A conceptual framework for modelling the role of livestock systems in sustainable diets and a sustainable planet
J. C. H. Dougherty



A
B
C
D
E
Abstract
The role of livestock in sustainable food systems and sustainable diets is a complex issue. It should be assessed in terms of its impacts on environmental, economic, and social sustainability, as well as the levels of animal performance, the human food supply, and the human food production system. However, such nuanced analyses are made difficult by the lack of multi-metric, multi-domain modelling frameworks and a lack of data on regional variation in livestock production. This paper proposes a conceptual biophysical modelling framework that could be used as a pathway to address existing methodology gaps and improve sustainability analyses across multiple levels. Realising this modelling framework requires clear, transparent, and enforceable frameworks for multi-scale sustainability assessments, as well as long-term investment into region-specific data collection, particularly from under-represented regions. To ensure representativeness and broader utility, this framework must also be able to model variation in both production systems and consumer dietary patterns, and the feedback loops between producer/consumer decisions and on-farm production. Beyond the level of science, this will also require concerted effort by the various actors in the livestock and food-chain sectors such as governmental bodies, the food production industry and local communities. Once realised, this framework could be used to assess trade-offs between potential food-system changes and to ensure that decisions are being made from a big picture, net-benefit perspective, while exploring methods for building flexible, diverse food systems that are sustainable across multiple scales.
Keywords: animal nutrition, human nutrition, life cycle assessment, livestock, modelling, sustainability, sustainable agriculture, sustainable diets, systems models, upcycling.
Introduction
Agriculture arose as a way for human societies to meet their food, fibre, work, and social needs; over time, economics emerged as an additional driver and constraint of agriculture. Modern society requires that agricultural products meet the nutritional needs of a growing global population while concurrently reducing the environmental impacts of food production in the face of increasing biophysical, economic and ethical constraints. Exploring the role of livestock in sustainable agriculture requires careful consideration of the nuances and trade-offs of different production systems from a multi-metric, multi-scale perspective, so that environmental impacts are considered along multiple scales, in conjunction with their contributions to the human food supply, economic security, and rural empowerment.
Big-picture assessments of sustainability and pathways to action are rare due to their complexity. The current literature is dominated by attributional studies that use a small number of metrics, predominantly carbon footprint (Picasso et al. 2014; Ridoutt et al. 2017; McClelland et al. 2018; Arvidsson Segerkvist et al. 2020; Harrison et al. 2021), to assess the sustainability of food production (i.e. the ‘carbon myopia’ discussed in Harrison et al. (2021)). The dynamic interconnectedness of global agriculture and the need to meet human dietary needs (while maintaining or improving agricultural impacts on the environment) requires big-picture, multi-level assessments. These assessments should examine the net impact of changes across a variety of levels and metrics to prevent improvement at one level hiding greater impacts in another level. Current methods also require revision to explicitly incorporate landscape and social aspects, reflecting the impacts of decisions on landscape/ecosystem health as well as the oft-neglected dimension of human choices and values. Such improvement requires looking beyond what current models are capable of, so as to build a robust, holistic approach that can model the impacts of human actions at a global scale in the context of ecosystem health and the health of the planet as a whole.
The aims of this review are therefore to (a) define and explore how conceptual frameworks can be used to assist in improving holistic, multi-scale assessment of the role and importance of livestock in both a sustainable food supply and a sustainable environment, (b) explore system challenges that need to be addressed to improve modelling of sustainable food production from a holistic perspective, (c) propose a conceptual modelling framework that could be used to address these challenges, and (d) identify gaps in data and perspectives that must be addressed to fully realise the proposed framework. This review will also discuss the role of modelling and sustainability frameworks in assisting stakeholder decision-making and policy by providing clear, usable information to stakeholders and the general public.
The role of conceptual frameworks in defining and assessing sustainability
Sustainability is a nebulous word, and its definition and usage depend on both scale and context (Barbier 1987; Morelli 2011; Thornton et al. 2018; Purvis et al. 2019; Ruggerio 2021). Conceptual frameworks are a tool that can be used to address this by providing an agreed-on perspective from which to define and assess sustainability in a specific set of circumstances (Lemke 2021). Frameworks can take the form of visual diagrams, ‘checklists’, or standards and practices guidebooks, etc. They serve the purpose of defining the specific perspectives from which an issue should be addressed, while also ensuring that all relevant aspects are considered in the assessment and decision-making process. This approach can then be used to explore complex scenarios and assess and compare the impacts of different interventions along different temporal and spatial scales, while making results easier to interpret (Weinberger et al. 2015; Thornton et al. 2018; Lemke 2021). For example, the Livestock Environmental Assessment and Performance Partnership (LEAP) guidelines of FAO (FAO 2016a, 2016b, 2016c) are a set of frameworks designed to provide best practices for assessment of the sustainability of different sectors of livestock production.
In the context of this review, sustainability as it applies to food systems can be thought of as creating a food system that feeds everyone, while simultaneously optimising resource use and minimising net negative impacts across multiple domains. In 1992, the United Nations conference on sustainable development defined a framework for assessing sustainability across three different domains (UNCED 1992, as cited by Lemke 2021). This definition of sustainability can be considered a framework in and of itself, and is known as a ‘triple bottom-line’ assessment (Hacking and Guthrie 2008). The three domains of sustainability contained in this triple bottom-line framework are as follows:
Environmental sustainability. This domain includes, but is not limited to, the reduction of pollution and waste, the conservation of the natural environment, and preserving water and land resources for future generations. Diversity of crops and breeds and genetic conservation also falls under this domain.
Economic sustainability. This domain includes ensuring that food is affordable to produce, that prices and costs are such that farmers can afford to produce the food, and making sure that all segments of society have access to affordable, healthy food. Economic sustainability must also be such that that built food systems are resilient to market shocks and/or the impacts of adverse climate events.
Social sustainability. This domain includes issues such as ensuring that the food produced meets the specific nutritional requirements and health needs of all populations and is explicitly inclusive of marginalised groups such as people with disabilities, lower socioeconomic-status groups, and marginalised ethnicities and genders. Increasing equity and participation/representation of all levels and groups in society in the food system is also key to this domain. Social sustainability must also specifically account for differences in cultural values and preferences surrounding food, as well as differences in individual opinions.
In addition to these domains, Lemke (2021) builds on the work of Witjes et al. (2017) and discusses the importance of geographical scale (local, national, regional, global) and temporal scale (now vs the future) in sustainable development. The temporal and regional scales of assessment are already incorporated into many existing sustainability frameworks, although specific methods vary widely among frameworks. Net impact assessment, as discussed by Harrison et al. (2021), inherently contains this temporal scale, because net impact, as used in sustainability, is defined as assessing the impact of future changes compared with a set baseline, i.e. the net difference between scenarios.
The framework described by Lemke (2021) was designed primarily for use in sustainable development and is not comprehensive. This review proposes that sustainability assessments of food production adapt the triple bottom-line conceptual framework to factor in the following key biophysical impact scales of food production and changes to food production systems:
Animal scale. This scale includes impacts at the individual-animal level, such as the effect of changing feeds or management on feed intake, metabolism, efficiency, animal-level emissions, and product quality.
Human food-supply scale. This scale specifically considers the quantity and quality of nutrients supplied post-farm to the consumer, ensuring that the socio-cultural and nutritional needs of all populations are met, with realistic assumptions about human diets and essential/discretionary food intake.
Food production-system scale. This scale has a cradle-to-consumer boundary, and considers environmental footprints, resource inputs, land/water/resource use, nutrient flows in/out, nutrients supplied to human populations, ecosystem services, including retail-level impacts and waste.
These scales are not linear, and (a) and (b) are nested in (c), each of enormous scale and diversity. For example, the combination of sustainability domain (environmental, economic, social) and biophysical scale (animal, human food supply, food production) could be expressed as a 3 × 3 factorial. This then builds a framework that can be used as a checklist for exploring the results of those changes. Such a framework serves as a mental model for comparing existing frameworks and models, and for discussing system challenges that can be addressed to improve the sustainability of food production systems, particularly livestock. This framework also serves as the foundation for the proposed livestock sustainability modelling framework described later in this review.
Existing sustainability frameworks, models, and limitations: a triple bottom-line perspective
Even within the context of the triple bottom-line sustainability framework, considerable variation exists in how to apply it in the context of food production and human diets. Few models or frameworks examine all three domains simultaneously, and existing studies vary widely in what methods and metrics are used to assess sustainability and performance (de Olde et al. 2018; McClelland et al. 2018; Lemke 2021). The choice of geographical and temporal scale at which decisions are assessed is critical to designing an assessment and interpreting the results (Witjes et al. 2017; Lemke 2021), but the selection of what specific metrics are used to define sustainability and how they are combined or weighted is even more crucial (de Olde et al. 2018; McClelland et al. 2018; Harrison et al. 2021).
Methods used for assessing sustainability in livestock systems and in human diets overlap substantially, varying primarily due to differences in system boundaries (cradle-to-farm-gate, cradle-to-retail, etc.) and functional units. The functional unit is the denominator which impacts are expressed in relation to, such as kg CO2 per kilogram of product or litres of water per gram of protein. In livestock production systems, the functional unit may be per kilogram of live animal weight, kilogram of carcass weight, kilogram of meat/eggs at retail, etc. (FAO 2016a), while in diet-level assessments, the functional unit may be a specific nutrient such as calcium or protein, or a multi-nutrient profile score (Ridoutt 2021a). Choice of functional unit is key to clear, transparent assessments and comparisons, and is a way of relating sustainability metrics to the functions of the products produced by the system under study (ISO 2006a, 2006b; FAO 2016a, 2016b, 2016c). Comparing results of studies with different functional units is difficult and can lead to biased interpretations if not undertaken with extreme caution. Because of this, the choice of functional unit can have dramatic effects on both the relative sustainability and relative value/ranking of products (van Zanten et al. 2018a; Ridoutt 2021a).
The sections below provide an overview of current best practices and frameworks for modelling sustainability in livestock production systems and human diets from the perspective of the 3 × 3 sustainability domain × biophysical scale framework discussed above. A comprehensive review of existing models and frameworks for assessing sustainability would require a body of several reviews in and of themselves, such as the review of small ruminant models by Dougherty et al. (2019a); however, selected key models and frameworks will be used as examples throughout this paper.
Modelling economic sustainability
Economic assessment of sustainability is the process of applying accounting frameworks to biophysical systems. This domain considers financial metrics such as profitability, efficiency, productivity, as well as diversification, risk, and durability (Lebacq et al. 2013). Many farm/system financial calculators exist, as do input–output models, although they vary in scope and metrics used. An example of this is the Model of an Integrated Dryland Agricultural System (MIDAS) model (Kingwell and Pannell 1987; Young et al. 2014), a whole-farm model used for modelling of economic sustainability in Australian farms, including the impacts of climate change on whole-farm performance (Thamo et al. 2017). The Australian farm-optimisation model (Young and Young 2022; Young 2023) builds on the MIDAS model by including environmental impacts from on-farm activities, allowing for economic and environmental sustainability assessment. Some other economic models also consider environmental sustainability, but at a more limited scope than economic sustainability, such as in the BIO model (Grealis and O’Donoghue 2015; Tsakiridis et al. 2020). The BIO model uses an input–output approach for assessment of economic and environmental impacts, but uses carbon footprint as its sole indicator of environmental sustainability, and does not analyse the impact of aquaculture or wild-caught fish on biodiversity or other marine ecosystem indicators. One key limitation of this approach is that economic input–output approaches work for sectors that produce homogenous goods, but are not appropriate for comparing different products within the same sector (Tsakiridis et al. 2020), such as differences in product quality among breeds of livestock.
Modelling environmental sustainability
Assessing environmental sustainability can be thought of as applying accounting methods to environmental impacts instead of financial impacts. Input–output analysis has been used to compare and analysis systems with regard to metrics such as greenhouse-gas (GHG) emissions (Hendrie et al. 2016) and water scarcity (Ridoutt et al. 2018). To be successful, this requires that input–output data are available at the desired level and resolution. Life-cycle assessment (LCA) is a common approach that examines the environmental impacts of a product by mapping out its life cycle (creation, processing, use, disposal, recycling) and tracing the flows of materials in and out of the product system, as well as the environmental impacts associated with these flows and stages (de Vries and de Boer 2010). Life-cycle assessments are conducted according to a set of international standards (ISO 2006a, 2006b, 2018a). Building on the ISO framework, the LEAP framework of FAO is a set of best practices for LCA of livestock production (FAO 2016a, 2016b, 2016c).
Life-cycle assessment can be combined with economic frameworks to create combined economic and environmental input–output LCA, or can be process-based, using life-cycle inventory data and biophysical modelling to represent all the flows involved in the system under study (Tsakiridis et al. 2020). Many LCA studies use a software designed for such a purpose, such as SimaPro (PRé Sustainability 2023), OpenLCA (GreenDelta 2023), and GaBi (Sphera 2023). Some industries have their own industry-specific software, such as the Pig Production Environmental Footprint Calculator (National Pork Board 2017) or the Vit’LCA program for viticulture (Renouf and Renaud-Gentié 2023). However, other assessments use existing biophysical models that simulate the metabolism and production of the species under consideration. The environmental impacts can then be calculated from within the biophysical model itself, or the outputs of the biophysical model can then be combined with those obtained using other software. One advantage of this approach is the flexibility it provides, allowing for clear consideration of the animal-level impacts of a wide range of management strategies as long as sufficient data are available (Rotz 2018; Dougherty et al. 2019a, 2019b; Van Amburgh et al. 2019; Thomas et al. 2021).
Sustainability assessment should begin with clearly defining the question being explored, and choosing metrics that directly address this question (McClelland et al. 2018). Metrics must also be identified and compared at the right level of aggregation. For example, GHG emissions have global impacts. However, impacts such as eutrophication potential, biodiversity impacts, and land/water scarcity have impacts at a more regional scale and have inherently regional boundaries (Picasso et al. 2014; Ridoutt et al. 2018; Lemke 2021). Carbon footprint, one of the most popular metrics, relates the environmental impacts of GHG relative to that of carbon dioxide, allowing for reporting as a single metric (Forster et al. 2021). This metric has become a default metric of reporting environmental impacts and sustainability, although such a myopic focus on carbon at the expense of examining other metrics has the potential to lead to reduction in GHG at the expense of other sustainability dimensions such as water use, nutrient cycling, and land use (Harrison et al. 2021).
Metrics such as resource use must be also reported in the context of local scarcity and/or sufficiency. ISO (2014) standards mandate that water footprints cannot solely consider total water usage; they must also contain region-specific assessments of the impacts of water use in terms of specific metrics such as water scarcity and/or eutrophication potential. Such weighting is vital to both the interpretation of results as well as the implementation of water-saving strategies. For example, the use of irrigation for crops will have different impacts depending on the water scarcity of the region they are produced in, leading to differences in recommended cropping strategies and mitigation methods (Ridoutt et al. 2018). Similar frameworks apply to the weighting and reporting of land usage, weighting by its contribution to relative cropland scarcity and the environmental impacts of other land uses.
Many of these principles also apply to modelling at the dietary/human food-supply level. Multiple different indices have been developed to rank the nutrient quality of foods and diets, and combining nutritional quality with LCA, i.e. ‘n-LCA’ (Green et al. 2020, 2021), requires clear definition of both the functional unit of the assessment and the criteria on which decisions are being made, particularly when GHG emissions are expressed relative to units of a specific nutrient. Because the objective of many health eating/dietary health-scoring systems (Trichopoulou et al. 2003; Shams-White et al. 2023) is primarily to identify patterns of dietary intake associated with mortality risk, chronic disease prevention or chronic disease outcome management, rather than nutrient adequacy or direct measures of sustainability, their scope of healthy diet patterns still may not accommodate other dimensions of health and sustainability, depending on personal/cultural requirements or food accessibility. For example, the planetary health-diet index uses GHG emissions as its sole metric of sustainability (Cacau et al. 2021), while the sustainable-healthy-diet index utilises a questionnaire to measure sustainable dietary consumption, including questions about use of local food sources, reducing food waste, bottled water use, recycling, and composting, with the goal of capturing sociocultural aspects of sustainability (Tepper et al. 2021). Additionally, few nutritional indices or studies examine differences in nutrient bioavailability among foods, which also affects results (Ridoutt 2021b). Similarly, Cifelli et al. (2022) examined the impacts of replacing nutrients in dairy with other foods and found that it was possible to do so while optimising for either cost, energy intake, or quantity of food required, but that improvements in one metric led to significant increases in the other metrics. Recommendations from nutritional LCAs are dependent on the choice of a functional unit and a nutritional scoring system (Ridoutt 2021a), and assessing the sustainability of foods without considering their nutritional quality and contribution to meeting human requirements will inherently bias results.
Modelling social sustainability
There are fewer frameworks for assessing social sustainability than economic or environmental sustainability (Lemke 2021). The United Nations (UNEP/SETAC 2009, 2020) developed a framework for applying LCA to social sustainability that is compliant with ISO standards. This framework currently serves as the primary best-practice framework for modelling and assessing social sustainability across multiple sectors. However, application of this framework to food production is still an emerging area; a recent review of social sustainability assessment in the agrifood sector (Tragnone et al. 2022) found that social LCA was hindered by a lack of clarity about boundaries and functional units, poor data availability, a lack of transparent methodological choices, and poor inclusion of stakeholders in definition of the social themes being assessed. Similarly, de Olde et al. (2018) reviewed trade-offs between comprehensiveness and implementation in social sustainability-assessment frameworks and tools, and recommended harmonising metrics as much as possible with current best practices, while still ensuring that they are flexible enough to capture differences among systems and provide actionable information. An example of this is the work of Rivera-Huerta et al. (2019), which synthesised indicators from multiple sources to develop a comprehensive set of metrics that were then used to assess the social sustainability of livestock production in Mexico. Aranda et al. (2021) applied a similar holistic social sustainability-assessment framework to pork production in Spain. These studies are examples of adapting the more general UNEP/SETAC framework to the particular needs and concerns of the livestock sector, and the methods of these studies could be used to further inform and develop social sustainability frameworks specifically for the livestock sector.
System challenges in holistic sustainability assessment
Despite the many frameworks and models discussed above, there are still challenges in the holistic assessment of food production, as well as in the interpretation and application of these results. This section describes some key system challenges in sustainability assessment and discusses how they could be addressed within a holistic framework to provide more comprehensive assessments of both sustainable livestock production and sustainable human diets.
Increasing the prevalence of multi-metric, multi-scale assessments
Despite the recognised need for multi-metric, multi-scale assessments, many studies consider a limited number of sustainability metrics, primarily environmental (de Vries and de Boer 2010; Gerber et al. 2014; Picasso et al. 2014; Ridoutt et al. 2017; McClelland et al. 2018; Harrison et al. 2021). A review by McClelland et al. (2018) found that 98% of LCA studies of livestock production included climate change as an impact category, and 28% of studies looked only at that category. Such a narrow focus is a key limitation in how sustainability is assessed and reported.
In contrast, multi-metric studies are vital for helping ensure that progress in one area does not come at the cost of worse performance in another, and correlations among different metrics vary depending on the scale being assessed. For example, there is a poor correlation between carbon and water footprints when comparing different systems producing the same kind of livestock (Dougherty et al. 2019b; Klopatek et al. 2022). However, intensification of livestock production by moving away from grazing-based systems lowers carbon footprint at the cost of higher fuel and energy use, greater cropland and mining land use, and increased risks of eutrophication owing to fertiliser use (Picasso et al. 2014; Dougherty et al. 2019b; Klopatek et al. 2022). When different foods are compared, Ridoutt and Navarro Garcia (2020) found that plant-based foods such as cocoa, coffee, tea, nuts, and oilseeds contributed more to cropland scarcity than did ruminant products, particularly when assessed in terms of biomass productivity, malnutrition risk, and biodiversity. Similarly, Ridoutt et al. (2018, 2019, 2021a) found that optimising diet patterns to reduce climate impact led to an increase in water-scarcity impacts, and that plant-based foods had a higher water-scarcity footprint than did livestock products, either when assessed on a commodity or whole-diet basis. Due to correlations between metrics and the complexity of production systems, it may be impossible in some circumstances to find a solution that is optimal across all metrics and scales. Therefore, multi-metric studies are vital to ensure that progress in one area does not come with unexpected consequences down the road.
Incorporating planetary health perspectives into models of livestock systems
Sustainable food production and systems require a shift in thought from ‘what is sustainable for humans?’ to ‘what is sustainable for the planet?’ One example of this is the provision of ecosystem services in grasslands. Rangelands in areas such as North America, Central Asia, and Africa evolved under grazing pressure from large herbivores, and such gazing pressure has been a key ecological feature of these areas (Price et al. 2022; Thompson et al. 2023). The specific effects of grazing on biodiversity and ecosystem health are highly variable by site, and depend on the ecosystem’s evolutionary history with grazing pressure (Price et al. 2022), but where appropriate, carefully managed grazing can be used to provide a range of ecosystem services, such as the following:
Improving soil C sequestration (Kristensen et al. 2022; Thompson et al. 2023)
Controlling invasive plant species (Maron and Lill 2005; Marty 2005; Demeter et al. 2021)
Improving plant survival and species diversity by disturbing vegetation canopies and improving light penetration (Demeter et al. 2021; Thompson et al. 2023)
Reducing bushfire risk by consuming forage mass that would otherwise serve as fuel for fires (Huntsinger and Barry 2021; Kristensen et al. 2022)
Two-thirds of the world’s agricultural land is either permanent pastures, meadows, or land that is too marginal to grow row crops on because of limitations of climate, terrain, soil characteristics, or other factors (Mottet et al. 2017; FAO 2020a). While some of this land has historically been converted to cropland, it comes with a reduction in soil quality and carbon sequestration potential (Zhang et al. 2021) and such conversion is a leading cause of habitat and biodiversity loss (McClelland et al. 2018; Lark et al. 2020). The IPCC (2022) noted that preventing degradation of existing rangelands and restoring previously converted land are key pathways to reducing emissions and sequestering carbon, and that animal management is a key tool towards this goal.
While the incorporation of ecosystem services into sustainability assessment frameworks is an emerging area, there are existing methods for modelling soil organic carbon and biodiversity that could be incorporated into this framework, as reviewed by Thomas et al. (2023). Fewer frameworks are currently available to address the issue of avoided emissions, largely due to a lack of methods to quantify the emissions reductions from novel management practices. The use of such frameworks and/or models would be dependent on sufficient data to robustly parameterise, independently validate, and implement these methods. However, among-site and within-site variation may be high enough that such methods may be feasible only for more generalised or regional assessments rather than site-specific analyses. Further exploration of data and methods available could be used to determine which of the above frameworks would be most feasible to implement and/or most useful to stakeholders and would aid in the inclusion of ecosystem services and their planet-level/ecosystem-level benefits into holistic modelling of livestock production.
Representing economic and social multifunctionality in livestock production systems
Livestock play multiple roles in human society and can contribute positively to economic and social sustainability. In addition to being a ‘battery of nutrients’ (Wyngaarden et al. 2020), livestock can serve as a ‘living bank’, being both a source of income but also a form of risk management against future economic insecurity, particularly in low- or middle-income countries (LMIC) and in lower-income households (Herrero et al. 2013; Alders et al. 2021a, 2021b). Livestock also have a strong synergy with cropping systems; manure accounts for half of world nitrogen fertiliser use and one-third of nitrogen fertiliser applied to crops (Gao and Cabrera Serrenho 2023). This latter point is especially the key, given that ammonia production for use as synthetic N fertiliser uses 1–2% of the world’s total energy supply, primarily from fossil fuels, and 3–5% of the world’s annual natural gas production, in addition to other environmental effects (Ghavam et al. 2021).
Livestock have historically been a key source of draught labour, a role that continues to this day in LMIC and rural areas worldwide; although understudied, this role is key to successful farming in many areas, and, depending on the region the value of draught services and manure, may exceed that of milk and meat, although the value of draught power is not often incorporated in sustainability assessments (Mota-Rojas et al. 2021). The rearing and sale of livestock and their products are a key pathway to food and financial security for women, (Herrero et al. 2013; Alders et al. 2021b), and greater support for women livestock producers, either individually or through the formation and promotion of women-led agricultural cooperatives (Oliver 2016; Lecoutere 2017), has been identified as a key pathway to resilience and sustainability by groups such as the FAO (2020b) and ILRI (2023). Incorporating these aspects into a social sustainability-assessment framework would help better reflect the varied reasons people keep livestock, and their important role in economic and social response to changes and longer-term sustainability.
Better incorporation of role of consumer choice and human diversity in sustainable diets
Discussion around sustainable diets, whether in scientific literature or the broader media, has been primarily framed in terms of environmental impacts. However, this is too narrow a perspective; diets must be evaluated from a triple bottom-line framework, while also accounting for variation in human needs and consumer choice. Many nutritional studies define optimal diets primarily in terms of meeting specific nutrient requirements, omitting the effects of regional and cultural differences in what foods are consumed (Steenson and Buttriss 2020; Ridoutt 2021a, 2021b; Biesbroek et al. 2023). Similarly, medical conditions such as food allergies, chronic kidney disease, or celiac disease require specialised diets, leading to differences in recommended nutrient intakes, higher than average food costs (Leiman et al. 2022; Scurlock et al. 2022), and therefore different optimal recommendations when evaluated from a triple bottom-line perspective. The lack of regional variation in dietary sustainability studies is especially concerning for LMIC (Reider et al. 2020; Tam et al. 2020), which have a high prevalence of nutrient-poor diets, affecting growth and development, and increasing risk of malnutrition and iron-deficiency anaemia (Keats et al. 2018; Khan et al. 2022). Therefore, certain areas, such as many LMIC, may benefit from higher proportions of animal foods, as rich sources of vitamin B12, iron, calcium, and zinc, to help combat prevalent nutrient deficiencies (Beal et al. 2023), contrary to current widespread recommendations of reducing meat and other animal-product consumption. Analyses must also consider regional variation in prices and availability of different foods, as well as substitution of one food for another on the basis of differences in cost and/or consumer income. All of these issues must be factored into assessments of sustainability at the dietary level, or risk excluding large sectors of the population; however, literature around sustainable diets does not often adequately consider these factors, and they have yet to be incorporated into many modelling frameworks.
Proposed dietary interventions must also be realistic in terms of reflecting human behaviour and consumption patterns. For example, US dietary guidelines recommend that ≤15% of calories come from discretionary foods such as sweetened beverages, candies, and alcohol; however, actual intake is often higher. Although reducing discretionary intake could significantly reduce environmental impacts, consumption trends suggest limited reduction feasibility and therefore such discretionary intake must be factored into modelled diets if they are to be realistic representations of how people eat (Hendrie et al. 2016; US DHHS and USDA 2020; Ridoutt et al. 2021b). Similarly, when people eliminate one type of food from their diet, they may not adequately replace lost nutrient sources. For example, individuals that exclude dairy products from their diet, whether due to personal preference or medical necessity, may replace dairy intake with dairy alternatives that vary in their ability to replace the nutrients previously present in dairy, particularly vitamin D and calcium (Ridoutt et al. 2020; Clegg et al. 2021). This is of particular concern for human health, as people in many countries already struggle to meet calcium and vitamin D intake recommendations, increasing the risk of negative health outcomes (Shlisky et al. 2022; Cui et al. 2023).
Multi-metric scoring systems have great potential to help explore the trade-offs among different diets from a net impact perspective, as long as the diets modelled are realistic in terms of variation in human choices and resources available. For example, Ridoutt et al. (2021a) found that optimising diet patterns to reduce climate impact led to an increase in water-scarcity impacts. Similarly, Cifelli et al. (2022) examined the impacts of replacing nutrients in dairy with other foods and found that it was possible to do so while optimising for either cost, energy intake, or quantity of food required, but that improvements in one metric led to significant increases in the other metrics. Recommendations from nutritional LCAs are dependent on the choice of functional unit and nutritional scoring system (Ridoutt 2021a) and assessing the sustainability of foods without considering their nutritional quality and contribution to meeting human requirements will inherently bias results.
Worldwide, animal-sourced foods provide 39% of per capita protein intake (FAOSTAT 2023) and are a key source of bioavailable nutrients such as iron, vitamin D, folic acid, and essential fatty acids (White and Hall 2017; Adesogan et al. 2020). As such, animal-sourced foods can be a key source of nutrients to prevent deficiencies in at-risk populations. It is therefore important to consider the role and efficacy of animal-derived nutrients in ensuring accessibility to nutrient-dense foods, especially where micronutrient supplementation and fortification programs continue to face barriers of access or coverage to healthcare services, adequate education, cultural acceptance and community engagement, and program sustainability (Zavaleta 2017; Stelle et al. 2019), and draws attention to the risk of ‘one size fits all’ dietary patterns exacerbating existing marginalisation, an issue that must be more addressed by sustainability science going forward. Incorporating regional and cultural variation in dietary patterns and substitution would enhance the social sustainability domain of a triple bottom-line assessment, and prevent the ‘one size fits all’ myopia seen elsewhere in the literature. This could then be a part of a multi-metric assessment framework that considered the impacts across nutritional, economic, and environmental scales.
The need for representation of the impacts of livestock consumption of crop by-products and food waste on triple bottom-line sustainability and the impact on human food supply
Livestock also play a vital role in upcycling human-inedible crop by-products into food, fuel, and fibre, adding nutrients contained in these products back into the human food chain and reducing waste. However, the net impacts of such circularity among different sectors of food production are not always captured in biophysical models and sustainability frameworks. Modelling these effects at the food production-system scale is key to exploration and assessment of the broader impacts of food production. The majority of biomass produced from crop production is not human-edible; every kilogram of common human-edible crops grow yields of roughly 3 kg of human-inedible biomass in the form of crop residues and process by-products such as canola meal, beet pulp, and straw. These by-products account for one-third of the mass of feed fed to livestock worldwide (Mottet et al. 2017, 2018; Thompson et al. 2023). At the farm scale, nutrients remain trapped in post-harvest residues such as corn stover and wheat stubble, hindering their use as soil amendments, although the stubble can still serve as an important nutrient source to later crops (Stavi et al. 2016; Li et al. 2021; Thompson et al. 2023). This residue can also be grazed by livestock, returning nutrients to the soil in a more bioavailable from as manure, increasing later crop yields (Hunt et al. 2016; Stavi et al. 2016; Li et al. 2021), and reducing net farm-level losses of carbon, nitrogen, sulfur and potassium from the farm when compared with removal of stubble via burning (Agriculture Victoria 2023).
Worldwide, 10–13% of feed fed to livestock across all species comes from the human-inedible by-products of crops grown primarily for human consumption, such as oilseed cake, beet pulp, spent grains, and distiller’s grains from ethanol production (Mottet et al. 2017, 2018; Sandström et al. 2022). However, the environmental and economic importance of this often goes unreported in sustainability assessments; most such assessments are cradle-to-farm gate, excluding further crop-processing stages and their associated by-products and co-products. One key example of this is dried distiller’s grains with solubles (DDGs), a by-product of ethanol production. Increasing world production of ethanol for biofuel has led to concurrent increases in the supply of DDGs, which could be used to replace almost half of cereals in swine and poultry diets, or even higher levels in cattle diets, with largely neutral-to-positive impacts on productivity depending on the inclusion level (Buenavista et al. 2021; Sandström et al. 2022). This improves whole-of-system efficiency and reduces feed–food competition (van Hal et al. 2019; van Zanten et al. 2019).
Similarly, growing per capita food consumption and the association of higher-quality diets with higher amounts of food waste mean that reducing food waste is a key sustainability issue (Australian DCCEEW 2018; Conrad et al. 2018; FIAL 2021), one which careful feeding of food waste to livestock could be used to address within a multi-scale sustainability framework. Food waste can be safely used in diets for livestock such as cattle, swine, and prawns, replacing human-edible ingredients without sacrificing performance (Pinotti et al. 2021; Truong et al. 2021). One key example of this is the EcoFeed program in Japan and South Korea; this process uses a combination of heat treatment and ensiling to turn food waste into pelleted stockfeed, decreasing food waste and feed–food competition, reducing biosecurity risks from the use of food waste as stockfeed and redirecting grain from livestock fodder to direct human consumption (Sugiura et al. 2009; zu Ermgassen et al. 2016; Salemdeeb et al. 2017).
The use of these by-products and waste represents a net upgrading of nutrients into the human food chain, as livestock take human-inedible nutrients and ‘upcycle’ them into highly digestible, high-quality food while reducing total resources required for food production, and increasing the net nutrient contribution to the human food supply. Mottet et al. (2017, 2018) reported that ruminants produce 67% more human-edible protein than they consume, with similar or even higher numbers when protein quality (digestibility and amino acid balance) is considered (Ertl et al. 2015; Baber et al. 2018; Thomas et al. 2021; van Barneveld et al. 2023). Because these effects span multiple biophysical scales, the true impacts of such changes are best realised within a multi-scale, multi-metric modelling framework. Such a framework could then be used to further explore livestock’s role as ‘upcyclers’, balancing effects on animal-scale productivity with system-scale impacts on nutrient capture, food loss, and waste reduction.
A proposed conceptual biophysical modelling framework for holistic sustainability assessment
Models are both analytical tools and frameworks in and of themselves; the conceptual framework underpins how the model approaches the issue and what scales and domains are assessed. Within this context, the conceptual domain × biophysical scale framework discussed above can be used as a pathway for building a holistic biophysical modelling framework that could be used to address system challenges in sustainability assessment. An example of how this could be implemented is shown in Fig. 1.
Proposed model structure incorporating biophysical modelling, multi-axis sustainability assessment, and decision/social modelling. Adapted in part from APSIM Initiative (2023) and used with author permission.
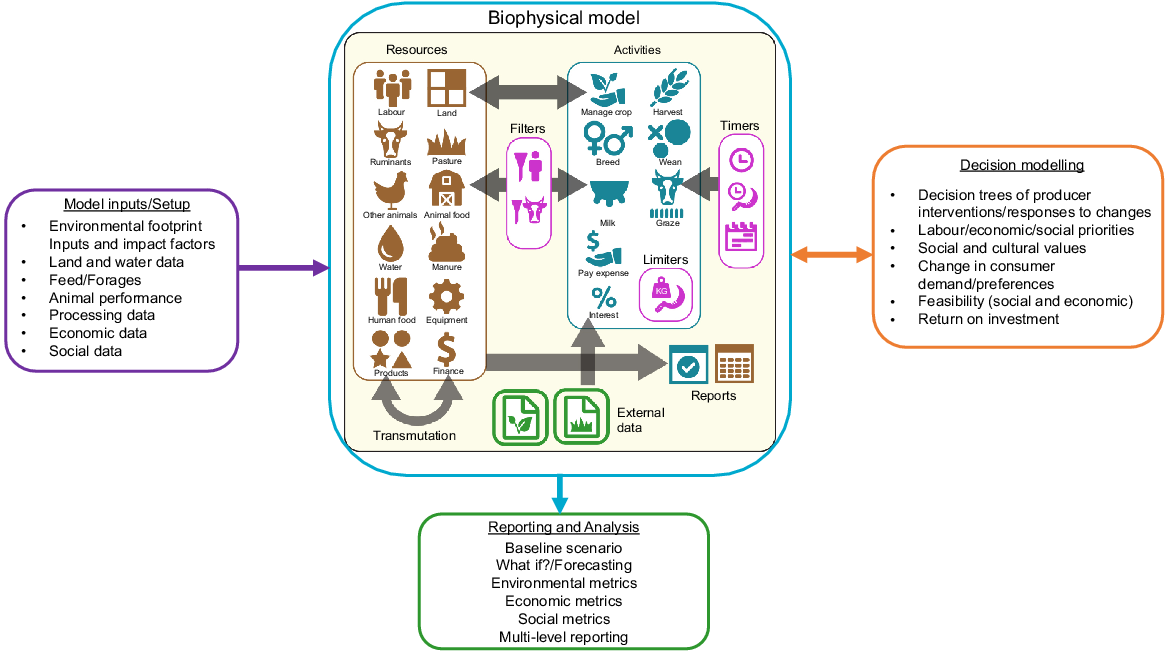
It is important to note that the framework discussed here is not meant to represent a specific existing or planned project, but rather to propose a conceptual modelling framework that provides guidance for what issues and scales need to be addressed, and gives examples of how modelling could be used to address current system challenges in sustainability assessment. Many of the aspects discussed in this proposed framework are already present to varying degrees in existing models. However, integration of these aspects into a cohesive framework or set of tools would make it easier to provide the kind of integrated biophysical scale × sustainability domain assessment that is key to improving our understanding of sustainable food systems. This framework should be developed such that it can be used to support producers and systems in improving their overall sustainability, and therefore should be flexible enough to represent a wide variety of circumstances. It is the hope of the authors that the framework pathway presented here will be considered by researchers and stakeholders as they seek to model existing systems and develop new models and/or sustainability assessment tools.
The proposed framework (Fig. 1) uses a triple bottom-line approach as a foundation to explore the impacts of changes at the animal scale and/or food production system scale on human food supply, and the feedback loops among the biophysical scales. This framework could be built by linking a biophysical farm system model to a decision model via feedback loops, for showing the iterative impacts of decision-making over time as the model runs. The modelling framework would take user inputs about the system under study and the environmental, economic, and social metrics of interest to deliver baseline and what-if analyses regarding a given production system. Model outputs could be customised by the user, but with the goal of delivering a holistic, multi-metric assessment of performance and sustainability
The modelling framework would consist of a biophysical model capable of representing the farm/production system under study from a holistic perspective, including but not limited to aspects such as
Livestock growth, metabolism, feed intake, and reproduction
Pasture/feed crop growth (including mixed farming systems and crop residue grazing)
Manure management (and use as fertiliser)
Methane and nitrous oxide emissions from livestock and manure
Variation in genetics and product quality
Inputs from side streams/by-products of other production systems across the food chain (as part of circularity)
The biophysical model could be initialised by allowing users to combine data from libraries inside the model to recreate the system under study, with the option for users to overwrite/edit data for custom scenarios as needed. A combination of user-provided data and data from internal and external libraries could be used as inputs to the model, such as:
Environmental impact factors/footprint data (calculated internally or from environmental impact databases like those used for LCA)
Land and water use data (from geospatial surveys or other data sources)
Feed data (on-farm feed production, off-farm feed purchases, feed quality)
Animal breed and performance data (age, weight, performance, mature size, breed, reproductive management)
Livestock and crop processing data (from literature or databases)
Economic data (historical costs and revenues, expected market trends, seasonal price variation)
Climate data (past or projected weather patterns)
Social data (from surveys or literature data)
A flexible output and reporting interface, such as is already present in some whole-farm models such as GrassGro (Freer et al. 2012), IFSM (Rotz et al. 2022), and CLEM (APSIM Initiative 2023), would allow users to select the biophysical outputs and sustainability metrics of interest such as average daily gain, cost per hectare, methane production intensity, water scarcity-weighted water footprint, or eutrophication potential. Users could also select the biophysical scale (animal level, herd level, farm level, food system level, etc.) and time scale (daily, monthly, yearly, etc.) of reporting. Once a baseline analysis has been established, the model could then be rerun to examine the effects of different changes at levels of interest, and outputs would be reported relative to the baseline.
The biophysical model should be flexible enough to represent a wide variety of production systems and management decisions, while remaining simple enough that users can readily parameterise it with the data available to them. Biophysical models are a key tool for assessing environmental and economic sustainability at the animal and farm-system scales (Dougherty et al. 2019a; Tedeschi 2023). Some existing whole-farm models designed to assess economic and environmental sustainability, such as GrassGro (Freer et al. 2012), APSIM (Holzworth et al. 2018), the Australian farm-optimisation model (Young and Young 2022; Young 2023), RuFaS (Hansen et al. 2021), the IFSM (Rotz et al. 2022), and CLEM (APSIM Initiative 2023), also incorporate some degree of decision-making modelling by allowing users to set rules for buying/selling/managing resources depending on the internal state of the model, or including social aspects such as labour constraints, or cultural and community values. These existing decision-making models could serve as examples of a starting point for developing the holistic modelling framework discussed here.
However, existing models do not go far enough in terms of fully capturing the feedback loops between the biophysical state of a production system and the decisions made on both the producer and consumer side. This could be expanded on in the modelling framework discussed here by more concretely building in iterative feedback loops between human actions (changes in management, prices, consumer demand, etc.) and the biophysical state of the system. Examples of this include the impact of changing cattle mature size or stocking rate on whole-farm productivity and environmental impacts, with feedback loops including dynamically optimising stocking rate on the basis of producer goals regarding pasture amount and ground cover, as well as producer risk profiles regarding drought risk and expected cattle prices.
A key strength of the proposed conceptual modelling framework would be its ability to apply a triple bottom-line perspective to examine the net impacts of proposed changes on production and sustainability across multiple biophysical scales. Such a framework would allow for examining the effects of management changes, changes in market prices/consumer demand, changes in biophysical conditions such as drought, or combinations of the above, and allow for robust assessment of the trade-offs of different management strategies or responses to external conditions.
An example of how this could be developed and used is the case of modelling net impacts of different management changes to a region’s dairy farms. A biophysical model could be used to simulate a regionally representative average dairy farm, by using a combination of publicly available data and information from local stakeholders. A baseline analysis would be conducted focusing on the impacts of ‘business as usual’ from a triple bottom-line perspective. Once the baseline was established, different ‘what if?’ analyses could be conducted, such as ‘what is the net impact of changing stocking rate and switching from wheat grain to canola meal in our supplement, particularly on carbon footprint and feed costs?’, or ‘what would be the net effects of switching from year-round to seasonal calving in terms of water usage, cost to produce, and labour requirements?’, looking at the impacts on the performance of the cows themselves but also that of the whole farm system and the quantity and quality of dairy supplied to the consumer from this farm system. Additional feedback loops between the biophysical model and social and economic factors such as consumer demand for dairy products and seasonal variation in prices could be added in by linking the biophysical model with a decision model, either an existing one or one adapted for this framework. Depending on resources and data available, methods for adding in more in-depth social sustainability assessment could also be added into the framework. Eventually, this analysis could be scaled up to represent dairy production across multiple regions and across the food supply chain, such as the impacts of farm management on the production of dairy products themselves as well as dairy beef, or other linkages between systems and regions.
The extent to which the above example could be realised is dependent on data availability and the specific questions users wish to answer, but it serves as an example of how the holistic conceptual modelling framework presented here can be thought of as a checklist, or even a ‘wishlist’ of how multi-metric sustainability assessments could be developed and implemented. While there are many different methods by which such a modelling framework could be realised, it is the hope of the authors that this framework could serve as a pathway for what should be considered for inclusion in new or updated sustainability assessment frameworks and models.
Data gaps and methodology roadblocks in realising the biophysical modelling framework
The framework discussed above represents a synthesis of multiple perspectives and approaches to holistically model and assess the sustainability of food production systems across multiple domains and biophysical scales. This section discusses key data and methodology gaps that should be addressed so as to fully realise the proposed framework, and discusses how these gaps could be resolved.
Filling data gaps by increasing collection of region-specific data and variation in livestock systems and food production systems
Scientists have a great opportunity to improve the applicability and utility of sustainability assessments by collecting additional data on regional variation in food production systems, specifically in understudied regions and types of production system. However, existing sustainability literature is imbalanced in terms of regions studied; Europe and North America combined contain only ~15% of the world’s population (United Nations 2022) and produce one-third of the world’s animal protein by mass (FAO GLEAM 2023), yet the majority of life-cycle inventory (LCI) data used in sustainability assessments come from these regions, as do the majority of agrifood sustainability studies (McClelland et al. 2018; Alhashim et al. 2021; United Nations 2023). This is an especially pressing issue for the assessment of sustainable livestock production, as world per capita demand for animal protein is expected to increase by 14% between 2020 and 2050, primarily in Africa and Asia, where demand is expected to increase 55% and 49% respectively (Komarek et al. 2021).
Data for many of these regions is sparse; what exists may not use common units or methods, or may be out of date, incomplete, or otherwise not usable in analyses. A report by Eady et al. (2014) identified ‘a deficiency in accurate and consistent local information’ as a key issue with conducting LCA in Australia and other poorly represented regions, noting that substantial differences in regions, yields, and production practices make the use of European/North American data outside their respective regions of origin a known source of error. There have been efforts to address this by creating region-specific databases in countries such as Australia (Eady et al. 2014), Japan (ILCAJ 2023), and Brazil (Shirosaki Marçal de Souza et al. 2021), but such efforts are constrained by data availability issues and a lack of local practitioners (Valdivia et al. 2017), as well as lack of funds for systemic data-collection and data-sharing initiatives. This is also a priority under the United Nations Sustainable Development Goals (United Nations 2023), which highlight the imbalances in data and resources that limit the progress of LMIC in developing region-specific solutions for sustainability.
As part of filling data gaps in underrepresented regions, particular care needs to be paid to representing a wide range of variation in production systems and their corresponding biophysical factors. Many LCI databases treat food products as more or less interchangeable bulk commodities, but in livestock systems the quality and source of a product can substantially effect environmental and economic impacts. Livestock breeds and management vary widely among and within regions, but this variation may be poorly represented in the literature or other data sources (Emerenciano et al. 2022). Just as North America and Europe are over-represented in current LCA data, so are their preferred breeds of livestock, while more tropically adapted breeds such as Nellore cattle are under-represented, further hindering sustainability efforts. Native breeds are also undervalued in terms of their role in sustainability, despite their adaptation to existing environments, particularly regarding heat tolerance and disease resistance (Mwai et al. 2015; Sejian et al. 2018; Son et al. 2019). Between-breed variation in resource needs and product quality can also substantially affect the environmental impacts of food production systems. For example, cheese produced from Jersey milk has lower environmental impacts and resource use than that produced from Holstein milk due to breed differences in milk composition and energy metabolism (Capper and Cady 2012; Kristensen et al. 2022), while Herron et al. (2021) found that genetic selection for maturity rate and carcass traits had significant effects on the environmental impacts of beef production, particularly when differences in carcass quality were included. This latter point is especially key, as such product differentiation (i.e. carcass quality and yield) also drives differences in producer payments and therefore economic sustainability.
Biophysical models can be used to represent among-breed differences in resource use, product quality, and environmental impact. Many models already incorporate bred-specific growth parameters for Bos indicus and Bos taurus cattle, such as GrassGro (Freer et al. 2012) and the NASEM beef model (NASEM 2016). Additional breeds can be represented, provided sufficient data are available for parameterisation. These differences should be included by using biophysical models to underpin LCA analyses, and among-breed variation in impacts should be included in LCA databases to reflect these differences and better capture the effects of different systems of livestock production. This would allow for broader representation of among-breed differences in product quality and environmental impacts, improving the specificity and applicability of analyses. To address these issues, regional data collection efforts should be prioritised for support to enable clear, representative assessment of regional variations in livestock production and sustainability, with a focus on historically under-represented areas. There is also a need for greater resourcing to develop and/or upgrade parameters.
Improve coordination of data collection and organisation to improve efficacy of data use across disciplines
Data can come from many sources such as research, industry, producer groups, government, all with their own goals, rules, and preferences regarding the collection and use of data. The framework proposed above requires the collection of additional data to ensure proper parameterisation, testing, and independent validation. It is true that advances in technology mean that more data are being collected than ever before, but actually accessing such data can be trickier; it may be siloed in proprietary software, vary in format, under existing intellectual property agreements, and vary in scope and scale (Osinga et al. 2022). Producers often have mixed feelings towards use of their data in ‘smart farming’ or broader data-collection initiatives, such as concerns about privacy, control over how the data will be used, data security. A survey of Australian farmer attitudes towards smart farming and data (Wiseman et al. 2019) found that the majority of respondents felt uncomfortable about service providers turning a profit from data collected on-farm, and trust in data security and control over data was low, similar to results found in other countries.
Therefore, this issue must be addressed from multiple perspectives, including the following:
Existing data must be better organised and centralised if possible so that it is findable and verifiable
Where possible, data collected as part of broader surveys and studies should follow area-specific best practices for data collection and format/methods, such as ISO guidelines, the LCI standards discussed in Eady et al. (2014), or others as appropriate
Data must be collected under a clear, transparent agreement where the confidentiality, security, and potential future uses of data are agreed to by the subjects and by those collecting and storing the data
Similar data collection and use agreements already exist in human-studies research conducted by governments and researchers, but private industry is not bound by such rules, contributing to the erosion of trust discussed above. Government agencies can play a key role in the collation and organisation of databases from multiple sources, but this requires specific support staff to ensure that data are managed appropriately and that data collected meets criteria for quality and format; such databases must be maintained and updated in the long term.
The need for clear, transparent standards and methods to define best practices for environmental sustainability assessment
Even within existing frameworks of best practices in sustainability assessment, such as the ISO and LEAP frameworks, there are still areas where frameworks require additional development. In particular, lack of consensus about allocation methods, system boundaries, and modelling of avoided impacts are key issues that should be addressed as part of developing clearer, more transparent assessment frameworks.
Uncertainty regarding modelling of avoided impacts is partly due to lack of consensus in the best methods for modelling impact allocation and system boundaries. Impact allocation refers to the divvying of impacts among multiple outputs of the same system, such as meat, dairy, and manure. Current best-practice frameworks such as ISO and LEAP (ISO 2006a, 2006b, 2014, 2018a, 2018b; FAO 2016a, 2016b, 2016c) state that allocation among livestock products should be based on biophysical relationships and/or properties where possible; otherwise, economic allocation can be used. However, allocation ratios vary substantially among study, species, and method; Wiedemann et al. (2015) found that the wool:meat allocation ratio in Australian sheep farming varied from 4:96 to 52:48, depending on the farm and the allocation method; similar variability has been found in other species (Chen et al. 2017; Dougherty et al. 2019b; Kyttä et al. 2022; Mazzetto et al. 2022).
System boundaries define the scope and scale of assessment. While it is impractical to model everything, many assessments of food production are cradle-to-farm-gate, omitting the processing and/or retail phases. Although data for these phases may be limited in many cases, not accounting for the resources and waste produced in the processing and delivery of food to the consumer will significantly affect allocation among products, and can lead to biased estimation of the impacts of a product or system (Kyttä et al. 2022; Sun and Ruiz-Carrascal 2023). For example, edible offal might account for only a small proportion of carcass weight, but it is rich in protein and micronutrients. Depending on the functional unit (i.e. weight, protein, contribution to human nutrient requirements), the choice of which products to model and the impacts and resources used in their processing can substantially change the perceived impacts and benefits of the entire system (Weidemann and Yan 2014; Wingett and Alders 2023).
A lack of consensus regarding allocation methods and optimal system boundaries has led to further uncertainty in how best to model the impacts of feeding crop residues and by-products to livestock. The most boundaries used in LCA of food production are cradle-to-farmgate. However, these boundaries do not include impacts from crop by-products or processing, leading to limited reporting and exploration of the impacts of these stages. This contributes to an inherent mismatch in boundaries between the product as it exits the farm and the product as it plays a role in human diets, as well as a lack of consistency in how to account for the wastes and co-products produced at different stages. In the LEAP framework, the distinction among by-product, residue, and waste can be either biophysical or based on revenue (FAO 2016a, 2016b, 2016c) as shown in Table 1.
Term | Definition | Allocation procedure | |
---|---|---|---|
Co-product | Product arising from the same production system | Included in allocation split along with other products of the system under study | |
Residue/residual | Material leaving the production system in the condition as created. Negligible economic value but has potential for further use | Is not allocated any impacts, except from that where its use occurs outside system boundaries | |
Waste | Substances or objects that the producer intends to or is required to dispose of | Emissions and resource use from its disposal are included in the inventory |
However, these definitions cause problems in practice, in that such methods are context-specific and sensitive to market fluctuations, or because changes in production methods or value-adding alter the possible uses and prices commanded by a product. At what point is the economic value no longer ‘negligible’? In the LEAP framework for large ruminants, if manure from a dairy farm is sold or otherwise considered a ‘valuable output of the farm’, biophysical allocation should be used on the basis of the proportion of feed energy used for milk, meat, and manure, with example allocation factors of 77.5%, 7.2%, and 15.3% respectively (FAO 2016a). However, if manure is classified as a residual, it receives no allocation of emissions. This is a methodological inconsistency in current best-practice frameworks that leads to variation in reported results, making it trickier to use these results can be used to inform decisions, and therefore is a key issue to address.
Such inconsistency is particularly key in the context of using crop wastes/by-products as livestock feed, and current best-practice frameworks regarding the use of food waste and crop by-products by livestock could be considered to be ‘billing the garbage truck for trash collection’. Crops such as almonds, sugarcane, grains, etc. are grown for the value of their primary products to the human food supply. Livestock consumption of the remnant biomass (almond hulls, bagasse, distiller’s grains, etc.) serves as active waste prevention by repurposing items rejected for human consumption. As an example, if a biscuit company disposes of burnt/rejected biscuits (a waste) by selling them for a nominal price to a local swine producer, the unsuitable biscuits become a residual. From an LCA perspective, the biscuits disappear from the LCA of the biscuit company and appear on that of the swine producer, with no emissions allocated to the biscuits except for those from their transport to the farm. Under such methods, the biscuit company is able to avoid emissions associated with waste disposal, the swine producer is able to get a ‘lower-emissions’ feed product, and the emissions associated with the avoided waste are not accounted for or credited to either side. However, if the rejected biscuits were to become more economically valuable over time, they would then instead incur a share of emissions during production and use, effectively ‘billing’ the end user for what had previously been a way of avoiding emissions associated with waste disposal.
The use of these products also has the potential to reduce ‘feed–food competition’ by reducing the human-edible fractions of livestock diets, while simultaneously repurposing human-inedible crop by-products (Van Zanten et al. 2019). For example, approximately 30% of human-edible crops fed to livestock are fed to poultry (Sandström et al. 2022). Approximately 70% of poultry diets are edible by humans, but replacing human-edible feed with human-inedible biomass such as oilseed meal, distiller’s grains, etc. may lead to a decline in performance, which would be economically infeasible despite its environmental benefits (Sandström et al. 2022). The environmental benefits of such replacements can be quite substantial; study van Hal et al. (2019) noted that factoring such reductions in feed–food competition into sustainability assessment reduced the carbon footprint of egg production by 57% and opportunity cost-weighted land use by 88%, without factoring in further avoided emissions or potential economic impacts.
Just as producers should be credited for improvement, it is equally important to give credit to those who are already doing the right thing, even if doing the right thing environmentally may be less economically feasible (Herrero et al. 2016; Harrison et al. 2021; Thomas et al. 2023). This is both an opportunity and a serious need that must be addressed for holistic assessment of the impact of food waste/crop by-products as food from a holistic perspective. Such benefits and tradeoffs would be explicitly considered under the holistic modelling framework presented in this review, but to properly accomplish this, there must be clearer frameworks in place for how to conduct these assessments. Once these clearer methods are in place, it would then be possible to develop and implement programs to make these reductions in feed–food competition more economically sustainable via the use of carbon credits, biodiversity credentialling programs, or other initiatives, which could then help stakeholders make better decisions.
Developing a long-term strategy for delivering impact with a conceptual biophysical modelling framework
There has been an increasing demand for an evidence-based approach to support sustainable decision-making both in the private (e.g. supply chains, processes and products) and public (e.g. policies and regulations) sectors. Sustainability criteria for goods and services are increasingly becoming part of trade and market access agreements between economies. An evidence-based framework approach is also key to limiting the proliferation of ‘greenwashing’ claims: for example, the European Union Parliament and Council have reached an agreement to proscribe terms that promote environmental claims, such as ‘climate neutral’, ‘eco or environmentally friendly’, or ‘biodegradable’ if there is no evidence provided to show ‘excellent environmental performance’ against these claims (European Parliament 2023). While LCA results have been used to inform product design, several barriers exist that hinder the use of LCA in informing policy decisions. A key barrier is that ‘Governments lack a framework for integrating LCA information into the decision-making process...with other factors, such as economics and social impacts’ (Seidel 2016). Other barriers to incorporating knowledge from sustainability assessments into policy include the higher time and expense associated with conducting comprehensive assessments, as well as the lack of data availability, applicability, and quality required to support these assessments (Seidel 2016).
To address these barriers and realise the framework proposed above, a long-term, concerted effort is needed, including public–private partnerships and consultation with communities and stakeholders. Government organisations have the internal capacity and framework to work trans-disciplinarily, combining expertise from a wide range of sectors such as agriculture, economics, and public health to synthesise information in such a way that it could be used to support the modelling efforts described above, as well as play a role in centralising data and ensuring data are collected and used ethically. This perspective should be tied in with long-term data collection and modelling efforts so that such research and exploration is sustained and impactful, rather than a series of shorter-term projects that may or may not deliver lasting results, and such long-term investment in programs benefiting the public good is entirely within the purview of government agencies. Such investment could also support government agencies; effective, evidence-based policy requires analysis and forecasting to ensure that policies have the desired effects and meet key goals, and increased transdisciplinary data collection and analysis has the potential to improve the efficacy of new or existing policy.
Government can play a key role as program operators and creators of policy/science frameworks in this space, ensuring that the standards are based on reliable, robust science, while working with industry and other stakeholders to develop benchmarks and ensure feasibility of measuring, reporting, and achieving the desired outcomes (FIAL 2021; Thomas et al. 2023). Such programs will help drive commitment to action from industry stakeholders, as consumer/market pressure to prove sustainability of their production systems will help promote adoption. This approach aligns with four key ‘triggers’ that can be leveraged to accelerate sustainable change for agrifood systems, namely, institutions and governance, consumer awareness, income and wealth distribution, and innovative technologies and approaches. The FAO State of Food and Agriculture 2022 (FAO 2020c) report expands on four types of policy approaches for sustainable, inclusive, and resilient agrifood systems, which rely on the triple-bottom line concept of sustainability to meet all criteria mentioned (Table 2). The FAO report outlines the importance of considering the wider range of policies that are often needed to support sustainability transitions outside of the specific agri-food context (e.g. enabling infrastructure, current and emerging policies and regulations that may be acting as barriers to change, and social determinants of sustainability) (FAO 2020a).
Key area and purpose | Description | |
---|---|---|
Agriculture-targeted policies, legislation, and investments | Policies directly targeting agrifood systems, such as agricultural research, knowledge and extension services, and specific financing of agricultural innovation or practice change. | |
Creating an enabling environment | Policies not specifically linked to agrifood systems but that support technology, etc. uptake. These types of policies address current or potential environmental or infrastructural issues (e.g. transport, connectivity, energy), as well as other policies that may constrain changes, such as financial or data management policies. | |
Coherence towards sustainability | Policies that focus on encouraging producers to adopt changes that align with broader sustainability transitions, e.g. increase system resilience, support environmental sustainability, and conserve natural resources. | |
Social justice and wellbeing | Policies that aim to support socially just transitions through ensuring all groups benefit from proposed changes (e.g. marginalised groups such as smallholder producers, women, youth, etc.) and that address potential negative impacts on livelihoods and income. |
Existing best practice frameworks regarding environmental labels and declarations (ISO 2018b) provide a valuable framework for the creation and development of sustainability credentialing schemes. A review by Thomas et al. (2023) highlighted several key features of credentialing programs, including flexibility (ensuring that farmers can adapt to changing circumstances and integrate new methods/technology), clear, verifiable, science-based measures and outcomes, and performance-based approaches that recognise and reward positive changes, and ensuring that recommended methods are scalable. The same review noted that feasibility of implementation is a key part of producer adoption of credentialling programs, as was the perceived value of obtaining certification and the overall value proposition of the program. Clear communication of this, as supported by examples from the modelling framework presented here and backed up by reliable methods and verification, will be key to long-term success of such methods. Similarly, it is important to ensure that credentialling methods are robust enough to avoid ‘perverse incentives’, i.e., gaming the system to meet criteria (Thomas et al. 2023), while multi-metric assessment of sustainability outcomes can help avoid circumstances where improvement in some metrics leads to detrimental effects in others (Locatelli et al. 2015). A scoping review of nearly 18 000 papers on incentives for sustainable agricultural practices found that specific programs linked to short-term economic benefits for farmers more likely tended to lead to the adoption of new practices on-the-ground, which had positive outcomes for environmental factors, as well as profitability and farm productivity (Piñeiro et al. 2020). Different types of policies will likely all play a role at multiple scales and timepoints of the transition, and to address the needs of different stakeholders, and will be critical for identifying how science, technology and innovation can support these evolving policy needs.
From an Australian context, new policies have recently been adopted and frameworks are being developed to align national directions with international sustainability agreements and guidelines. These include targets to achieve net zero carbon dioxide emissions by 2050 (Climate Change Act 2022; Australian Federal Register of Legislation 2022), reduce waste and achieve a national circular economy by 2030 (National Waste Policy 2018 and Action Plan 2019; Australian DCCEEW 2018, 2019), while supporting the Australian agriculture industry to reach its goal of A$100 billion in production value by 2030 (Ag2030 Plan; Australian DAFF 2022). Similar frameworks and strategies are being developed internationally, such as the EU Farm to Fork Strategy (Molitorisová and Burke 2023), which covers the sustainability of both food production and consumption; Japan’s Green Food System Strategy (Japan MAFF 2021), and Canada’s Sustainable Agriculture Strategy (Agriculture and Agri-Food Canada 2023).
While high-level policies are important drivers of system transitions, it is important to review the barriers to system change, and how science and technology could have different types of policies to address them. Three key barriers that could be addressed by the holistic conceptual modelling framework discussed above are (a) incomplete costing of environmental and social externalities from food production, (b) variation in stakeholder willingness to change practices or adopt new methods, and (c) consumer and trade partner attitudes to and trust in ‘sustainability’ of value chains and products. Incomplete costing of externalities refers to the fact that impacts occur at different scales, not all of which are equally visible to stakeholders along the supply chain; climate change and carbon footprint are global issues, but resource depletion and soil erosion are more local issues that stakeholders further down the value chain may be unaware of, or not factor into their assessments of sustainability as much as issues that directly affect them (Picasso et al. 2014; von Braun and Hendriks 2023). This can be addressed via the use of holistic, multi-scale, multi-metric modelling frameworks such as that discussed above that explicitly address the environmental, social and economic components associated with value chains and systems as well as economic barriers to implementation and ‘off-shoring’ of environmental costs not reflected in market pricing. Increasing the likelihood of changing existing business practices can be addressed by using modelling frameworks to create data-driven business cases and locally relevant case studies, showing the clear value propositions of such changes, as well as the wider opportunities and risks of both adoption or maintaining current practices. Perceived benefits for the farm, the environment, or both, were the strongest motivators for practice adoption, although technical assistance and extension activities are also key drivers of producer uptake (Setsoafia et al. 2022). Finally, improved multi-metric sustainability frameworks can be used to develop data-driven sustainability credentials, and ensure traceability, transparency, and validity of value-chain claims while maintaining social licence to operate.
Conclusions
This review has discussed existing gaps and imbalances in modelling of sustainable livestock production and assessment of sustainable diets from a multi-domain (economic, environmental, social) and multi-scale (animal, human food supply, and food production system) sustainability perspective. A holistic modelling framework was proposed as a pathway to help guide future researchers in building tools to address these gaps. This framework proposes combining a biophysical model with expanded decision modelling and feedback loops to explore the iterative effects of producer decisions on animal-level and farm-level outputs, as well as how those outputs in turn affect future producer decisions. Realistic modelling of variation in livestock production systems, producer decision-making, and human diets, will in turn help target solutions to the specific needs of stakeholders and improve the likelihood of adoption of sustainable mitigation solutions. Within such a framework, modelling can then be used to examine impacts across multiple time horizons, and to explore the impacts of genetic selection of crops and livestock on the human food supply and its environmental impacts, or how different climate scenarios will affect food availability.
A long-term strategy is urgently needed to fill data gaps and develop clearer, broader sustainability accounting standards, so that it can be applied to help explore and create impactful, sustainable solutions from a triple bottom-line perspective. Such strategy must be transdisciplinary across multiple sectors of science such as animal science, agronomy, ecology, human health, and social sciences to create a holistic, integrated approach. This will also require concerted effort by various actors in the livestock and food chain sectors, as well as by local communities and government (local, regional, state, federal, international) to identify key metrics and data sources that can be used within a modelling framework to explore the net impacts of changes to production systems.
Data availability
The results presented in this review are all drawn from the scientific literature and, therefore data sharing is not applicable as no new data were generated or analysed during this review.
Conflicts of interest
James Dougherty is an Associate Editor of Animal Production Science. To mitigate this potential conflict of interest he was blinded from the review process.
Declaration of funding
This project was supported by CSIRO’s Future Protein Mission and CSIRO’s Farms of the Future Target Area initiative.
Acknowledgements
The authors thank Anette Cowie, Cathryn O’Sullivan, and Tracy Henderson for their comments on prior drafts of the manuscript. We also thank Adam Liedloff for serving as an internal reviewer and for providing the CLEM user interface image file used in creating Fig. 1.
References
Adesogan AT, Havelaar AH, McKune SL, Eilittä M, Dahl GE (2020) Animal source foods: sustainability problem or malnutrition and sustainability solution? Perspective matters. Global Food Security 25, 100325.
| Crossref | Google Scholar |
Agriculture and Agri-Food Canada (2023) Sustainable Agriculture Strategy. Available at https://agriculture.canada.ca/en/environment/sustainable-agriculture-strategy [accessed August 2023].
Agriculture Victoria (2023) Managing stubble. Available at https://agriculture.vic.gov.au/crops-and-horticulture/grains-pulses-and-cereals/crop-production/general-agronomy/stubble-burning [accessed 10 August 2023]
Alders RG, Chadag MV, Debnath NC, Howden M, Meza F, Schipp MA, Swai ES, Wingett K (2021a) Planetary boundaries and veterinary services. OIE Scientific and Technical Review 40, 439-453.
| Crossref | Google Scholar | PubMed |
Alders RG, Campbell A, Costa R, Guèye EF, Ahasanul Hoque M, Perezgrovas-Garza R, Rota A, Wingett K (2021b) Livestock across the world: diverse animal species with complex roles in human societies and ecosystem services. Animal Frontiers 11(5), 20-29.
| Crossref | Google Scholar | PubMed |
Alhashim R, Deepa R, Anandhi A (2021) Environmental impact assessment of agricultural production using LCA: a review. Climate 9(11), 164.
| Crossref | Google Scholar |
APSIM Initiative (2023) CLEM user guide and reference manual. Available at https://www.apsim.info/clem/Content/Home.htm [accessed August 2023]
Aranda J, Zambrana-Vásquez D, Del-Busto F, Círez F (2021) Social impact analysis of products under a holistic approach: a case study in the meat product supply chain. Sustainability 13, 12163.
| Crossref | Google Scholar |
Arvidsson Segerkvist K, Hansson H, Sonesson U, Gunnarsson S (2020) Research on environmental, economic, and social sustainability in dairy farming: a systematic mapping of current literature. Sustainability 12(14), 5502.
| Crossref | Google Scholar |
Australian DAFF (2022) Delivering Ag 2030. Australian Department of Agriculture, Fisheries, and Forestry, Canberra, ACT, Australia. Available at https://www.agriculture.gov.au/agriculture-land/farm-food-drought/ag2030 [accessed August 2023]
Australian DCCEEW (2018) 2018 national waste policy: less waste, more resources. Australian Department of Climate Change, Energy, the Environment, and Water, Canberra, ACT, Australia. Available at https://www.dcceew.gov.au/environment/protection/waste/publications/national-waste-policy-2018 [accessed August 2023]
Australian DCCEEW (2019) National waste policy action plan 2019, 2022. Australian Department of Climate Change, Energy, the Environment, and Water, Canberra, ACT, Australia. Available at https://www.dcceew.gov.au/environment/protection/waste/publications/national-waste-policy-action-plan [accessed November 2023]
Australian Federal Register of Legislation (2022) Climate change act 2022. Australian Federal Government, Canberra, ACT, Australia. Available at https://www.legislation.gov.au/Details/C2022A00037 [accessed August 2023]
Baber JR, Sawyer JE, Wickersham TA (2018) Estimation of human-edible protein conversion efficiency, net protein contribution, and enteric methane production from beef production in the United States. Translational Animal Science 2(4), 439-450.
| Crossref | Google Scholar | PubMed |
Barbier EB (1987) The concept of sustainable economic development. Environmental Conservation 14(2), 101-110.
| Crossref | Google Scholar |
Beal T, Ortenzi F, Fanzo J (2023) Estimated micronutrient shortfalls of the EAT-Lancet planetary health diet. The Lancet Planetary Health 7(3), e233-e237.
| Crossref | Google Scholar | PubMed |
Biesbroek S, Kok FJ, Tufford AR, Bloem MW, Darmon N, Drewnowski A, Fan S, Fanzo J, Gordon LJ, Hu FB, Lähteenmäki L, Nnam N, Ridoutt BG, Rivera J, Swinburn B, Veer Pv (2023) Toward healthy and sustainable diets for the 21st century: importance of sociocultural and economic considerations. Proceedings of the National Academy of Sciences 120(26), e2219272120.
| Crossref | Google Scholar |
Buenavista RME, Siliveru K, Zheng Y (2021) Utilization of Distiller’s dried grains with solubles: a review. Journal of Agriculture and Food Research 5, 100195.
| Crossref | Google Scholar |
Cacau LT, De Carli E, de Carvalho AM, Lotufo PA, Moreno LA, Bensenor IM, Marchioni DM (2021) Development and validation of an index based on EAT-lancet recommendations: the planetary health diet index. Nutrients 13(5), 1698.
| Crossref | Google Scholar |
Capper JL, Cady RA (2012) A comparison of the environmental impact of Jersey compared with Holstein milk for cheese production. Journal of Dairy Science 95(1), 165-176.
| Crossref | Google Scholar | PubMed |
Chen X, Wilfart A, Puillet L, Aubin J (2017) A new method of biophysical allocation in LCA of livestock co-products: modeling metabolic energy requirements of body-tissue growth. The International Journal of Life Cycle Assessment 22, 883-895.
| Crossref | Google Scholar |
Cifelli CJ, Auestad N, Fulgoni VL, III (2022) Replacing the nutrients in dairy foods with non-dairy foods will increase cost, energy intake and require large amounts of food: National Health and Nutrition Examination Survey 2011–2014. Public Health Nutrition 25(2), 332-343.
| Crossref | Google Scholar |
Clegg ME, Tarrado Ribes A, Reynolds R, Kliem K, Stergiadis S (2021) A comparative assessment of the nutritional composition of dairy and plant-based dairy alternatives available for sale in the UK and the implications for consumers’ dietary intakes. Food Research International 148, 110586.
| Crossref | Google Scholar | PubMed |
Conrad Z, Niles MT, Neher DA, Roy ED, Tichenor NE, Jahns L (2018) Relationship between food waste, diet quality, and environmental sustainability. PLoS ONE 13(4), e0195405.
| Crossref | Google Scholar |
Cui A, Zhang T, Xiao P, Fan Z, Wang H, Zhuang Y (2023) Global and regional prevalence of vitamin D deficiency in population-based studies from 2000 to 2022: a pooled analysis of 7.9 million participants. Frontiers in Nutrition 10, 1070808.
| Crossref | Google Scholar | PubMed |
de Olde EM, Sautier M, Whitehead J (2018) Comprehensiveness or implementation: challenges in translating farm-level sustainability assessments into action for sustainable development. Ecological Indicators 85, 1107-1112.
| Crossref | Google Scholar |
de Vries M, de Boer IJM (2010) Comparing environmental impacts for livestock products: a review of life cycle assessments. Livestock Science 128(1-3), 1-11.
| Crossref | Google Scholar |
Demeter L, Molnár ÁP, Bede-Fazekas A, Öllerer K, Varga A, Szabados K, Tucakov M, Kiš A, Biró M, Marinkov J, Molnár Z (2021) Controlling invasive alien shrub species, enhancing biodiversity and mitigating flood risk: a win–win–win situation in grazed floodplain plantations. Journal of Environmental Management 295, 113053.
| Crossref | Google Scholar | PubMed |
Dougherty HC, Ahmadi A, Oltjen JW, Mitloehner FM, Kebreab E (2019a) Review: modeling production and environmental impacts of small ruminants – incorporation of existing ruminant modeling techniques, and future directions for research and extension. Applied Animal Science 35(1), 114-129.
| Crossref | Google Scholar |
Dougherty HC, Oltjen JW, Mitloehner FM, DePeters EJ, Pettey LA, Macon D, Finzel J, Rodrigues K, Kebreab E (2019b) Carbon and blue water footprints of California sheep production. Journal of Animal Science 97(2), 945-961.
| Crossref | Google Scholar | PubMed |
Eady S, Grant T, Cruypenninck H, Renouf M, Mata G (2014) AusAgLCI – a life cycle inventory database for Australian agriculture. Australian Government Rural Industries Research and Development Corporation, Armidale, NSW, Australia. Available at agrifutures.com.au [accessed August 2023]
Emerenciano MCG, Rombenso AN, Vieira FdN, Martins MA, Coman GJ, Truong HH, Noble TH, Simon CJ (2022) Intensificaiton of Penaeid shrimp culture: an applied review of advances in production systems, nutrition and breeding. Animals 12, 236.
| Crossref | Google Scholar | PubMed |
Ertl P, Klocker H, Hörtenhuber S, Knaus W, Zollitsch W (2015) The net contribution of dairy production to human food supply: the case of Austrian dairy farms. Agricultural Systems 137, 119-125.
| Crossref | Google Scholar |
European Parliament (2023) EU to ban greenwashing and improve consumer information on product durability. European Parliament News. Available at https://www.europarl.europa.eu/news/en/press-room/20230918IPR05412/eu-to-ban-greenwashing-and-improve-consumer-information-on-product-durability [accessed November 2023]
FAO (2016a) Environmental performance of large ruminant supply chains: guidelines for assessment. Livestock environmental assessment and performance partnership. FAO, Rome, Italy. Available at https://www.fao.org/3/i6494e/i6494e.pdf [accessed August 2023]
FAO (2016b) Greenhouse gas emissions and fossil energy use from poultry supply chains: guidelines for assessment. Livestock environmental assessment and performance partnership. FAO, Rome, Italy. Available at https://www.fao.org/3/i6421e/i6421e.pdf [accessed August 2023]
FAO (2016c) Environmental performance of animal feeds supply chains: guidelines for assessment. Livestock environmental assessment and performance partnership. FAO, Rome, Italy. Available at https://www.fao.org/3/i6433e/i6433e.pdf [accessed August 2023]
FAO (2020a) Land use in agriculture by the numbers. FAO Sustainable Food and Agriculture. FAO, Rome. Available at https://www.fao.org/sustainability/news/detail/en/c/1274219/ [accessed August 2023]
FAO (2020b) Addressing gender inequalities to build resilience. Food and Agriculture Organization of the United Nations. Rome, 2020. Available at https://www.fao.org/3/cb0433en/CB0433EN.pdf [accessed December 2023]
FAO (2020c) The State of Food and Agriculture 2020. Overcoming water challenges in agriculture. (FAO, Rome, Italy). 10.4060/cb1447en. Available at https://www.fao.org/3/cb1447en/cb1447en.pdf [accessed August 2023].
FAO GLEAM (2023) Global Livestock Environmental Assessment Model. (FAO: Rome, Italy). Available at https://www.fao.org/gleam/en/ [accessed August 2023].
FAOSTAT (2023) FAOSTAT statistical database: suite of food security indicators. Available at https://www.fao.org/faostat/en/ - data/FS [accessed June 2023]
FIAL (2021) The national food waste strategy feasibility study – final report. The Food and Agribusiness Growth Centre Manly, Sydney, NSW, Australia. Available at https://www.fial.com.au/sharing-knowledge/food-waste [accessed August 2023]
Forster P, Storelvmo T, Armour K, Collins W, Dufresne J-L, Frame D, Lunt DJ, Mauritsen T, Palmer MD, Watanabe M, Wild M, Zhang H (2021) The earth’s energy budget, climate feedbacks, and climate sensitivity. In ‘Climate change 2021: the physical science basis. contribution of working group I to the sixth assessment report of the intergovernmental panel on climate change’. (Eds V Masson-Delmotte, P Zhai, A Pirani, SL Connors, C Péan, S Berger, N Caud, Y Chen, L Goldfarb, MI Gomis, M Huang, K Leitzell, E Lonnoy, JBR Matthews, TK Maycock, T Waterfield, O Yelekçi, R Yu, B Zhou) pp. 923–1054. (Cambridge University Press: Cambridge, UK; and New York, NY, USA) doi:10.1017/9781009157896.009
Freer M, Moore AD, Donnelly JR (2012) The GRAZPLAN animal biology model for sheep and cattle and the GrazFeed decision support tool. CSIRO Plant Industry Technical Paper. CSIRO, Canberra, ACT, Australia. Available at https://grazplan.csiro.au/wp-content/uploads/2007/08/TechPaperMay12.pdf [accessed October 2023]
Gao Y, Cabrera Serrenho A (2023) Greenhouse gas emissions from nitrogen fertilizers could be reduced by up to one-fifth of current levels by 2050 with combined interventions. Nature Food 4, 170-178.
| Crossref | Google Scholar |
Gerber PJ, Uwizeye A, Schulte RPO, Opio CI, de Boer IJM (2014) Nutrient use efficiency: a valuable approach to benchmark the sustainability of nutrient use in global livestock production? Current Opinion in Environmental Sustainability 9-10, 122-130.
| Crossref | Google Scholar |
Ghavam S, Vahdati M, Wilson IAG, Styring P (2021) Sustainable ammonia production processes. Frontiers in Energy Research 9, 580808.
| Crossref | Google Scholar |
Grealis E, O’Donoghue C (2015) The economic impact of the Irish bio-economy. Joint report issued by the Teagasc Rural Economy Programme and the Socio-Economic Marine Research Unit (SEMRU). National University of Ireland, Galway, Ireland. Available at https://ageconsearch.umn.edu/record/210704/ [accessed September 2023]
Green A, Nemecek T, Chaudhary A, Mathys A (2020) Assessing nutritional, health, and environmental sustainability dimensions of agri-food production. Global Food Security 26, 100406.
| Crossref | Google Scholar |
Green A, Nemecek T, Smetana S, Mathys A (2021) Reconciling regionally-explicit nutritional needs with environmental protection by means of nutritional life cycle assessment. Journal of Cleaner Production 312, 127696.
| Crossref | Google Scholar |
GreenDelta (2023) openLCA – the life cycle and sustainability modeling suite. Available at https://www.openlca.org/openlca/ [accessed November 2023]
Hacking T, Guthrie P (2008) A framework for clarifying the meaning of triple bottom-line, integrated, and sustainability assessment. Environmental Impact Assessment Review 28(2-3), 73-89.
| Crossref | Google Scholar |
Hansen TL, Li M, Li J, Vankerhove CJ, Sotirova MA, Tricarico JM, Cabrera VE, Kebreab E, Reed KF (2021) The ruminant farm systems animal module: a biophysical description of animal management. Animals 11(5), 1373.
| Crossref | Google Scholar | PubMed |
Harrison MT, Cullen BR, Mayberry DE, Cowie AL, Bilotto F, Badgery WB, Liu K, Davison T, Christie KM, Muleke A, Eckard RJ (2021) Carbon myopia: the urgent need for integrated social, economic and environmental action in the livestock sector. Global Change Biology 27(22), 5726-5761.
| Crossref | Google Scholar | PubMed |
Hendrie GA, Baird D, Ridoutt B, Hadjikakou M, Noakes M (2016) Overconsumption of energy and excessive discretionary food intake inflates dietary greenhouse gas emissions in Australia. Nutrients 8(11), 690.
| Crossref | Google Scholar | PubMed |
Herrero M, Grace D, Njuki J, Johnson N, Enahoro D, Silvestri S, Rufino MC (2013) The roles of livestock in developing countries. Animal 7(S1), 3-18.
| Crossref | Google Scholar |
Herrero M, Henderson B, Havlík P, Thornton PK, Conant RT, Smith P, Wirsenius S, Hristov AN, Gerber P, Gill M, Butterbach-Bahl K, Valin H, Garnett T, Stehfest E (2016) Greenhouse gas mitigation potentials in the livestock sector. Nature Climate Change 6, 452-461.
| Crossref | Google Scholar |
Herron J, Curran TP, Moloney AP, McGee M, O’Riordan EG, O’Brien D (2021) Life cycle assessment of pasture-based suckler steer weanling-to-beef production systems: effect of breed and slaughter age. Animal 15(7), 100247.
| Crossref | Google Scholar | PubMed |
Holzworth D, Huth NI, Fainges J, Brown H, Zurcher E, Cichota R, Verrall S, Herrmann NI, Zheng B, Snow V (2018) APSIM next generation: overcoming challenges in modernising a farming systems model. Environmental Modelling & Software 103, 43-51.
| Crossref | Google Scholar |
Hunt JR, Swan AD, Fettell NA, Breust PD, Menz ID, Peoples MB, Kirkegaard JA (2016) Sheep grazing on crop residues do not reduce crop yields in no-till, controlled traffic farming systems in an equi-seasonal rainfall environment. Field Crops Research 196, 22-32.
| Crossref | Google Scholar |
Huntsinger L, Barry S (2021) Grazing in California’s mediterranean multi-firescapes. Frontiers in Sustainable Food Systems 5, 715366.
| Crossref | Google Scholar |
ILCAJ (2023) Institute of life cycle assessment, Japan databases and guidelines. Available at http://ilcaj.org/en/database.php [accessed August 2023]
ILRI (2023) Women’s Empowerment in Livestock Index (WELI). International Livestock Research Institute, Nairobi, Kenya. Available at https://www.ilri.org/womens-empowerment-livestock-index-weli [accessed August 2023]
IPCC (2022) Climate Change 2022: Impacts, Adaptation and Vulnerability. Contribution of Working Group II to the Sixth Assessment Report of the Intergovernmental Panel on Climate Change. [Pörtner H-O, Roberts DC, Tignor M, Poloczanska ES, Mintenbeck K, Alegría A, Craig M, Langsdorf S, Löschke S, Möller V, Okem A, Rama B (eds.)]. Cambridge University Press. Cambridge University Press, Cambridge, UK and New York, NY, USA, 3056 pp., 10.1017/9781009325844. Available at https://report.ipcc.ch/ar6/wg2/IPCC_AR6_WGII_FullReport.pdf [accessed August 2023]
Japan MAFF (2021) Sustainable food systems strategy ‘MIDORI’. Japanese Ministry of Agriculture, Forestry, and Fisheries, Tokyo, Japan. Available at https://www.maff.go.jp/e/policies/env/env_policy/meadri.html [accessed August 2023]
Keats EC, Rappaport AI, Shah S, Oh C, Jain R, Bhutta ZA (2018) The dietary intake and practices of adolescent girls in low- and middle-income countries: a systematic review. Nutrients 10(12), 1978.
| Crossref | Google Scholar | PubMed |
Khan DSA, Das JK, Zareen S, Lassi ZS, Salman A, Raashid M, Dero AA, Khanzada A, Bhutta ZA (2022) Nutritional status and dietary intake of school-age children and early adolescents: systematic review in a developing country and lessons for the global perspective. Frontiers in Mutrition 8, 739447.
| Crossref | Google Scholar |
Klopatek SC, Marvinney E, Duarte T, Kendall A, Yang X, Oltjen JW (2022) Grass-fed vs. grain-fed beef systems: performance, economic, and environmental trade-offs. Journal of Animal Science 100(2), skab374.
| Crossref | Google Scholar |
Komarek AM, Dunston S, Enahoro D, Godfray HCJ, Herrero M, Mason-D’Croz D, Rich KM, Scarborough P, Springmann M, Sulser TB, Wiebe K, Willenbockel D (2021) Income, consumer preferences, and the future of livestock-derived food demand. Global Environmental Change 70, 102343.
| Crossref | Google Scholar | PubMed |
Kristensen JA, Svenning J-C, Georgiou K, Malhi Y (2022) Can large herbivores enhance ecosystem carbon persistence? Trends in Ecology & Evolution 37(2), 117-128.
| Crossref | Google Scholar | PubMed |
Kyttä V, Roitto M, Astaptsev A, Saarinen M, Tuomisto HL (2022) Review and expert survey of allocation methods used in life cycle assessment of milk and beef. The International Journal of Life Cycle Assessment 27, 191-204.
| Crossref | Google Scholar |
Lark TJ, Spawn SA, Bougie M, Gibbs HK (2020) Cropland expansion in the United States produces marginal yields at high costs to wildlife. Nature Communications 11, 4295.
| Crossref | Google Scholar | PubMed |
Lebacq T, Baret PV, Stilmant D (2013) Sustainability indicators for livestock farming. A review. Agronomy for Sustainable Development 33, 311-327.
| Crossref | Google Scholar |
Lecoutere E (2017) The impact of agricultural co-operatives on women’s empowerment: evidence from Uganda. Journal of Co-operative Organization and Management 5(1), 14-27.
| Crossref | Google Scholar |
Leiman DA, Madigan K, Carlin M, Cantrell S, Palakshappa D (2022) Food Insecurity in Digestive Diseases. Gastroenterology 163(3), 547-551.e13.
| Crossref | Google Scholar |
Lemke C (2021) Conceptual framework of sustainable development. In ‘Accounting and statistical analyses for sustainable development’. Sustainable Management, Wertschöpfung und Effizienz. (Ed. C Lemke) pp. 9–39. (Springer Gabler: Wiesbaden, Germany) doi:10.1007/978-3-658-33246-4_2
Li X, Zhu W, Xu F, Du J, Tian X, Shi J, Wei G (2021) Organic amendments affect soil organic carbon sequestration and fractions in fields with long-term contrasting nitrogen applications. Agriculture, Ecosystems & Environment 322, 107643.
| Crossref | Google Scholar |
Locatelli B, Pavageau C, Pramova E, Di Gregorio M (2015) Integrating climate change mitigation and adaptation in agriculture and forestry: opportunities and trade-offs. Wires Climate Change 6(6), 585-598.
| Crossref | Google Scholar |
Maron M, Lill A (2005) The influence of livestock grazing and weed invasion on habitat use by birds in grassy woodland remnants. Biological Conservation 124(4), 439-450.
| Crossref | Google Scholar |
Marty JT (2005) Effects of cattle grazing on diversity in ephemeral wetlands. Conservation Biology 19(5), 1626-1632.
| Crossref | Google Scholar |
Mazzetto AM, Falconer S, Ledgard S (2022) Mapping the carbon footprint of milk production from cattle: a systematic review. Journal of Dairy Science 105(12), 9713-9725.
| Crossref | Google Scholar | PubMed |
McClelland SC, Arndt C, Gordon DR, Thoma G (2018) Type and number of environmental impact categories used in livestock life cycle assessment: a systematic review. Livestock Science 209, 39-45.
| Crossref | Google Scholar |
Molitorisová A, Burke C (2023) Farm to fork strategy: animal welfare, EU trade policy, and public participation. Applied Economic Perspectives and Policy 45(2), 881-910.
| Crossref | Google Scholar |
Morelli J (2011) Environmental sustainability: a definition for environmental professionals. Journal of Environmental Sustainability 1(1), 2.
| Crossref | Google Scholar |
Mota-Rojas D, Braghieri A, Álvarez-Macías A, Serrapica F, Ramírez-Bribiesca E, Cruz-Monterrosa R, Masucci F, Mora-Medina P, Napolitano F (2021) The use of draught animals in rural labour. Animals 11(9), 2683.
| Crossref | Google Scholar | PubMed |
Mottet A, de Haan C, Falcucci A, Tempio G, Opio C, Gerber P (2017) Livestock: on our plates or eating at our table? A new analysis of the feed/food debate. Global Food Security 14, 1-8.
| Crossref | Google Scholar |
Mottet A, Teillard F, Boettcher P, De’ Besi G, Besbes B (2018) Review: domestic herbivores and food security: current contribution, trends and challenges for a sustainable development. Animal 12(S2), s188-s198.
| Crossref | Google Scholar | PubMed |
Mwai O, Hanotte O, Kwon Y-J, Cho S (2015) African indigenous cattle: unique genetic resources in a rapidly changing world. Asian–Australasian Journal of Animal Sciences 28(7), 911-921.
| Crossref | Google Scholar | PubMed |
National Pork Board (2017) Carbon footprint of pork production calculator. Pork Checkoff. Available at https://porkcheckoff.org/pork-production-management/sustainability/ [accessed August 2023]
Oliver B (2016) ‘The Earth Gives Us So Much’: agroecology and rural women’s leadership in Uruguay. Culture, Agriculture, Food and Environment 38(1), 38-47.
| Crossref | Google Scholar |
Osinga SA, Paudel D, Mouzakitis SA, Athanasiadis IN (2022) Big data in agriculture: between opportunity and solution. Agricultural Systems 195, 103298.
| Crossref | Google Scholar |
Picasso VD, Modernel PD, Becoña G, Salvo L, Gutiérrez L, Astigarraga L (2014) Sustainability of meat production beyond carbon footprint: a synthesis of case studies from grazing systems in Uruguay. Meat Science 98(3), 346-354.
| Crossref | Google Scholar | PubMed |
Pinotti L, Luciano A, Ottoboni M, Manoni M, Ferrari L, Marchis D, Tretola M (2021) Recycling food leftovers in feed as opportunity to increase the sustainability of livestock production. Journal of Cleaner Production 294, 126290.
| Crossref | Google Scholar |
Piñeiro V, Arias J, Dürr J, Elverdin P, Ibáñez AM, Kinengyere A, Opazo CM, Owoo N, Page JR, Prager SD, Torero M (2020) A scoping review on incentives for adoption of sustainable agricultural practices and their outcomes. Nature Sustainability 3, 809-820.
| Crossref | Google Scholar |
Price JN, Sitters J, Ohlert T, Tognetti PM, Brown CS, Seabloom EW, Borer ET, Prober SM, Bakker ES, MacDougall AS, Yahdjian L, Gruner DS, Olde Venterink H, Barrio IC, Graff P, Bagchi S, Arnillas CA, Bakker JD, Blumenthal DM, Boughton EH, Brudvig LA, Bugalho MN, Cadotte MW, Caldeira MC, Dickman CR, Donohue I, Grégory S, Hautier Y, Jónsdóttir IS, Lannes LS, McCulley RL, Moore JL, Power SA, Risch AC, Schütz M, Standish R, Stevens CJ, Veen GF, Virtanen R, Wardle GM (2022) Evolutionary history of grazing and resources determine herbivore exclusion effects on plant diversity. Nature Ecology & Evolution 6, 1290-1298.
| Crossref | Google Scholar | PubMed |
PRé Sustainability (2023) SimaPro. Available at https://simapro.com/about/ [accessed November 2023]
Purvis B, Mao Y, Robinson D (2019) Three pillars of sustainability: in search of conceptual origins. Sustainability Science 14, 681-695.
| Crossref | Google Scholar |
Reider CA, Chung R-Y, Devarshi PP, Grant RW, Hazels Mitmesser S (2020) Inadequacy of immune health nutrients: intakes in US adults, the 2005-2016 NHANES. Nutrients 12(6), 1735.
| Crossref | Google Scholar | PubMed |
Renouf M, Renaud-Gentié C (2023) Customised LCA tool for viticulture (VitLCA) for identifying environmental improvement opportunities. In ‘Proceedings of the 11th Australian conference on life cycle assessment. Responding to the climate emergency: metrics and tools for rational action’. p. 169. (Australian Life Cycle Assessment Society). Available at https://www.lcaconference.com.au/_files/ugd/9ffc42_9f5474e256de4f64ac9569b159ca2d49.pdf
Ridoutt B (2021a) Bringing nutrition and life cycle assessment together (nutritional LCA): opportunities and risks. The International Journal of Life Cycle Assessment 26, 1932-1936.
| Crossref | Google Scholar |
Ridoutt B (2021b) An alternative Nutrient Rich Food index (NRF-ai) incorporating prevalence of inadequate and excessive nutrient intake. Foods 10(12), 3156.
| Crossref | Google Scholar | PubMed |
Ridoutt B, Navarro Garcia J (2020) Cropland footprints from the perspective of productive land scarcity, malnutrition-related health impacts and biodiversity loss. Journal of Cleaner Production 260, 121150.
| Crossref | Google Scholar |
Ridoutt BG, Hendrie GA, Noakes M (2017) Dietary strategies to reduce environmental impact: a critical review of the evidence base. Advances in Nutrition 8(6), 933-946.
| Crossref | Google Scholar | PubMed |
Ridoutt BG, Hadjikakou M, Nolan M, Bryan BA (2018) From water-use to water-scarcity footprinting in environmentally extended input-output analysis. Environmental Science & Technology 52, 6761-6770.
| Crossref | Google Scholar | PubMed |
Ridoutt BG, Baird D, Anastasiou K, Hendrie GA (2019) Diet quality and water scarcity: evidence from a large australian population health survey. Nutrients 11(8), 1846.
| Crossref | Google Scholar | PubMed |
Ridoutt B, Anastasiou K, Baird D, Garcia JN, Hendrie G (2020) Cropland footprints of Australian dietary choices. Nutrients 12(5), 1212.
| Crossref | Google Scholar | PubMed |
Ridoutt BG, Baird D, Hendrie GA (2021a) Diets within planetary boundaries: what is the potential of dietary change alone? Sustainable Production and Consumption 28, 802-810.
| Crossref | Google Scholar |
Ridoutt BG, Baird D, Hendrie GA (2021b) The role of dairy foods in lower greenhouse gas emission and higher diet quality dietary patterns. European Journal of Nutrition 60, 275-285.
| Crossref | Google Scholar | PubMed |
Rivera-Huerta A, de la Salud Rubio Lozano M, Padilla-Rivera A, Güereca LP (2019) Social Sustainability Assessment in Livestock Production: A Social Life Cycle Assessment Approach. Sustainability 11(16), 4419.
| Crossref | Google Scholar |
Rotz CA (2018) Modeling greenhouse gas emissions from dairy farms. Journal of Dairy Science 101(7), 6675-6690.
| Crossref | Google Scholar | PubMed |
Rotz CA, Corson MS, Chianese DS, Montes F, Hafner SD, Bonifacio HF, Coiner CU (2022) The integrated farm system model: reference manual version 4.7. United States Department of Agriculture, Agricultural Research Service, Pasture Systems and Watershed Management Research Unit, University Park, Pennsylvania, USA. Available at https://www.ars.usda.gov/ARSUserFiles/80700500/Reference%20Manual.pdf [accessed July 2023]
Ruggerio CA (2021) Sustainability and sustainable development: a review of principles and definitions. Science of The Total Environment 786, 147481.
| Crossref | Google Scholar | PubMed |
Salemdeeb R, zu Ermgassen EKHJ, Kim MH, Balmford A, Al-Tabbaa A (2017) Environmental and health impacts of using food waste as animal feed: a comparative analysis of food waste management options. Journal of Cleaner Production 140, 871-880.
| Crossref | Google Scholar | PubMed |
Sandström V, Chrysafi A, Lamminen M, Troell M, Jalava M, Piipponen J, Siebert S, van Hal O, Virkki V, Kummu M (2022) Food system by-products upcycled in livestock and aquaculture feeds can increase global food supply. Nature Food 3, 729-740.
| Crossref | Google Scholar |
Scurlock AM, Brown E, Davis CM (2022) Food insecurity in children and adults with food allergies. Annals of Allergy, Asthma & Immunology 129(4), 424-429.
| Crossref | Google Scholar | PubMed |
Seidel C (2016) The application of life cycle assessment to public policy development. The International Journal of Life Cycle Assessment 21, 337-348.
| Crossref | Google Scholar |
Sejian V, Bhatta R, Gaughan JB, Dunshea FR, Lacetera N (2018) Review: adaptation of animals to heat stress. Animal 12(S2), s431-s444.
| Crossref | Google Scholar | PubMed |
Setsoafia ED, Ma W, Renwick A (2022) Effects of sustainable agricultural practices on farm income and food security in northern Ghana. Agricultural and Food Economics 10(1), 9.
| Crossref | Google Scholar |
Shams-White MM, Pannucci TE, Lerman JL, Herrick KA, Zimmer M, Meyers Mathieu K, Stoody EE, Reedy J (2023) Healthy eating index-2020: review and update process to reflect the Dietary Guidelines for Americans, 2020-2025. Journal of the Academy of Nutrition and Dietetics 123, 1280-1288.
| Crossref | Google Scholar | PubMed |
Shirosaki Marçal de Souza L, Nunes AO, Giusti G, Saavedra YMB, Rodrigues TO, Nunes Braga TE, Lopes Silva DA (2021) Evaluating and ranking secondary data sources to be used in the Brazilian LCA database – ‘SICV Brasil’. Sustainable Production and Consumption 26, 160-171.
| Crossref | Google Scholar |
Shlisky J, Mandlik R, Askari S, Abrams S, Belizan JM, Bourassa MW, Cormick G, Driller-Colangelo A, Gomes F, Khadilkar A, Owino V, Pettifor JM, Rana ZH, Roth DE, Weaver C (2022) Calcium deficiency worldwide: prevalence of inadequate intakes and associated health outcomes. Annals of the New York Academy of Sciences 1512(1), 10-28.
| Crossref | Google Scholar |
Son HN, Chi DTL, Kingsbury A (2019) Indigenous knowledge and climate change adaptation of ethnic minorities in the mountainous regions of Vietnam: a case study of the Yao people in Bac Kan Province. Agricultural Systems 176, 102683.
| Crossref | Google Scholar |
Sphera (2023) Sphera product sustainability solutions software (Formerly known as GaBi). Available at https://sphera.com/product-sustainability-software/ [accessed November 2023]
Stavi I, Argaman E, Zaady E (2016) Positive impact of moderate stubble grazing on soil quality and organic carbon pool in dryland wheat agro-pastoral systems. Catena 146, 94-99.
| Crossref | Google Scholar |
Steenson S, Buttriss JL (2020) The challenges of defining a healthy and ‘sustainable’ diet. Nutrition Bulletin 45(2), 206-222.
| Crossref | Google Scholar |
Stelle I, Kalea AZ, Pereira DIA (2019) Iron deficiency anaemia: experiences and challenges. Proceedings of the Nutrition Society 78(1), 19-26.
| Crossref | Google Scholar |
Sugiura K, Yamatani S, Watahara M, Onodera T (2009) Ecofeed, animal feed produced from recycled food waste. Veterinaria Italiana 45(3), 397-404.
| Google Scholar | PubMed |
Sun Y-T, Ruiz-Carrascal J (2023) Home made vegan nuggets with texturized soy protein and tempeh as compared to chicken-based ones: texture, consumer perception and environmental impact. International Journal of Gastronomy and Food Science 33, 100748.
| Crossref | Google Scholar |
Tam E, Keats EC, Rind F, Das JK, Bhutta ZA (2020) Micronutrient supplementation and fortification interventions on health and development outcomes among children under-five in low- and middle-income countries: a systematic review and meta-analysis. Nutrients 12(2), 289.
| Crossref | Google Scholar | PubMed |
Tedeschi LO (2023) Review: Harnessing extant energy and protein requirement modeling for sustainable beef production. Animal 17, 100835.
| Crossref | Google Scholar |
Tepper S, Geva D, Shahar DR, Shepon A, Mendelsohn O, Golan M, Adler D, Golan R (2021) The SHED index: a tool for assessing a Sustainable HEalthy Diet. European Journal of Nutrition 60(7), 3897-3909.
| Crossref | Google Scholar | PubMed |
Thamo T, Addai D, Pannell DJ, Robertson MJ, Thomas DT, Young JM (2017) Climate change impacts and farm-level adaptation: economic analysis of a mixed cropping–livestock system. Agricultural Systems 150, 99-108.
| Crossref | Google Scholar |
Thomas DT, Beletse YG, Dominik S, Lehnert SA (2021) Net protein contribution and enteric methane production of pasture and grain-finished beef cattle supply chains. Animal 15(12), 100392.
| Crossref | Google Scholar | PubMed |
Thomas DT, Mata G, Toovey AF, Hunt PW, Wijffels G, Pirzl R, Strachan M, Ridoutt BG (2023) Climate and biodiversity credentials for Australian grass-fed beef: a review of standards, certification and assurance schemes. Sustainability 15, 13935.
| Crossref | Google Scholar |
Thompson L, Rowntree J, Windisch W, Waters SM, Shalloo L, Manzano P (2023) Ecosystem management using livestock: embracing diversity and respecting ecological principles. Animal Frontiers 13(2), 28-34.
| Crossref | Google Scholar | PubMed |
Thornton PK, Whitbread A, Baedeker T, Cairns J, Claessens L, Baethgen W, Bunn C, Friedmann M, Giller KE, Herrero M, Howden M, Kilcline K, Nangia V, Ramirez-Villegas J, Kumar S, West PC, Keating B (2018) A framework for priority-setting in climate smart agriculture research. Agricultural Systems 167, 161-175.
| Crossref | Google Scholar |
Tragnone BM, D’Eusanio M, Petti L (2022) The count of what counts in the agri-food Social Life Cycle Assessment. Journal of Cleaner Production 354, 131624.
| Crossref | Google Scholar |
Trichopoulou A, Costacou T, Bamia C, Trichopoulos D (2003) Adherence to a Mediterranean diet and survival in a Greek population. The New England Journal of Medicine 348(26), 2599-2608.
| Crossref | Google Scholar | PubMed |
Truong H, Frerichs S, Blyth D, Hines B, Bourne N, Rombenso A, Simon S (2021) Evaluating the usefulness of food waste ingredients in juvenile prawn diets. CSIRO, Australia. Available at https://uploads-ssl.webflow.com/639cdc558defc036babb846f/639ecca098945184f6209d9a_CSIRO-FR%20Prawn%20report%20June%202021%20Final.pdf [accessed August 2023]
Tsakiridis A, O’Donoghue C, Hynes S, Kilcline K (2020) A comparison of environmental and economic sustainability across seafood and livestock product value chains. Marine Policy 117, 103968.
| Crossref | Google Scholar |
UNCED (1992) Report of the United Nations Conference on Environment and Development (UNCED). United Nations (UN), Rio de Janeiro, Brazil. Available at https://www.un.org/en/conferences/environment/rio1992 [accessed October 2023]
UNEP/SETAC (2009) Guidelines for social life cycle assessment of products, France. United Nations Environment Programme – Society of Environmental Toxicology and Chemistry, United Nations, Belgium. Available at https://wedocs.unep.org/bitstream/handle/20.500.11822/7912/-Guidelines%20for%20Social%20Life%20Cycle%20Assessment%20of%20Products-20094102.pdf?sequence=3andamp%3BisAllowed= [accessed September 2023]
UNEP/SETAC (2020) Guidelines for social life cycle assessment of products, France. United Nations Environment Programme – Society of Environmental Toxicology and Chemistry, United Nations, Belgium. Available at https://wedocs.unep.org/handle/20.500.11822/34554?show=full [accessed September 2023]
United Nations (2022) World population prospects 2022. Summary of results. United Nations, New York City, USA. Available at https://www.un.org/development/desa/pd/sites/www.un.org.development.desa.pd/files/wpp2022_summary_of_results.pdf [accessed August 2023]
United Nations (2023) Global sustainable development report 2023. Advance Unedited Version. United Nations, New York City, USA. Available at https://sdgs.un.org/sites/default/files/2023-06/Advance%20unedited%20GSDR%2014June2023.pdf [accessed September 2023]
US DHHS and USDA (2020) Dietary guidelines for Americans, 2020–2025. 9th edn. Available at DietaryGuidelines.gov [accessed August 2023]
Valdivia S, Sonnemann G, Milà i Canals L (2017) LCA mainstreaming conditions in Latin America—based on learnings from 2005 to 2014. The International Journal of Life Cycle Assessment 22, 485-491.
| Crossref | Google Scholar |
Van Amburgh ME, Russomanno KL, Higgs RA, Chase LE (2019) Invited Review: modifications to the Cornell Net Carbohydrate and Protein System related to environmental issues – capability to evaluate nitrogen and phosphorus excretion and enteric carbon dioxide and methane emissions at the animal level. Applied Animal Science 35(1), 101-113.
| Crossref | Google Scholar |
van Barneveld RJ, Hewitt RJE, D’Souza DN (2023) Net protein contribution from an intensive Australian pork supply chain. Animal Production Science
| Crossref | Google Scholar |
van Hal O, Weijenberg AAA, de Boer IJM, van Zanten HHE (2019) Accounting for feed-food competition in environmental impact assessment: towards a resource efficient food-system. Journal of Cleaner Production 240, 118241.
| Crossref | Google Scholar |
van Zanten HHE, Bikker P, Meerburg BG, de Boer IJM (2018a) Attributional versus consequential life cycle assessment and feed optimization: alternative protein sources in pig diets. The International Journal of Life Cycle Assessment 23, 1-11.
| Crossref | Google Scholar |
Van Zanten HHE, Van Ittersum MK, De Boer IJM (2019) The role of farm animals in a circular food system. Global Food Security 21, 18-22.
| Crossref | Google Scholar |
von Braun J, Hendriks SL (2023) Full-cost accounting and redefining the cost of food: implications for agricultural economics research. Agricultural Economics 54(4), 451-454.
| Crossref | Google Scholar |
Weidemann S, Yan M (2014) Livestock meat processing: inventory data and methods for handling co-production for major livestock species and meat products. In ‘Proceedings of the 9th international conference on life cycle assessment in the agri-food sector’. pp. 1512–1520. Available at https://www.cabdirect.org/cabdirect/abstract/20153221306
White RR, Hall MB (2017) Nutritional and greenhouse gas impacts of removing animals from US agriculture. Proceedings of the National Academy of Sciences 114(48), E10301-E10308.
| Crossref | Google Scholar | PubMed |
Wiedemann SG, Ledgard SF, Henry BK, Yan M-J, Mao N, Russell SJ (2015) Application of life cycle assessment to sheep production systems: investigating co-production of wool and meat using case studies from major global producers. The International Journal of Life Cycle Assessment 20, 463-476.
| Crossref | Google Scholar |
Wingett K, Alders R (2023) Distribution of nutrients across the edible components of a modelled typical Australian lamb: a case study. Research Directions: One Health 1, 1-9.
| Crossref | Google Scholar |
Wiseman L, Sanderson J, Zhang A, Jakku E (2019) Farmers and their data: an examination of farmers’ reluctance to share their data through the lens of the laws impacting smart farming. NJAS: Wageningen Journal of Life Sciences 90–91, 100301.
| Crossref | Google Scholar |
Witjes S, Vermeulen WJV, Cramer JM (2017) Assessing corporate sustainability integration for corporate self-reflection. Resources, Conservation and Recycling 127, 132-147.
| Crossref | Google Scholar |
Wyngaarden SL, Lightburn KK, Martin RC (2020) Optimizing livestock feed provision to improve the efficiency of the agri-food system. Agroecology and Sustainable Food Systems 44(2), 188-214.
| Crossref | Google Scholar |
Young M, Young J (2022) Australian farm optimisation model documentation. Available at https://australian-farm-optimising-model.readthedocs.io/en/latest/CorePyomo.html [accessed September 2023]
Young JM, Trompf J, Thompson AN (2014) The critical control points for increasing reproductive performance can be used to inform research priorities. Animal Production Science 54(6), 645-655.
| Crossref | Google Scholar |
Zavaleta N (2017) Anemia infantil: retos y oportunidades al 2021 [Childhood anemia: challenges and opportunities for 2021]. Revista Peruana de Medicina Experimental y Salud Pública 34(4), 588-589.
| Crossref | Google Scholar | PubMed |
Zhang X, Lark TJ, Clark CM, Yuan Y, LeDuc SD (2021) Grassland-to-cropland conversion increased soil, nutrient, and carbon losses in the US Midwest between 2008 and 2016. Environmental Research Letters 16, 054018.
| Crossref | Google Scholar | PubMed |
zu Ermgassen EKHJ, Phalan B, Green RE, Balmford A (2016) Reducing the land use of EU pork production: where there’s swill, there’s a way. Food Policy 58, 35-48.
| Crossref | Google Scholar |