6A1], pH (CaCl2) [Method 4B4], Colwell phosphorus (mg kg−1) and Colwell K (mg kg−1) [Methods 9B and 18A1], extractable sulfur (mg kg−1) [Method 10B3], ammonium acetate exchangeable K [AA-K] (molc+ kg−1) [Method 15D3], TPB-K (mg kg−1) [Method 18G1, 4 hour incubation] and ammonium chloride and barium chloride exchangeable K [Exch-K] (molc+ kg−1) [Method 15E1]. Cation exchange capacity (CEC) was calculated as the sum of ammonium chloride and barium chloride exchangeable calcium (Ca), magnesium (Mg), K and sodium (Na). Percent exchangeable K was calculated from CEC and Exch-K. AA-K, Exch-K and Colwell K variously measure solution K and exchangeable K with different procedures; AA-K uses 1 M ammonium acetate buffered at pH 7 with a soil:solution of 1:10 for 30 min, Exch-K uses 0.1 M NH4Cl and BaCl2 with a soil:solution of 1:10 for 2 h and Colwell K uses 0.5 M NaHCO3 buffered at pH 8.5 with a soil:solution of 1:100 for 16 h. TPB-K measures solution K, exchangeable K and non-exchangeable K.
Plant measurements were done on whole shoot samples collected at early stem elongation (Growth stage 31, Zadoks et al. 1974) and mid-flowering (Growth stage 65, Zadoks et al. 1974), and on grain sub-samples at maturity (Growth stage 92, Zadoks et al. 1974). Shoot samples at stem elongation were only sampled in 2021. Whole shoot samples were dried at 60°C in a forced-draught oven, weighed, and ground for chemical analysis. Nutrient concentrations were determined by Inductively Coupled Plasma–Atomic Emission Spectrometry (ICP-AES) (McQuaker et al. 1979). Grain protein and test weight (kg hL−1) were measured with a Foss Infratec Nova grain analyser as these parameters affect wheat grain grade and price (e.g. Grains Industry Association of Western Australia 2024). Plant data were combined into three replicates, to align with the soil data, for the analysis of soil test-relative yield relationships below.
Calculated properties
Relative yield (RY) was calculated for each replicate based on grain yield in the nil K treatment and grain yield in the highest-yielding treatment:
Statistical analysis
Treatment effects on grain yield, protein and test weight were assessed using analysis of variance (ANOVA) with Genstat (VSN International 2022). Where significant (P < 0.05) treatment effects were detected, means were separated using Fisher’s protected least significant difference. Correlations between soil properties within a depth layer, and between layers were assessed using the ggcorrplot package (Kassambara 2019) in R (R Core Team 2022). Deming regression was used to assess the relationships between soil K measurements, because both the X and Y data have measurement error, using the SimplyAgree package (Caldwell 2022) in R (R Core Team 2022).
Soil test calibration curves were assessed using the modified arcsine-log calibration curve (ALCC) method (Correndo et al. 2017) in the soiltestcorr package (Correndo et al. 2023) in R (R Core Team 2024). This method transforms the RY (arcsine of square root) and soil test (natural log) data, then flips the axes so that soil test value is the dependent variable. The Pearson correlation coefficient (r-value) for the transformed soil test-RY data are calculated. The transformed RY data are centred to the target RY, then standardised major axis (SMA) regression is used to estimate the linear relationship between the two variables. The critical soil test value (CSTV) is where the SMA regression intercepts x = 0 (x axis data centred to the target RY). The back-transformed regression model is used to predict the calibration curve and to calculate root mean square error for the data being analysed. Due to the relatively small data set used in this study, bootstrapping (n = 1000) was implemented using replicate data from each trial to provide more robust estimates of the r-value, CSTV and RMSE for the calibration curves using 90% confidence intervals (CI). Calibration curves were modelled for a target RY of 90%.
Plant test calibration curves were also assessed using the soiltestcorr package. The ALCC model was used for the plant test calibration curve because the same considerations apply as with soil test data; plant test data are an observation from the experiment which has an error component, rather than a fixed treatment, so the regression model needs to suit a bivariate relationship. Bootstrapping was also implemented for the plant test calibration curves. The K25 + 25 treatment was omitted from the analysis of stem elongation data because the second application of 25 kg K ha−1 was applied close to the time of plant sampling and would not have affected shoot K concentration.
Results
Crop growth response to potassium treatments
Treatment effects on grain yield occurred at 4 of the 8 sites (Table 5). At 2 of the 4 sites where a treatment effect occurred for grain yield, the lowest level of K applied, K25, was higher than the K0 treatment (Table 5). At the other two sites where a response occurred, K25 + 25 was required to increase grain yield above K0. At 3 of these four sites, grain yield increases were detected up to 200 kg K ha−1.
Location | K0 | K25 | K50 | K25 + 25 | K100 | K200 | l.s.d. (5%) | |
---|---|---|---|---|---|---|---|---|
York S 2022 | 3.08a | 3.77b | 3.72b | 3.76b | 3.89b | 3.83b | 0.31 | |
York N 2022 | 5.37a | 5.84b | 6.21c | 6.07c | 6.50d | 6.74e | 0.18 | |
Beverley E 2021 | 4.38a | 4.51ab | 4.64abc | 4.76bc | 4.89c | 5.27d | 0.27 | |
York N 2021 | 4.84a | 4.92ab | 4.99ab | 5.11b | 5.14b | 5.53c | 0.24 | |
Quairading W 2022 | 4.92 | 5.00 | 4.98 | 5.14 | 5.13 | 5.12 | P = 0.17 | |
Beverley E 2022 | 5.68 | 5.89 | 5.82 | 5.74 | 5.77 | 5.8 | P = 0.95 | |
Greenhills S 2021 | 4.38 | 4.40 | 4.51 | 4.27 | 4.45 | 4.50 | P = 0.47 | |
Greenhills E 2021 | 6.13 | 6.05 | 6.18 | 6.10 | 6.12 | 6.16 | P = 0.94 |
Treatments within a trial with different letters are significantly different (P < 0.05). l.s.d. = least significant difference. Trial sites are ordered in this table to match ordering by Colwell K concentrations (0–50 cm depth) in Table 3.
Potassium application affected grain quality at two sites but the effects were small. At York N 2022, grain protein in K100 and K200 treatments was 8.4% and 8.6%, respectively, higher than 8.2% in K0. The site mean for grain protein at the other seven sites ranged from 8.2% to 9.0%. At Beverley E 2021, test weight in K25 + 25 and K200 treatments was 84.3 and 84.2 kg hL−1, respectively, higher than 83.5 kg hL−1 in K0. The site mean for test weight at the other seven sites ranged 81.1 to 85.9 kg hL−1.
Relationships between soil chemical properties
Measurements of soil K were highly correlated among methods of extraction and between soil layers. The Pearson correlation coefficient for Colwell K and Exch-K or AA-K was greater than or equal to 0.95 at all soil depths, and for Exch-K or AA-K was 0.99 or 1 at all soil depths (Data for 0–10 cm shown in Fig. 1). Deming regression showed that Colwell K was about 60% of TPB-K at 0–10 cm depth, and about 30% of TPB-K at 20–30 cm, 30–40 cm and 40–50 cm (Fig. S1). Colwell K was 20% to 30% higher than Exch-K or AA-K at 0–10 cm and 10–20 cm, 10% higher at 20–30 cm and equivalent at 30–40 cm and 40–50 cm (Fig. S2, S3). Colwell K for each depth was highly correlated (r > 0.8) with depth increments that were adjacent (above or below) but less so for the 0–10 cm vs 30–40 or 40–50 cm depths (Table S1).
Correlation plot for selected soil chemical properties (0–10 cm depth) at the trial sites. Values shown are the Pearson correlation coefficient. Variables are grouped based on their correlations using hierarchical clustering. The symbol ‘X’ shows correlations for which P > 0.05. Correlation plot was produced using the ggcorrplot package in R (Kassambara 2019).
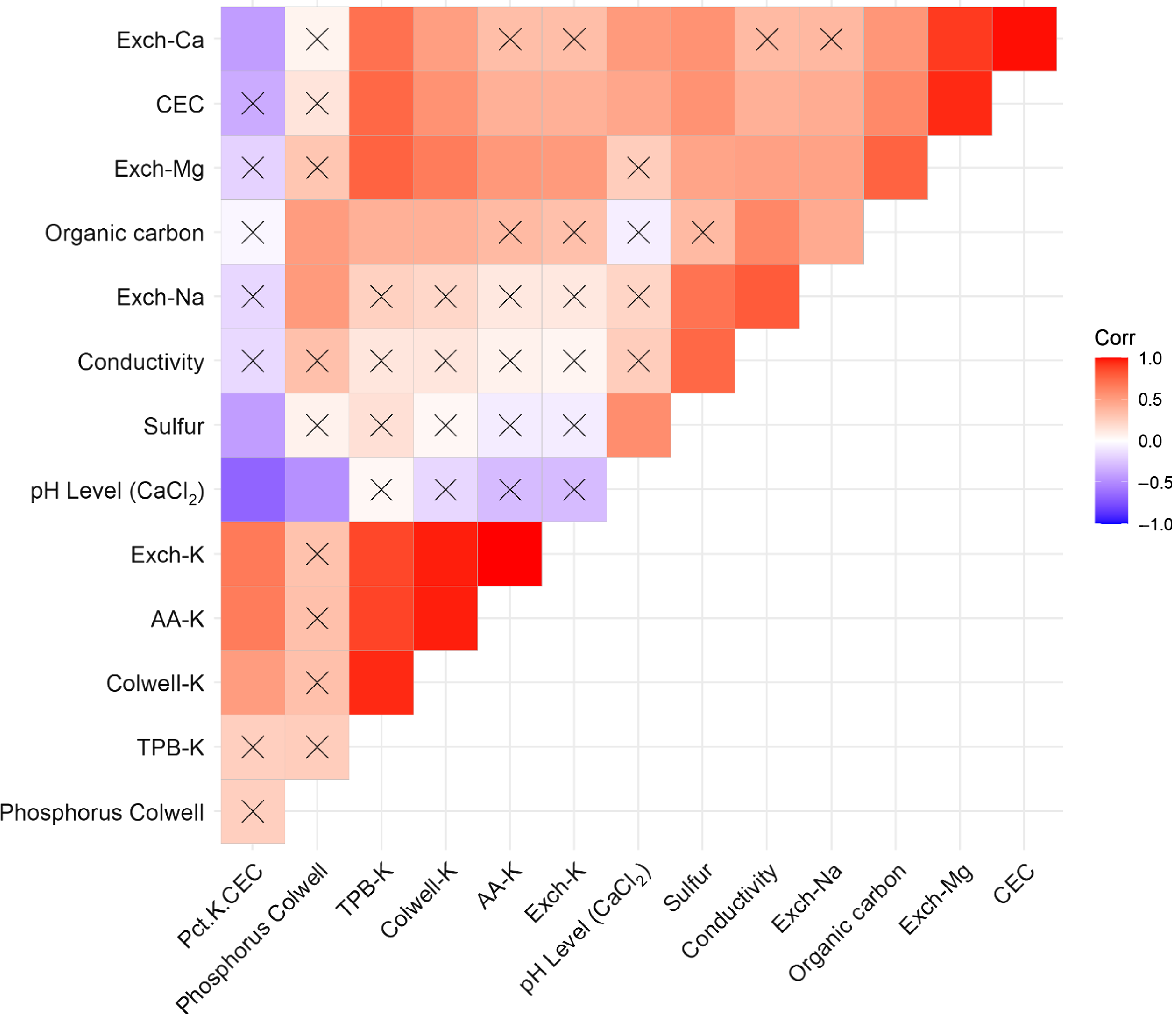
Comparison of soil test calibration curves for different sampling depths and soil properties
Overall, AA-K, Exch-K and Colwell K provided the best calibration curves (Fig. 2). Based on the values within, and width of the 90% CI for r-value and RMSE, the calibration curves improved as sampling depth increased for AA-K, Exch-K and Colwell K, worsened as sampling depth increased for PCT-K, and did not change with sampling depth for the other soil variables.
90% confidence intervals obtained from the bootstrap samples for r-value and root mean square error (RMSE) for the arcsine-log calibration curves that relate soil properties for increasing depth intervals to crop relative grain yield. r-value = Pearson correlation coefficient of the transformed soil test and RY data. AA-K = ammonium acetate exchangeable K, Exch-K = ammonium chloride and barium chloride exchangeable K, TPB-K = sodium tetraphenylboron K, PCT-K was calculated from CEC and Exch-K, pH = soil pH (CaCl2), CEC = sum of ammonium chloride and barium chloride exchangeable calcium, magnesium, K and sodium, OC = organic carbon. Soil properties are ordered top to bottom using median r-values for soil properties.
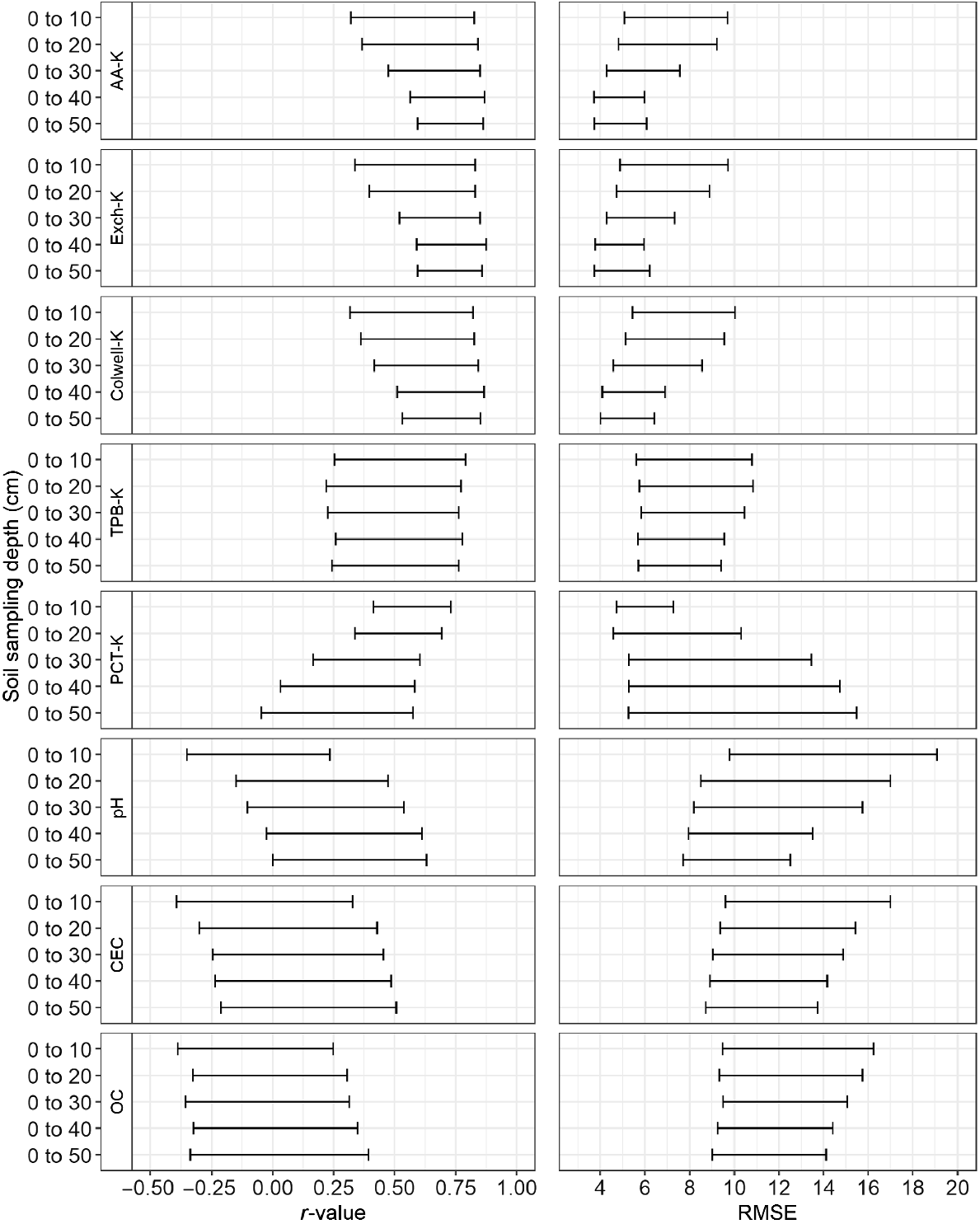
For AA-K, Exch-K and Colwell K, there was a marginal improvement in the 90% CIs for r-value and RMSE as sampling depth increased from 0–10 cm to 0–20 cm, and 0–20 to 0–30 cm, a larger improvement from 0–30 to 0–40 cm, then a marginal improvement from 0–40 to 0–50 cm. For example, the 90% CIs for r-value for AA-K 0–10, 0–20, 0–30, 0–40 and 0–50 cm were 0.32–0.83, 0.37–0.84, 0.47–0.85, 0.56–0.87 and 0.59–0.86, respectively. The 90% CIs for RMSE for AA-K 0–10, 0–20, 0–30, 0–40 and 0–50 cm were 5.1–9.7, 4.8–9.2, 4.3–7.6, 3.7–6.0 and 3.7–6.1, respectively. Our analysis suggests that to gain a meaningful improvement in the soil test calibration curve for AA-K for 0–10 cm, sampling 0–40 cm is required. The calibration curves for AA-K, Exch-K and Colwell for 0–40 cm depth were similar. The 90% CIs for r-value for Exch-K and Colwell K 0–40 cm were 0.59–0.88 and 0.51–0.87, respectively, and the 90% CIs for RMSE for Exch-K and Colwell K were 3.8–6.0 and 4.1–6.9, respectively. Our analysis below focusses on Colwell K because it provides a similar level of accuracy to AA-K and Exch-K and is an industry standard for soil K analysis in Australia.
The soil test calibration curves for Colwell K for the different sampling depths are shown in Fig. 3. This figure illustrates the improvement in the goodness of fit of the calibration curves as sampling depth increases. The CSTV for the 0–20 and 0–30 cm sampling depth was not different to that for 0–10 cm, however, CSTV for 0–40 cm sampling depth is significantly lower than 0–10 cm.
Comparison of soil test calibration curves for Colwell K at different soil sampling depths. Data shown are the relative yield (RY) and Colwell K for replicates within the field trials. Soil test calibration curves were modelled with the arcsine-log calibration curve (ALCC) method in the soiltestcorr package (Correndo et al. 2023) in R (R Core Team 2024). r-value = Pearson correlation coefficient of the transformed soil test and RY data, RMSE = root mean square error for RY, CSTV = critical soil test value for 90% RY. Values shown are the 90% confidence interval (CI) from the bootstrap samples. Dashed vertical lines are the 90% CI for CSTV. Dashed horizontal line is 90% RY.
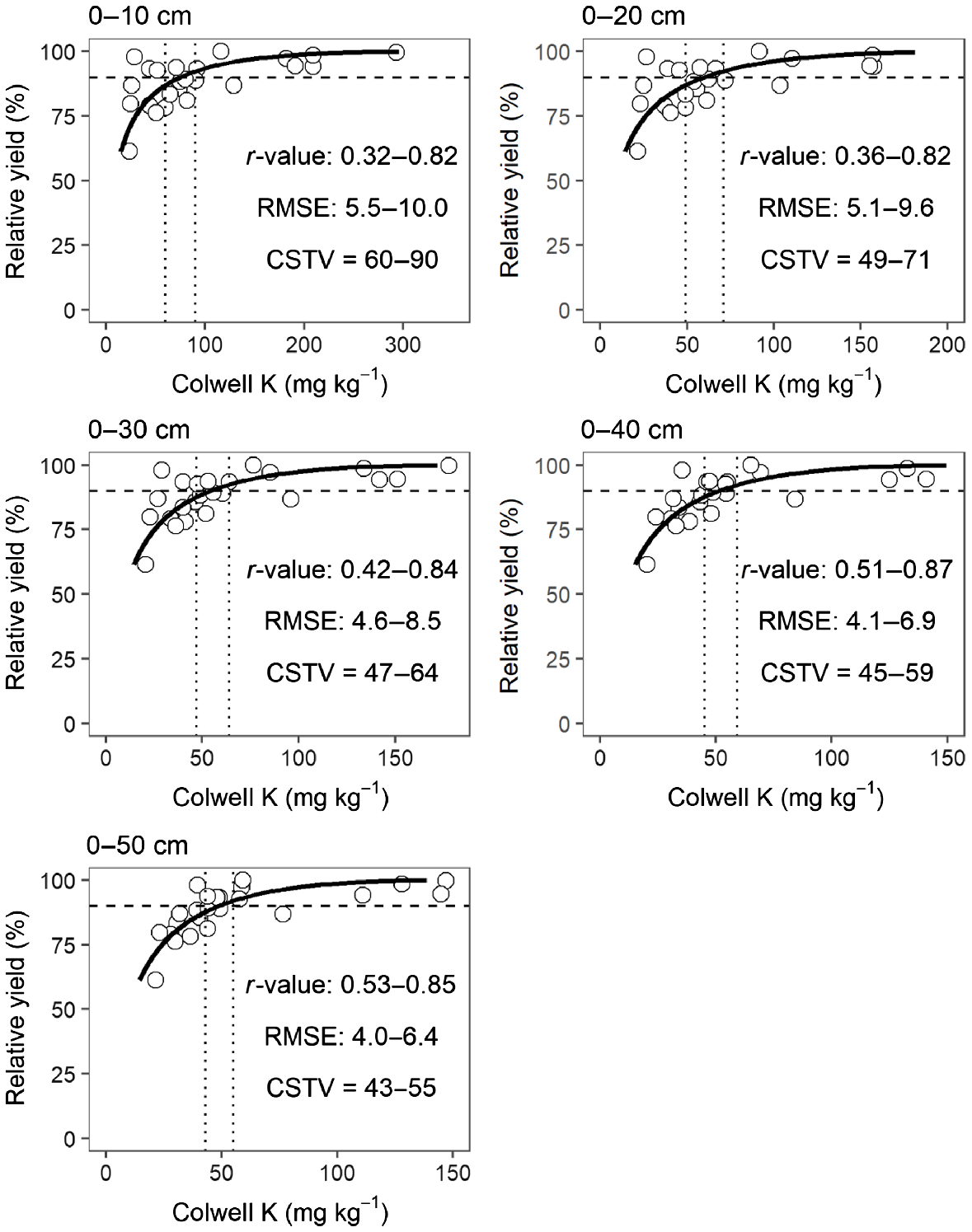
Comparison of yield responses with shoot K concentration
Relative yield showed a closer relationship with shoot K concentration at stem elongation than at flowering (Fig. 4). The 90% CI for r-value was higher for stem elongation sampling than flowering, and the 90% CI for RMSE was lower for the stem elongation sampling than flowering.
Comparison of plant test calibration curves for shoot K concentration measured at stem elongation (a) and flowering (b). Data shown are the relative yield (RY) and shoot K concentration for each treatment and replicate within the trials. Plant test calibration curves were modelled with the arcsine-log calibration curve (ALCC) method in the soiltestcorr package (Correndo et al. 2023) in R (R Core Team 2024). r-value = Pearson correlation coefficient of the transformed plant test and RY data, RMSE = root mean square error for RY, CPTV = critical plant test value for 90% RY. Values shown are the 90% confidence interval (CI) from the bootstrap samples. Dashed vertical lines are the 90% CI for CPTV. Dashed horizontal line is 90% RY.
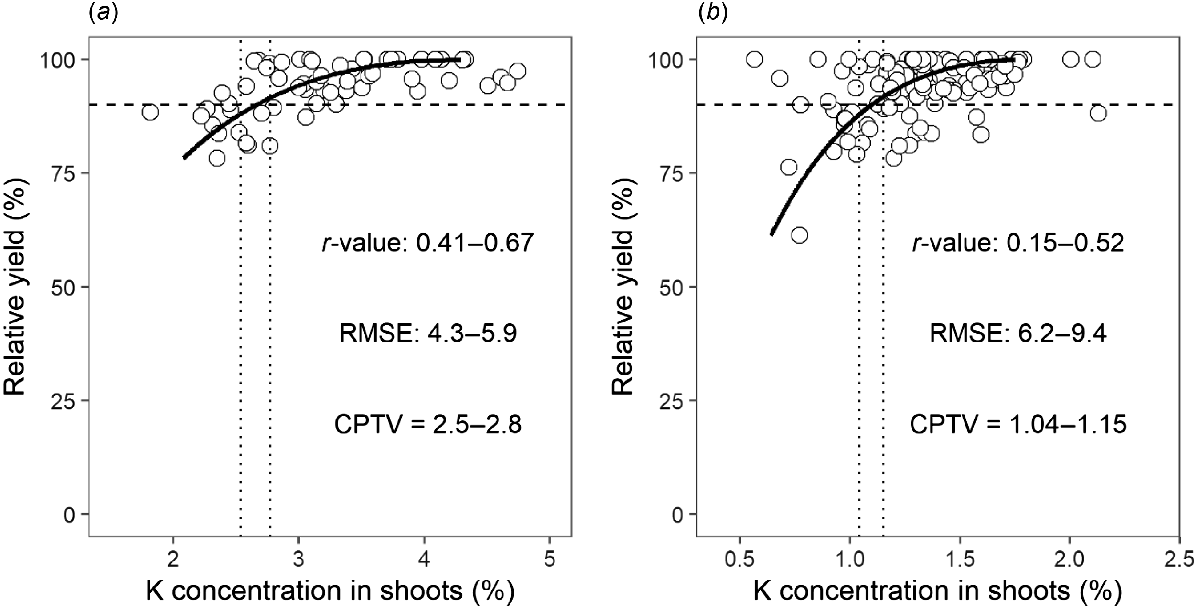
Discussion
Significant grain yield responses of 0.69 to 1.37 t ha−1 to fertiliser K in 4 of 8 field experiments provided confirmation that on some loam-textured soils, grain yield loss is occurring to K deficiency if no K fertiliser is applied. This is the first report of K deficiency for wheat on these soils in WA.
The relationship between yield response to fertiliser K and methods that measure exchangeable K identified in our analysis is in agreement with a glasshouse study using a wider range of soils (Cheng et al. 2023). In our study, the 90% CI for r-value and RMSE were similar for AA-K, Exch-K and Colwell at each depth, meaning that any of these methods could be used to develop reliable soil test calibration curves for the soil types included in this study. In the glasshouse study, wheat K uptake showed a better relationship with AA-K than Colwell K, but the difference in goodness of fit was small (ΔBIC, the change in the Bayesian information criterion, = 8). Combined, these studies show that Colwell K is adequate for measuring plant-available K over a range of soils used for cropping in WA. Also, both studies showed closer relationships between crop growth (K uptake in the glasshouse study, RY in the current study) and Colwell K or AA-K than with measurements of non-exchangeable K. The poorer fit of calibration curves for TPB-K compared to AA-K, Exch-K or Colwell K in this study indicate that non-exchangeable K is currently not a major component of K supply to crops on these soils.
Increasing sampling depth improved the goodness of fit of soil test calibration curve. The 90% CI for r-value for the calibration curve for Colwell K at 0–10 cm depth in the current study was 0.32–0.82, overlapping the value for Chromosols (r = 0.46, n = 46) and Kandodols (r = 0.78, n = 37) in a national study (Brennan and Bell 2013). Our results showed a marginal improvement in the soil test calibration curve by sampling 0–20 cm, or 0–30 cm compared to 0–10 cm, but a more meaningful improvement when sampling 0–40 cm, compared to 0–10 cm. The improvement in the goodness of fit as sampling depth increases is attributed to crop uptake from both surface and subsoil layers. The contribution of K from the subsoil to total uptake can be significant; on average 34% of K uptake by wheat was derived from the subsoil (Kuhlmann 1990), and it can change the grain yield response to K fertiliser on soils with low exchangeable K in the surface layer (Scanlan et al. 2015).
The rundown of subsoil K in long-term experiments provides further evidence of the contribution of K from subsoils to crop uptake. The depletion of AA-K and TPB-K stocks was greater in subsoils (20–100 cm) than topsoils (0–20 cm) over 9 years of continuous cropping (Correndo et al. 2021). In a 16-year study, the annual rate of decline (mg kg−1 year−1) for AA-K and nitric acid-K at 20–40 cm was the same as for 0–20 cm. These results, in combination with the improvement in calibration curve with increased sampling depth, suggest that sampling to 40 cm on loam-textured soils, at intervals several years apart e.g. 5 years, is required to monitor the rundown of soil K reserves.
The impact of subsoil K on soil test calibrations in previous studies in WA is mixed. Brennan and Bolland (2006) found that the threshold of Colwell K for a response to fertiliser K was similar for 0–10, 10–20 and 20–30 cm. However, we reanalysed their data using the same approach as in this study (ALCC method for mean soil test values for sampling depth, confidence intervals calculated from bootstrap samples) and found that the calibration curve improved as sampling depth increased (Fig. S4). The improvement in goodness of fit as sampling depth increases supports sampling 0–20 cm for canola to provide a more reliable prediction of relative yield than 0–10 cm. However, in a previous study with wheat conducted on Chromosols, Wong et al. (2000) found that a depth-weighted function for the soil K profile K did not provide a better prediction of relative yield than Colwell K at 0–10 cm depth alone. In the study by Wong et al. (2000), Colwell K at 0–10 cm depth was less than 50 mg kg−1 at all sites and was less than 50 mg kg−1 below 10 cm depth for 8 of the 10 sites. It is likely that the greater range of Colwell K concentrations at all depths in our current study and the study by Brennan and Bolland (2006) has allowed the impact of subsoil K on grain yield response to fertiliser K to be detected.
The 90% CI for Colwell K at 0–10 cm depth in our current study (60–90 mg kg−1) is higher than reported for wheat in a national analysis based on Colwell K (Brennan and Bell 2013). The CSTV (and range) was 45 mg kg−1 (41–49) for all soils in the national study (n = 201) and ranged from 41 mg kg−1 (32–52) for Tenosols (n = 70) to 64 mg kg−1 (57–70) for Brown Ferrosols (n = 18). The 90% CI for Colwell K (0–10 cm) in this study only overlapped with Brown Ferrosols in the national study. The reason for the higher critical level in this study is not clear. It may be due to higher yields in this study than in previous research, but the impact of grain yield potential was not consistent in the national study; critical level was influenced by yield level in Tenosols but not Kandosols (Brennan and Bell 2013). In previous work on N × K interactions for wheat, RY was lower when 138 kg N ha−1 was applied [RY = 0.65] compared to the nil N control [RY = 0.81] (Fig. 1, Brennan and Bolland 2009). The higher critical level may be due to higher levels of CEC in this study, but it was not reported in the national study, so the relationship between CEC and critical level over the historical and current data cannot be assessed. The influence of sampling depth on CSTV was not reported for the national study, so it’s not possible to compare this aspect of sampling between the two studies either.
The long-term rundown in Colwell K and negative K balances in all soils of the cropping regions in WA (Harries et al. 2021; Ma et al. 2022) suggests that the incidence of K deficiency on coarse (sand, loamy sand, sandy loam) and medium (loam, silt loam, silt, sandy clay loam) textured soils will increase in the future. As a result, there will be increasing demand for empirical models to include a wider range of soils than in the current study. Future research designed to calibrate these models should include measurements of soil properties that are likely to influence K buffering; the proportions of minerals present in the clay fraction (Breker et al. 2019), clay content and CEC (Gourley et al. 2019). Although RY was not closely related to TPB-K in this study, we recommend this measurement be included in future work as the influence of non-exchangeable K on crop response to fertiliser K may increase as exchangeable K is further depleted and as a wider diversity of loamy and clay-textured soils are assessed. Also, the influence of fertiliser K placement should be examined; it is likely that there will be a benefit from banding compared to surface application on loam and clay-textured soils where CEC is greater than 15 molc+ kg−1 (Bell et al. 2021).
The rate of rundown of soil K levels from adequate to deficient on loam-textured soils will depend on the yields of grain removed but will be accelerated by hay production in the crop sequence. Based on the 5-year average grain yield in this region [DPIRD Agzone H3] (Planfarm 2019, 2020, 2021, 2022, 2023) and typical grain K concentrations in WA (Ma et al. 2022), annual removal for wheat, barley and canola would be 13, 15 and 16 kg K ha−1 per year respectively. In this region, dry matter yield for oaten hay is typically 6 to 8 t ha−1, removing 102 to 136 kg K ha−1 per year (Blackwood 2007). Previous work with WA soils has shown that cumulative K removal over six harvests of ryegrass was closely related to exchangeable K plus water-soluble K with relatively small changes in non-exchangeable K (Pal et al. 2001). The dependence on exchangeable K in WA cropping soils identified in previous work (Pal et al. 2001; Cheng et al. 2023) suggests that plant-available K in loam-textured soils is susceptible to rundown, but the rate of rundown, and equilibrium between K pools has not been characterised for these soils and should be addressed in future research. The equilibrium between these pools is critical, because this study has shown significant reserves of non-exchangeable K in the sub-surface layers in loam-textured soils; TPB-K levels were about three times Colwell K.
Our analysis of plant test calibrations curves showed that shoot K analysis at stem elongation is valuable for monitoring crop K status. Analysis of shoot K concentration at stem elongation provides some flexibility in K management; if a deficiency is identified with plant testing it is possible that a yield response to applying fertiliser K could occur at this stage (about 8 weeks after sowing) (Ma et al. 2015). The 90% CI for critical plant test value for the stem elongation calibration curve is higher than the value reported from a glasshouse study by Brennan and Bolland (2007) (2.25% K) for 90% RY, and for the flowering calibration curve, falls between the critical level in whole shoots at head emergence [Z50] (1.5% K) and milky-dough [Z75) (0.9% K) reported by Reuter et al. (1997). It’s possible that the higher critical level for stem elongation in the current study compared to previous research is due to higher yield levels, but there is not sufficient data to explore this relationship.
The emergence of K deficiency on loam-textured soils poses a new management challenge for grain production on these soil types: management of spatial variation. The loam-textured soils of the Avon sub-region are part of dissected landscapes where soil types vary from shallow sandy, gravelly surfaced soils in the upslope, to duplex soils with sandy-loam to loam-textured A horizons and sandy-clay to clay-textured B horizons in the mid-slope, and sand over clay duplex soils in valley floors (McArthur 2004). Airborne and ground-based radiometric surveys have potential for identifying the presence, or depth of ironstone gravel (Taylor et al. 2002; Pracilio et al. 2006; Wong et al. 2009) in these landscapes, which may have practical value in delineating soil zones that differ in K supply, but these relationships need to be investigated. It’s likely that a combination of visual cues – better growth on harvester windrows from the previous year (Brennan et al. 2004) or visual leaf symptoms of K deficiency (e.g. Murrell and Pitchay 2021) – and plant and soil sampling within soil management zones will be required to optimise the spatial management of K on these soils.
Conclusion
This study showed wheat grain yield response to the application of fertiliser K (P < 0.05) on loam-textured soils used for crop production in WA, confirming the emergence of K deficiency on these soils that were previously considered non-responsive to K. Wheat grain yield response to fertiliser K was related to exchangeable K but the soil test calibration curve for all measurements of exchangeable K improved as the depth of sampling increased. Soil testing to 40 cm depth was required for the most accurate prediction of relative yield. The improved prediction of relative yield with increased sampling depth is attributed to the contribution of subsoil K to total K uptake. Analysis of shoot K concentration at stem elongation provides complementary data to soil testing and can be used to guide in-season fertiliser K applications.
Data availability
The data that support this study will be shared upon reasonable request to the corresponding author.
Declaration of funding
This research was funded by the GRDC project ‘Increasing profit from N, P and K fertiliser inputs into the evolving cropping sequences in the Western Region’ (UMU1801-006RTX, UWA1801-002RTX).
Acknowledgements
Our thanks to the farmers who have provided us access to their properties for the initial plant testing and for field trials: Tristan O’Brien, Peter Monger, Leon Ryan, Jeremy Marwick, Andrew Reynolds, and Erin Emin. Thanks also to our colleagues at DPIRD who contributed to this work: Gavin Sarre, Zac Way, Pete Gray, Daron Malinowski, Bidhyut Banik, Trey Beeson, Shahab Pathan and Raj Malik. We also thank the CSBP field research team: Justin Mercy, Ryan Guthrie, and Miles Ellery.
References
Barr JB, Desbiolles JMA, Fielke JM, Ucgul M (2019) Development and field evaluation of a high-speed no–till seeding system. Soil and Tillage Research 194, 104337.
| Crossref | Google Scholar |
Bell MJ, Mallarino AP, Volenec J, Brouder S, Franzen DW (2021) Considerations for selecting potassium placement methods in soil. In ‘Improving potassium recommendations for agricultural crops.’ (Eds TS Murrell, RL Mikkelsen, G Sulewski, R Norton, ML Thompson) pp. 341–362. (Springer: Cham, Switzerland)
Breker JS, DeSutter T, Rakkar MK, Chatterjee A, Sharma L, Franzen DW (2019) Potassium requirements for corn in North Dakota: influence of clay mineralogy. Soil Science Society of America Journal 83, 429-436.
| Crossref | Google Scholar |
Brennan RF, Bell MJ (2013) Soil potassium-crop response calibration relationships and criteria for field crops grown in Australia. Crop & Pasture Science 64, 514-522.
| Crossref | Google Scholar |
Brennan RF, Bolland MDA (2004) Lupin takes up less potassium but uses the potassium more effectively to produce shoots than canola and wheat. Australian Journal of Experimental Agriculture 44, 309-319.
| Crossref | Google Scholar |
Brennan RF, Bolland MDA (2006) Soil and tissue tests to predict the potassium requirements of canola in south-western Australia. Australian Journal of Experimental Agriculture 46, 675-679.
| Crossref | Google Scholar |
Brennan RF, Bolland MDA (2007) Comparing the potassium requirments of canola and wheat. Australian Journal of Agricultural Research 58, 359-366.
| Crossref | Google Scholar |
Brennan RF, Bolland MDA (2009) Comparing the nitrogen and potassium requirements of canola and wheat for yield and grain quality. Journal of Plant Nutrition 32, 2008-2026.
| Crossref | Google Scholar |
Brennan RF, Bolland MDA, Bowden JW (2004) Potassium deficiency, and molybdenum deficiency and aluminium toxicity due to soil acidification, have become problems for cropping sandy soils in south-western Australia. Australian Journal of Experimental Agriculture 44, 1031-1039.
| Crossref | Google Scholar |
Brownlie WJ, Alexander P, Maslin M, Cañedo-Argüelles M, Sutton MA, Spears BM (2024) Global food security threatened by potassium neglect. Nature Food 5, 111-115.
| Crossref | Google Scholar |
Caldwell AR (2022) SimplyAgree: an R package and jamovi module for simplifying agreement and reliability analyses. Journal of Open Source Software 7, 4148.
| Crossref | Google Scholar |
Cheng M, Bell R, Brown J, Ma Q, Scanlan C (2023) Comparison of soil analytical methods for estimating plant-available potassium in highly weathered soils. Soil Research 61, 717-733.
| Crossref | Google Scholar |
Correndo AA, Salvagiotti F, García FO, Gutiérrez-Boem FH (2017) A modification of the arcsine–log calibration curve for analysing soil test value–relative yield relationships. Crop & Pasture Science 68, 297-304.
| Crossref | Google Scholar |
Correndo AA, Rubio G, García FO, Ciampitti IA (2021) Subsoil-potassium depletion accounts for the nutrient budget in high-potassium agricultural soils. Scientific Reports 11, 11597.
| Crossref | Google Scholar | PubMed |
Correndo AA, Pearce A, Bolster CH, Spargo JT, Osmond D, Ciampitti IA (2023) The soiltestcorr R package: an accessible framework for reproducible correlation analysis of crop yield and soil test data. SoftwareX 21, 101275.
| Crossref | Google Scholar |
Das D, Sahoo J, Raza MB, Barman M, Das R (2022) Ongoing soil potassium depletion under intensive cropping in India and probable mitigation strategies. A review. Agronomy for Sustainable Development 42, 4.
| Crossref | Google Scholar |
Drover DP (1961) The potassium status of some course textured soils of the south western region of Western Australia. Australian Journal of Experimental Agriculture and Animal Husbandry 1, 11-14.
| Crossref | Google Scholar |
Fitzpatrick EN, Dunne TC (1956) Potassium for subterranean clover. The Journal of Agriculture of Western Australia 5, 321-325.
| Google Scholar |
Gourley CJP, Weaver DM, Simpson RJ, Aarons SR, Hannah MM, Peverill KI (2019) The development and application of functions describing pasture yield responses to phosphorus, potassium and sulfur in Australia using meta-data analysis and derived soil-test calibration relationships. Crop & Pasture Science 70, 1065-1079.
| Crossref | Google Scholar |
Grains Industry Association of Western Australia (2024) Grain Standards. Available at https://www.giwa.org.au/industry-projects/grain-standards/
Harries M, Flower KC, Scanlan CA, Rose MT, Renton M (2020) Interactions between crop sequences, weed populations and herbicide use in Western Australian broadacre farms: findings of a six-year survey. Crop & Pasture Science 71, 491-505.
| Crossref | Google Scholar |
Harries M, Flower KC, Scanlan CA (2021) Sustainability of nutrient management in grain production systems of south-west Australia. Crop & Pasture Science 72, 197-212.
| Crossref | Google Scholar |
Kassambara A (2019) Package ‘ggcorrplot’. R package version 0.1 3, 908. 10.32614/CRAN.package.ggcorrplot
Kuhlmann H (1990) Importance of the subsoil for the K nutrition of crops. Plant and Soil 127, 129-136.
| Crossref | Google Scholar |
Ma Q, Bell R, Scanlan C, Sarre G, Brennan R (2015) Growth and yield responses in wheat and barley to potassium supply under drought or moderately saline conditions in the south-west of Western Australia. Crop & Pasture Science 66, 135-144.
| Crossref | Google Scholar |
Ma Q, Bell R, Scanlan C, Neuhaus A (2022) Long-term rundown of plant-available potassium in Western Australia requires a re-evaluation of potassium management for grain production: a review. Crop & Pasture Science 73, 981-996.
| Crossref | Google Scholar |
Majumdar K, Norton RM, Murrell TS, García F, Zingore S, Prochnow LI, Pampolino M, Boulal H, Dutta S, Francisco E, Tan MS, He P, Singh VK, Oberthür T (2021) Assessing potassium mass balances in different countries and scales. In ‘Improving potassium recommendations for agricultural crops.’ (Eds TS Murrell, RL Mikkelsen, G Sulewski, R Norton, ML Thompson) pp. 283–340. (Springer: Cham, Switzerland)
McQuaker NR, Brown DF, Kluckner PD (1979) Digestion of environmental materials for analysis by inductively coupled plasma–atomic emission spectrometry. Analytical Chemistry 51, 1082-1084.
| Crossref | Google Scholar |
Pal Y, Gilkes RJ, Wong MTF (2001) Soil factors affecting the availability of potassium to plants for Western Australian soils: a glasshouse study. Australian Journal of Soil Research 39, 611-625.
| Crossref | Google Scholar |
Pracilio G, Adams ML, Smettem KRJ, Harper RJ (2006) Determination of spatial distribution patterns of clay and plant available potassium contents in surface soils at the farm scale using high resolution gamma ray spectrometry. Plant and Soil 282, 67-82.
| Crossref | Google Scholar |
Scanlan CA, Bell RW, Brennan RF (2015) Simulating wheat growth response to potassium availability under field conditions in sandy soils. II. Effect of subsurface potassium on grain yield response to potassium fertiliser. Field Crops Research 178, 125-134.
| Crossref | Google Scholar |
Siatwiinda SM, Ros GH, Yerokun OA, de Vries W (2024) Options to reduce ranges in critical soil nutrient levels used in fertilizer recommendations by accounting for site conditions and methodology: a review. Agronomy for Sustainable Development 44, 9.
| Crossref | Google Scholar |
Taylor MJ, Smettem K, Pracilio G, Verboom W (2002) Relationships between soil properties and high-resolution radiometrics, central eastern Wheatbelt, Western Australia. Exploration Geophysics 33, 95-102.
| Crossref | Google Scholar |
Toms WJ, Fitzpatrick EN (1961) Potassium deficiency in medium rainfall areas. Journal of the Department of Agriculture, Western Australia 2, 1.
| Google Scholar |
Wang C, Xie Y, Tan Z (2024) Soil potassium depletion in global cereal croplands and its implications. Science of The Total Environment 907, 167875.
| Crossref | Google Scholar | PubMed |
Wong MTF, Edwards NK, Barrow NJ (2000) Accessibility of subsoil potassium to wheat grown on duplex soils in the south-west of Western Australia. Australian Journal of Soil Research 38, 745-751.
| Crossref | Google Scholar |
Wong MTF, Oliver YM, Robertson MJ (2009) Gamma-radiometric assessment of soil depth across a landscape not measurable using electromagnetic surveys. Soil Science Society of America Journal 73, 1261-1267.
| Crossref | Google Scholar |
Zadoks JC, Chang TT, Konzak CF (1974) A decimal code for the growth stages of cereals. Weed Research 14, 415-421.
| Crossref | Google Scholar |