A regional-scale assessment of nutritional-system strategies for abatement of enteric methane from grazing livestock
A. K. Almeida

A School of Environmental and Rural Science, University of New England, Armidale, NSW 2351, Australia.
B School of Agriculture and Environment, Massey University, Private Bag 11-2221, 4440 Palmerston North, New Zealand.
Animal Production Science 63(15) 1461-1472 https://doi.org/10.1071/AN22315
Submitted: 5 September 2022 Accepted: 17 July 2023 Published: 15 August 2023
Abstract
Progress towards methane (CH4) mitigation for the red meat, milk and wool sectors in Australia and reduced CH4 emissions intensity (g CH4/kg animal product, typically milk or liveweight gain) involves not only reduced net emissions but also improved productive efficiency. Although nutritional additives have potential to reduce CH4 production rate of livestock (g CH4/head.day), systemic improvement of the nutrition of grazing breeding females, the largest source of CH4 emissions in Australian agriculture, will also be required to reduce emissions intensity. Systemic changes that increase productive efficiency for producers are part of the economic and environmental ‘win–win’ of reducing emissions intensity, and so offer good potential for adoption by industry. For sheep and cattle breeding enterprises, improved nutrition to achieve a younger age at first joining and increased reproductive rate will reduce the proportion of CH4-emitting, but unproductive, animals in a herd. However, if breeding stock are managed to be more productive (e.g. by superior nutrition leading to greater product/breeder) and more efficient (e.g. greater product per kilogram DMI) the producer is faced with the following management challenge. Should the enterprise increase stock numbers to utilise surplus feed and gain extra product, or reduce stock numbers to maintain previous product output with smaller enterprise net emissions (and emissions intensity), and so make land available for other uses (e.g. tree plantings, conservation zones). The right balance of incentives and price on carbon is necessary to achieve a result whereby total emissions from Australian agriculture are reduced, and so a positive impact on climate change is achieved.
Keywords: emissions, farm-level solutions, GHG inventory, greenhouse gases, methane, mitigation pathways, ruminant, simulation.
Introduction
Enteric-methane (CH4) emission represents a natural energy loss from the ruminant fermentation process, and it is the principal greenhouse-gas (GHG) emission from agriculture. Enteric CH4 accounts for 43% of the GHG emissions from agriculture, globally (Herrero et al. 2016). In Australia 73% of the GHG emissions from the agriculture sector are CH4 (Fig. 1). As CH4 has a brief lifetime in the atmosphere (i.e. from 8.4 to 12 years, Ehhalt et al. 2001), mitigating CH4 may offer a substantial pathway to achieve climate stabilisation targets. Inspired by the methane pledge raised on the 26th United Nations Climate Change Conference of the parties (COP26) recently held in Glasgow, in which a global partnership committed to lower CH4 emissions by 30% by 2030, we used the state of New South Wales to assess whether and how we can achieve this goal.
Contribution of each ruminant species and category (%) to the total enteric-methane production in New South Wales (NSW). (Source: AGEIS; Australian Government 2020).
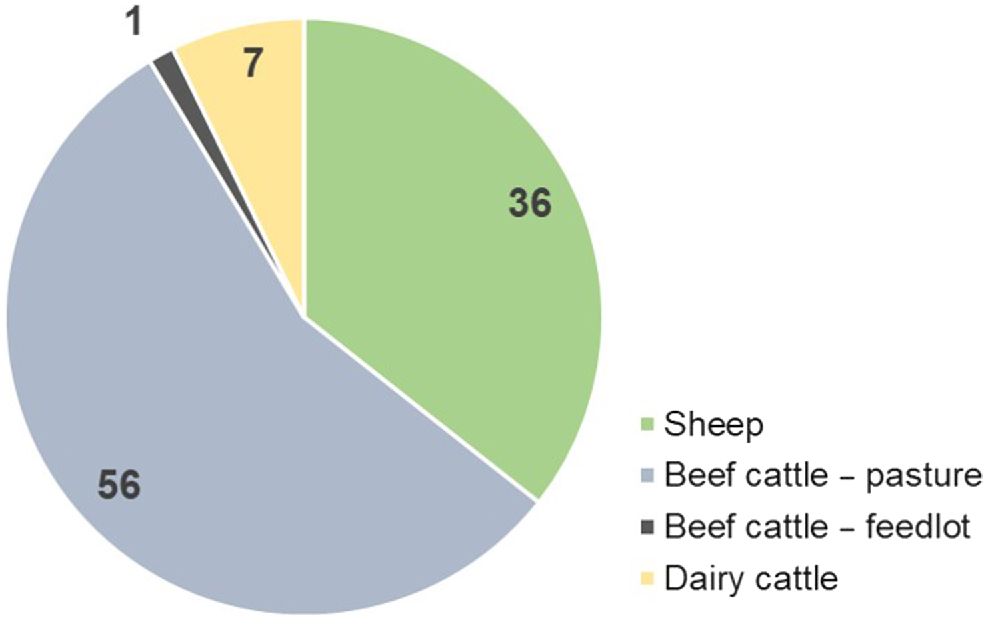
In NSW, livestock CH4 emissions are mainly due to the 26.1 million head of sheep and 4.92 million head of cattle (MLA 2019; Australian Government 2020). There are opportunities to reduce CH4 emissions while improving ruminant efficiency from a metabolic to a farming-system level. Inspiration for the potential of improved productivity and efficiency to reduce CH4 emissions at a state-wide scale can be taken from Capper et al. (2009) who, speaking of the change in the US dairy industry since 1944, said that ‘Modern dairy practices require considerably fewer resources than dairying in 1944 with 21% of animals, 23% of feedstuffs, 35% of the water, and only 10% of the land required to produce the same 1 billion kilograms of milk. Waste outputs were similarly reduced, with modern dairy systems producing 24% of the manure, 43% of CH4, and 56% of N2O per billion kg of milk compared with equivalent milk from historical dairying.’ (p. 2160).
The developing suite of mitigation options for enteric CH4 have been regularly reviewed and tend to focus on dietary additives, with potential to reduce CH4 yield at animal scale (e.g. Martin et al. 2010; Cottle et al. 2011; Grainger and Beauchemin 2011; Hristov et al. 2013; Almeida et al. 2021). However, progress towards carbon neutrality for the red meat sector in Australia as well as reduced emissions intensity (g CH4/kg animal product, typically milk or liveweight gain) by 30% for the dairy sector involves not only reduced emissions but also improved productive efficiency. Thus, whole-farm approaches based on systemic nutritional improvement, which can lead to reductions in net CH4 emissions at enterprise scale, will be essential.
The NSW state GHG inventory shows that 91% of enteric CH4 is sourced from grazing cattle and sheep (i.e. 92% of ruminant population; Fig. 1). Moreover, cows (3 021 292 head) and ewes kept for reproduction purposes (15 229 986 head) are 58.6% of the total ruminant population in NSW (Fig. 2), contributing to 60% of the state’s enteric CH4 emissions; thus, targeting improved fertility in breeding females is a real opportunity to reduce CH4 emissions in NSW.
Ruminant population by species (vertical bar) and category (pie chart) in New South Wales (NSW). (Source: AGEIS; Australian Government 2020).
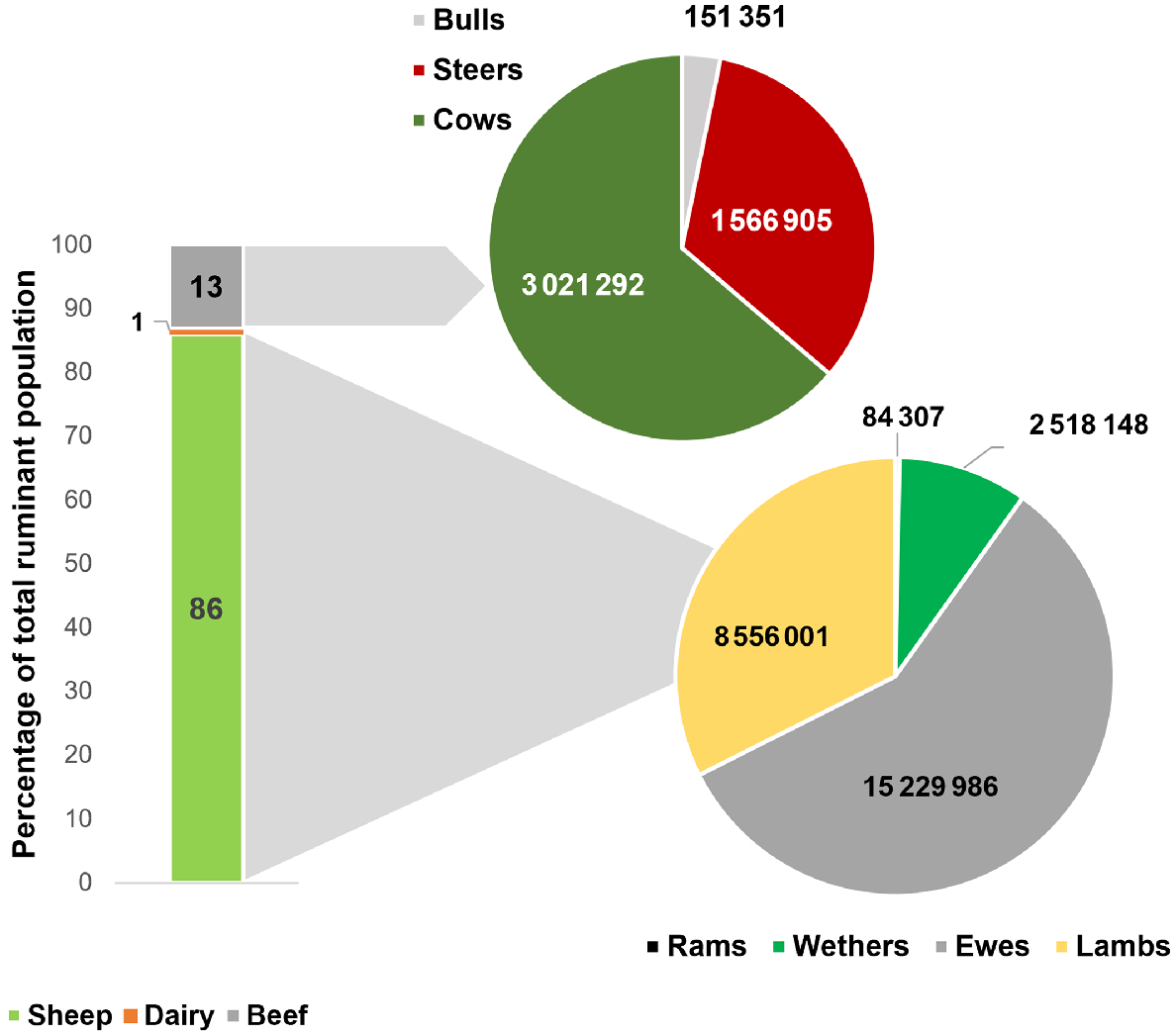
This review assesses whole-farm strategies to reduce total enteric emissions produced by ruminant livestock in NSW, with a focus on nutritional strategies that can improve grazing-animal efficiency at enterprise scale, in particular that of breeding females. While improved nutrition will be the most important strategy for achieving reduced emission intensity, other strategies that can improve productivity, such as genetic selection, improved animal health, and targeted turning off of unproductive animals, may also contribute (in some cases, additively), but are not the focus of this review. Methane-abating dietary additives have been comprehensively reviewed elsewhere (Almeida et al. 2021; Almeida and Hegarty 2021), and in most cases, challenges with delivery of such additives to grazing production systems are yet to be overcome. This review, first, addresses the means by which improved nutritional management can achieve improvement in reproductive efficiency. It then assesses the means and implications of improving the nutritional quality of the grazing basal feed for enterprise-scale emissions and/or using methane-supressing feed additives. Finally, the impact of changing reproductive rate (as a sum of these strategies) in NSW livestock by 5–10% is assessed using simulations to verify the sensitivity of the current CH4 emissions by using the National Greenhouse Gases Inventory methodology in different scenarios.
Calculations of enteric-CH4 contribution to GHG inventories
The method used in the national and state inventories to estimate enteric CH4 emissions (Department of Industry, Innovation, Climate Change, Science, Research and Tertiary Education 2011) differs between sheep and cattle, but both ultimately rest on the relationship between CH4 yield and dry-matter intake (DMI). Sheep emissions are based on calculations that include liveweight, growth and dry-matter digestibility (DMD) of feed to calculate DMI, while grazing beef emissions rely on animal liveweight and growth to calculate DMI. For grazing beef and dairy cattle, there is an assumed relationship between dry-matter intake (DMI) and CH4 production of 20.7 g CH4/kg DMI (Charmley et al. 2016) where DMI is calculated from liveweight and liveweight gain by using the equations of Minson and McDonald (1987), for each class of cattle × season × state × region, and a separate feed intake adjustment for milk production for lactating beef or dairy cattle, which for dairy cattle incorporates the metabolisability of the diet (Minson and McDonald 1987).
For sheep, CH4 production is calculated by the the following linear equation proposed by Howden et al. (1994):
where DMI is the product of potential feed intake (as calculated by AFRC 1990) by using a function of liveweight and metabolisable energy content (Minson and McDonald 1987; Department of Industry, Innovation, Climate Change, Science, Research and Tertiary Education 2011), relative feed intake (as calculated by White et al. (1983)) by using a function of the dry-matter availability), and additional DMI for milk production (Department of Industry, Innovation, Climate Change, Science, Research and Tertiary Education 2011), for each class of sheep × season × region.
The first point of consideration is whether Minson and McDonald’s (1987) equation should be applied to sheep, as it was built for cattle. The implication of these methods for calculating livestock emissions is that increased animal productivity (e.g. liveweight gain), which in grazing livestock is primarily achieved by increasing digestible organic-matter (OM) intake, will lead to an increased CH4 production rate (g CH4/head.day), albeit with a reduced emissions intensity. The impact of changing the system productivity on enterprise-scale emissions is wholly dependent on how the manager chooses to respond to increased productivity in terms of livestock numbers and area under grazing. All that these management strategies provide to a livestock manager is opportunity, i.e. opportunity to choose the balance of emission change or productivity change they want to achieve. Alcock and Hegarty (2011) explored this for lamb producers at Cowra in NSW and found that most of the management technologies considered reduced emissions intensity by 10–20%, comparing the worst with the best practises. However, if breeding stock are managed to be more productive (e.g. by superior nutrition leading to greater product/breeder) and more feed efficient (e.g. greater product per kilogram DMI), the farmer is faced with the challenge. The challenge is whether to increase stock numbers to utilise surplus feed and gain extra product (for potentially reduced emissions intensity, but usually greater net enterprise emissions), or reduce stock numbers to maintain previous product output with smaller enterprise net emissions (and emissions intensity), and so make land available for other uses (e.g. tree plantings, conservation zones). Although the first option is most likely to be adopted by most producers; with the right balance of incentives, including a price on carbon, the latter option may have a pathway to adoption via the potential for carbon sequestration in ungrazed land, thus further reducing net GHG emissions by the enterprise. The means by which management of the animal and feed can be changed to provide this opportunity are described below.
Modifications of the on-farm production system itself are particularly desirable in reducing livestock emissions, because in general they use known and available technologies, do not require new chemical products, and are associated with increased animal productivity co-benefits. These changes include intensification made possible through improved basal feed (Pinares-Patiño et al. 2009), improved reproductive performance (Alcock and Hegarty 2011) and/or improved metabolic efficiency of the animal that could be achieved by breeding (Nkrumah et al. 2006) or improved health (Eckard et al. 2010). To decrease CH4 emissions from a grazing enterprise, all these strategies are most effectively applied to decrease the quantity of feed consumed (and therefore the quantity of CH4 produced) by the breeding females in the flock or herd. By either reducing daily emissions from this group or increasing the commercial product they yield, the emissions intensity is reduced. Consequently, they provide significant flexibility for the land manager to rearrange their land allocation and stock numbers around personal priorities for emission and productivity targets (Alcock and Hegarty 2011). Significantly, there is a slightly different balance of management for productivity or emissions in breeding and slaughter animals. For offspring raised for meat production, maximising intake and growth to reach weaning and slaughter points sooner can reduce both emissions intensity and total enterprise emissions. However, because breeding females are retained in the herd, even during unproductive phases (until culling for on-going lack of fertility, age or other reasons), optimising, rather than maximising, intake to achieve maximal reproductive performance is likely to be more efficient economically, and from an emissions perspective.
Changing the nutritional management of breeding animals
Nutritional management by which the reproductive efficiency of grazing ruminants can be improved focus on reducing age at first joining, inter-calving or inter-lambing interval, and increasing weaning rates, especially per kilogram of breeding female.
Age at first joining
Animals intended for breeding are not contributing economically until they deliver their first progeny, but are always consuming feed and generating GHG emissions. Therefore, one way to increase efficiency in breeding flocks and herds is to reduce the unproductive time before animals are first joined. This has substantial impacts in reducing the emission intensity of milk (Christie et al. 2016) and of lamb, as well as increasing profitability (Alcock et al. 2015; Tocker et al. 2020). It also increases the speed of genetic gain (Newton et al. 2017). Timing of onset of puberty is determined by liveweight, rather than the rate or timing of liveweight gain, and so is subject to post-weaning nutritional control (Bruinje et al. 2021). Dietary energy restriction can delay the onset of puberty by inhibiting the frequency of pulsatile release of lutenising hormone (LH) (Schillo et al. 1992). Within breeds, this appears to be driven by bodyweight and thus metabolic fuel availability. Adipose fat is an endocrine tissue that affects reproduction through the secretion of leptin (Schillo et al. 1992; D’Occhio et al. 2019). A higher post-weaning plane of nutrition is associated with increasing plasma leptin concentrations, which stimulate the hypothalamus kisspeptin release and maturation of gonadotropin-releasing hormone (GnRH) expressing neurons with the onset of puberty, and ultimately release of LH (D’Occhio et al. 2019). Other proposed mechanisms for the effect of nutrition on the onset of puberty include the action of ghrelin (elevated during negative energy balance) and insulin-like growth factor-1 (mediated via insulin–glucose homeostasis) to regulate activity of the GnRH receptors in the brain (D’Occhio et al. 2019). Research for determining an optimal rate of post-weaning growth to enable early joining and maximising lifetime breeding productivity in cattle has shown that results from more recent work (Le Cozler et al. 2008) diverge from those of early research (Short and Bellows 1971). This suggests that with genetic progress, the optimal nutritional strategies to achieve early joining may have changed and need re-assessment (Pitchford et al. 2018; Walmsley et al. 2018).
Simulations of reducing age of joining in Merino ewes from a baseline of 19 months of age to 7 months (Alcock and Hegarty 2011; Alcock et al. 2015) resulted in a removal of a cohort of unproductive, but still methane-emitting, maiden ewes. Despite lower lambing rates in this cohort, the resulting increase in lamb weaned per female and per hectare reduced the emissions intensity of both wool and lamb by ~5% and increased overall enterprise profit by 6% (Alcock et al. 2015). However, to achieve reduction in enterprise-scale emissions, this additional profit must be at least partially foregone by a reduction in stocking rate. When stocking rates in the simulation were adjusted for a profit-neutral outcome, reductions in absolute emissions of 0.26 and 0.36 t CO2-eq/ha were observed for yearling and weaner enterprises respectively (Alcock et al. 2015). Alcock et al. (2015) calculated a break-even carbon price of more than AUD$150/t CO2-eq for a yearling enterprise (AUD$37/ha) and AUD$267/t CO2-eq (AUD$94/ha) for a weaner enterprise to account for the opportunity cost of the reduced stocking rate in the profit-neutral scenario.
Similarly, in beef production, joining at a younger age was shown to be economically preferable to joining at later ages, irrespective of any culling for age rule (Nunez-Dominguez et al. 1991). In rangeland beef production systems (a small part of NSW beef production), early joining may have less benefit than it has in sheep production systems. A case study analysis (Cullen et al. 2016) of an enterprise that joined Brahman heifers at 360 kg at 1 year of age, instead of a typical enterprise that joins heifers at 360 kg at 2 years of age, increased liveweight sold-off property by 8%. If the same animal equivalent (AE) stocking rate was maintained, emissions intensity of liveweight gain (LWG) was reduced by 7%, but enterprise emissions were maintained. By taking advantage of the increased productive efficiency of earlier joining, and reducing AEs stocked so that liveweight weaned and/or sold was maintained, both emissions intensity and total enterprise emissions were reduced by ~ 7%. The gross margin outcomes of both strategies were ~10% higher than was baseline. More modelling is needed to assess the economic and emission impacts of improving age at first joining in temperate beef production systems. There is likely to be little scope for reducing GHG in dairy production systems from improved breeding (Lean and Moate 2021).
Unless replacement females are at a suitable weight to achieve successful early joining, then joining underweight young animals will be disadvantageous to the economic yield of the herd or flock (Farrell et al. 2019) and it would be expected that the same adverse effect would diminish emission-intensity gains due to the need for supplementary feeding of lighter weight dry females that either fail to get pregnant or fail to rebreed due to weight and condition.
The implications of retaining the more-productive females in the flock/herd is two-fold for emissions. (1) The number of non-productive females being reared to mating age is reduced, resulting in less CH4 produced by animals not contributing to marketable livestock and (2) the non-productive (but CH4-producing) rearing period of replacements becomes a lower proportion of the average lifetime of breeders in the herd/flock, so the emissions intensity over the lifetime is reduced.
Fecundity management
Measuring improvement in reproductive rate of multiparous females is complex as there are several indices available, all with their own issues of inaccuracy. The best measures of fertility account for the efficiency with which females reconceive and successfully raise their offspring. Weaning rate is the most comprehensive single index, because it takes into account reproductive losses from mating to lamb weaning, although it does not account for maternal deaths. In terms of emissions intensity and enterprise-scale emissions, breeder-herd efficiency indices, such as those for beef production systems which measure kilograms of weaner liveweight weaned per 400 kg of breeding female are the most useful measures of breeding efficiency, due to the breeder liveweight impacts on CH4 emissions.
The nutritional driver of reproductive performance in multiparous females is not bodyweight, as for maidens, but body condition score (BCS), which is an indication of subcutaneous fat. BCS at calving, rather than change in BCS, is the single most important determinant of resumption of ovarian cycling post-partum (D’Occhio et al. 2019). It is possible that mechanisms of the effect of nutrition and adipose tissue mass are acting on the resumption of ovarian cycling in the post-partum female similar to those acting in the pubertal maiden (D’Occhio et al. 2019).
Development of BCS depends on mature bodyweight. In this respect, selection for females with lower maintenance-energy requirements, (i.e. lower mature bodyweight), can achieve productivity efficiencies concurrent with lower enterprise emissions and emissions intensity. However, larger frame size is associated with faster LWG and thus improved emissions intensity of lamb and beef, and so it is important not to compromise the efficiency of offspring for slaughter, by the use of low-liveweight terminal sires.
Consistent with reducing emissions intensity, increasing the reproductive output can also be achieved by targeted nutritional interventions to ovulation. Specifically, this includes ovulation stimulation by a nutritional pulse (flushing; Banchero et al. 2021). The greatest nutritional impact on ovulation is overall energy intake, although short-term supplementation with either rumen degradable protein or rumen undegraded protein can also increase ovulation rate (Banchero et al. 2021)
Harrison et al. (2014) showed that increasing fecundity reduced emissions intensity from 9.3 to 7.3 t CO2-e/t clean fleece weight plus liveweight, in a sheep breeding enterprise. There is scope for strategic supplements to be used by breeding flocks in NSW to increase reproductive output and, consequently, change herd/flock structure and reduce emissions intensity (Almeida et al. 2021; Almeida and Hegarty 2021). The same principles apply in dairy where extended lactations (within a year) reduce emissions intensity of milk production (Lehmann et al. 2014). Doran-Browne et al. (2015) found that the economic impact of extending productive life was greater in the dairy herd than in wool-growing enterprises.
Basal-feed management change
Improving quality of available forage
The key consequences of improved pasture quality occur through animal productivity impacts such as faster body and or wool growth, shorter lifespan for slaughter animals, higher breeder BCS, so reduced age to joining and period to the subsequent conception. This reduces the need for replacement animals and perhaps sustains longer persistence in the herd/flock. Therefore, improving the basal feed supports the implementation of other improved animal management strategies.
The importance of DMD (and thus intake) in the National Inventory method for sheep CH4 emissions has implications for the estimated emissions of enterprises undertaking basal-feed quality improvement. DMD has strong positive correlations with DMI in temperate pastures, but this relationship varies among seasons (Arnold and Dudzinski 1967; Michell 1973), with, for example, low residuals in winter (r = +0.81) but significantly higher when considered over all seasons (r = +0.48) (Michell 1973). For many improvements in pasture species and management, increases in digestibility are also concurrent with improved leaf density or plant morphology for grazing, which increases bite size and thus intake, separate from the effects of DMD (Stobbs 1973).
Very simplistically and without considering known feed-forward effects of improved DMD, as the National Inventory largely does not permit this, we simulated the effect of increased DMD for sheep in NSW by using the National Inventory Report (https://www.industry.gov.au/data-and-publications/national-greenhouse-accounts-2019/national-inventory-report-2019) framework. In the inventory, NSW seasonal values for DMD are assumed to be 75%, 61%, 64%, and 72% for spring, summer, autumn and winter respectively, for sheep only. Simulating the effect of improved digestibility, National Inventory seasonal DMD values were increased by 5% before insertion of values in the of Minson and McDonald (1987) equation, (qm = 0.00795 DMD – 0.0014 – DMD expressed as a percentage). This showed that the estimated CH4 output of the sheep industry would increase by 6% (considering 100% adoption rate), as a consequence of increase in DMI by grazing sheep (Fig. 3). When Hegarty et al. (2010) simulated the effect of increasing DMD and DMI on daily CH4 production per head, the response was almost linear, although the rate of increase in CH4 production was lower for high DMD diets than for low to moderate DMD diets. However, this present simulation does not include DMD effects on average DWG in sheep, and, so, does not reflect the reality of the biological system where pasture and animals are interdependent.
Sensitivity of total methane production per year in New South Wales (NSW) to a 5% improvement in dry-matter digestibility of pastures across all seasons, on the basis of the National Inventory report calculations (compared with the baseline methane production (https://www.industry.gov.au/data-and-publications/national-greenhouse-accounts-2019/national-inventory-report-2019).
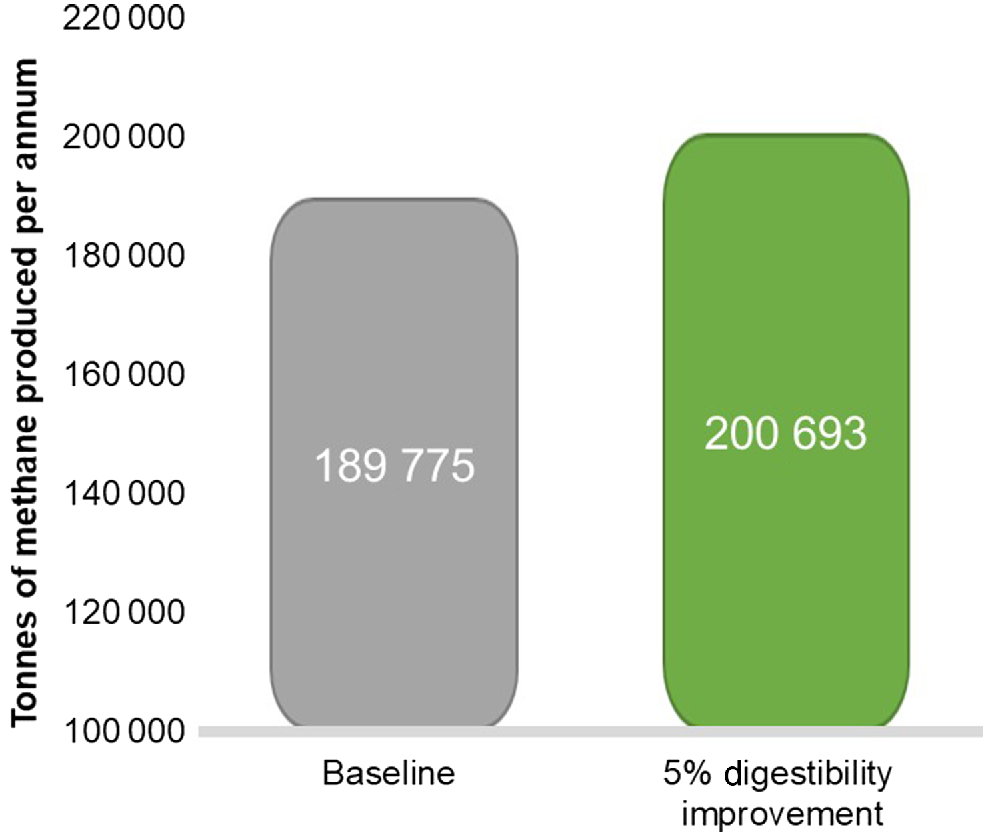
Pasture quality, DMI, animal productivity and daily CH4 production are inter-related and cannot be simplistically described (Hegarty et al. 2010). Hegarty et al. (2010) simulated the effect of a 30 kg Border–Leicester lamb offered an ad libitum roughage diet of increasing DMD on LWG, DMI, daily CH4 production, and emissions intensity. As DMI increased, daily CH4 production and LWG responded almost linearly. The CH4 efficiency of consumed energy (i.e. daily CH4 production per unit of metabolisable-energy intake; MEI) was highest for high-DMD diets, and for the same MEI, daily CH4 emissions will decrease with an increasing DMD because less DMI is required to achieve the same MEI. The implication of these responses is that increasing DMI, regardless of DMD, increases LWG and thus reduces emissions intensity of each kilogram of liveweight. However, at any given DMI, increased DMD will reduce emissions intensity (Hegarty et al. 2010).
However, in practice, improved digestibility may not lift feed intake, as a result of pasture availability or plant morphology and density. For example, in a tropical pasture grazed at 14 days of regrowth, compared with 28 days of regrowth, organic-matter (OM) digestibility improved to 0.67 from 0.70 respectively, but the consequent changes in plant morphology caused steer bite size, and thus OM intake, to decline from 4.81 to 4.22 kg OM/day (Boval et al. 2007). In a situation where DMD increases without an increase in DMI, the realised impact is likely to be no change in total CH4 produced (driven by the steady DMI), but likely to be accompanied by an increase in growth (driven by the increasing metabolisability of DM consumed); thus, the emissions intensity of the meat, wool or milk produced would decline, but the per animal emissions would not decrease. Therefore, the responsiveness of the set of equations used in the framework must be improved before the producer could benefit from carbon credit income from improving pasture digestibility. While this lack of responsiveness is not apparent in the National Inventory, reflecting its insensitivity, these system-effects are the basis for most progress in intensification of ruminant production. There must be a coming together of basal-feed change and animal number or management change, for a grazing system to maintain some sort of equilibrium and thus to be sustainable. This coming together is exemplified in the push to improve the basal feed and intensify animal agriculture, and is discussed below.
Including methane suppressing forages in grazing systems
No matter what grazing-based production system is in use, there is scope to change not only the general nutritional value of the forage, but to introduce specific CH4-suppressing forages. More than 200 000 plant secondary compounds, or phytochemicals, have been identified (Hartmann 2007), and some have anti-methanogenic proprieties, including tannins, essential oils, and saponins (Durmic et al. 2022). In many cases, these may possess anti-nutritional characteristics, and so the aim is to find the equilibrium between the beneficial CH4 abatement and the optimum nutrient utilisation. This balance is particularly complex to attain as the composition and quantity of phytochemicals varies widely within natural sources. Plants that have elevated levels of condensed tannins offer the greatest forage-based possibility of reducing enteric CH4 production while maintaining or increasing animal performance. These include Lotus spp., (Banik et al. 2013), Desmanthus (Suybeng et al. 2019) and the more tropically adapted Leucaena leucocephala and Gliricidia septum, browses that are all at commercial or near-commercial stages of availability. Desmanthus is suited to the warm non-frosting regions of NSW. Similarly, the tree legume L. leucocephala is also suited to these areas and can deliver commercially significant CH4 mitigation (Tomkins et al. 2019), but is not currently recommended in NSW due to its propensity to become a weed. While other forages have shown promise (e.g. Biserrula pelecinus, Eremophila glabra;Durmic et al. 2014), they are not close to commercial release.
One of the key advantages of leguminous CH4-active legumes is the additional dietary protein they supply, which can be seasonally important in drier regions such as northern and north-western NSW. Thus, the advantage of these CH4-inhibitory forages is they not only suppress emissions chemically, but can affect total diet digestibility and DMI, and thus have a feed-forward catalytic role in stimulating animal liveweight and, therefore, move the system to both lower emissions and higher productivity as long as the cost to establish and maintain these forages in vast farm lands is managed.
Supplementing CH4-supressing additives
Several feed supplements can be fed to ruminants and result in lower CH4 emissions [e.g. oils, Asparagopsis, nitrate, ionophores, bacteriocins, a wide array of phytochemicals, 3-nitrooxypropanol (3-NOP), etc.; Almeida and Hegarty 2021]. The adoption of feed strategies can contribute to Australia’s efforts to reduce GHG emissions as well as create opportunity to producers in earning Australian carbon credit units (ACCUs). In this regard, we simulated the CH4 mitigation potential within NSW by using two feed additives commercially available (phytochemicals and nitrate) and two novel feed additives in the process of entering the market (Asparagopsis and 3-NOP). Previously reported abatement potential of these feed additives was used as the basis of the state-wide CH4-mitigation quantification, considering the ruminant population in NSW (Fig. 4).
Monte Carlo-based simulation of total CH4 production per year in New South Wales (NSW) to a 10%, 30%, 60%, and 90% adoption rate of CH4-supressing feed additives, on the basis of the National Inventory report calculations (compared with the baseline CH4 production (https://www.industry.gov.au/data-and-publications/national-greenhouse-accounts-2019/national-inventory-report-2019). Monte Carlo techniques (Fan et al. 2002) were used to estimate the uncertainty of CH4 output, considering the variation of the CH4 abatement reported by Almeida et al. (2021) and assuming a fixed ruminant population.
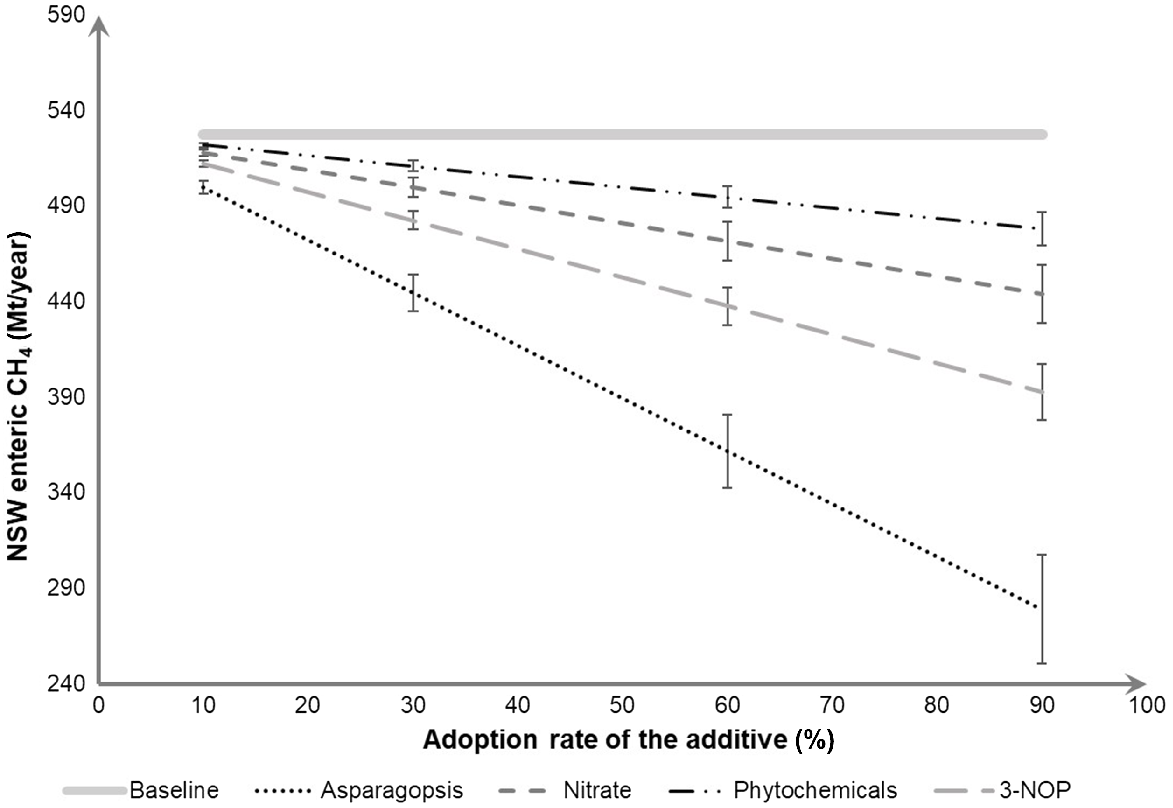
A previously published meta-analysis by Almeida et al. (2021) that quantified the mitigation potential of several methane suppressing additives clustered tannins, essential oils, and saponins into phytochemicals comprising in 33 different studies (17 with sheep, 7 with dairy cattle and 9 with beef cattle). We tested whether the CH4 mitigation differed among sheep, dairy and beef cattle and our results showed no evidence of such difference [P = 0.59; this analysis was performed using the MIXED procedure of SAS, ver. 9.4, SAS/STAT, SAS Institute Inc., Cary, NC, USA, considering study as a random effect; please refer to Almeida et al. (2021) for further details]; thus, we used the CH4 reduction reported by Almeida et al. (2021) to perform the simulations (i.e. 11.0 ± 1.84%). Depending on the adoption rate, phytochemicals can mitigate up to 9.30% of enteric-CH4 emissions in NSW, according to our simulation. To simulate the abatement potential of nitrate, we used 24 different studies (5 with sheep, 6 with dairy cattle and 13 with beef cattle). We found that the CH4 mitigation achieved when feeding nitrate to sheep was greater than that observed in cattle (beef or dairy) (26.8 ± 4.96% vs 12.4 ± 4.23%; P = 0.03). Thus, we simulated the State’s CH4 production considering 12.4% reduction on CH4 output when feeding nitrate to cattle, and 26.8% CH4 reduction in sheep. This difference might be due to the nature of the basal diets used in different ruminant species. Our simulation showed that using adoption rates from 10% to 90%, the methane abatement increased from 1.76% to 15.8% (Fig. 4).
Although feed additives such as nitrate and phytochemicals do not provide the greatest methane abatement of the feed additives, they are readily available supplements at a low cost. Additionally, nitrate has a co-benefit as a dietary non-protein nitrogen source and has the advantage of an existing emission reduction fund (ERF) method supplementing grazing cattle with nitrate, enabling producers to access the ACCUs market. One may note that upper limits of nitrate supplementation must be respected to avoid nitrate toxicity. Therefore, these feed additives potentially offer greater likelihood of adoption.
The 3-NOP effect on CH4 production was simulated using information of 14 different studies (none with sheep, seven with dairy cattle and seven with beef cattle). We have found evidence that CH4 abatement differs between dairy and beef cattle (P = 0.71); thus, we used the CH4 reduction reported by Almeida et al. (2021) for all ruminant animals to perform the simulations (i.e. 29.0 ± 3.11%). Our results showed that the potential CH4 abatement achieved when feeding 3-NOP to ruminants varied from 2.83% to 25.5%, considering adoption rates ranging from 10% to 90%. Only four studies using Asparagopsis were available (one with sheep, one with dairy cattle and two with beef cattle); thus, we considered its CH4 mitigation in ruminants in general, as published by Almeida et al. (2021) (i.e. CH4 mitigation of 52.3 ± 6.03%). Feeding Asparagopsis to ruminants showed the greatest CH4 mitigation, potentially reducing State’s CH4 output from 5.23% up to 47.1%, depending on the adoption rate.
One might note that adoption is a determinant of magnitude in a State’s CH4 mitigation. In turn, adoption is affected by the price of the product compared with the return to the producer, which might be in ACCUs and/or productivity gains (co-benefits). The CH4 quantification potential of a combination of feed additives is a gap yet to be explored in future studies.
Intensification of livestock systems
The impact of intensification on enteric emissions has been regularly displayed in the decline in milk emission intensity that has been reported globally (Niu et al. 2018; Naranjo et al. 2020). As with improved reproductive performance, intensifying production creates opportunity to raise more stock on the same land area or keep the same number of animals, but on a reduced area of land, while keeping product output unchanged. With ‘systems thinking’ this gives the land manager an opportunity to take some land out of grazing should they wish, perhaps for reforestation or carbon-sequestration activities; this choice will depend on balance of environmental and economic priorities for the livestock manager. While the long-term impact of this has been modelled for sheep enterprises in NSW (Alcock et al. 2015), further system modelling on the merit of land-use change associated with increased intensity, animal efficiency and reproductive performance is required especially for beef. Although difficult to quantify, trees planting can also be integrated into livestock production systems in ways that it may result in increased production (i.e. through providing shade and shelter) and other co-benefits (i.e. improved biodiversity and soil and water health).
Supplementation of grazing livestock with energy concentrates (i.e. grain) can be predicted to increase CH4 production per day, in line with the responses to increasing MEI described by Hegarty et al. (2010), above. However, the effects of partial substitution of supplement for basal forage (Dove 2002) mean that total DM and OM intake will increase but forage intake will decrease, with only marginal effect on total daily CH4 production or emissions intensity (Boadi et al. 2002). Improving digestible OM and ME intake by increasing pasture DMD may have an influence on CH4 emissions, although this has not yet been tested. The current methods for predicting CH4 emissions of grazing sheep account only for expected pasture digestibility, not the whole diet including supplements. However, improved metabolisability of the whole diet will increase the LWG function, and thus the emissions estimate, by the national inventory methods. The national inventory methods may need to be adjusted to account for grain supplementation if the body of evidence can be strengthened, but this evaluation should extend beyond inventory to also include life-cycle analysis of emissions.
What is clear is that managing the animals through grazing, genetic selection, culling and early breeding decisions, together with managing the basal feed in its composition, quality and potentially CH4-suppressing swards, have great potential to mitigate emissions both in absolute terms (kg CH4/day) and in emission intensity of the livestock products. The enterprise-scale outcome is subject to matching decisions on total livestock kept and land area used. The efficacy of combinations of subsets of this suite of decisions is evident in assessment in the dairy industry (Beukes et al. 2010), sheep industry (Alcock et al. 2015) and in explaining differences in greenhouse outputs and profitability of diverse beef enterprises (Harrison et al. 2016). These positive responses in emissions to animal and feed management are seen across globally diverse feeding/grazing systems (Gerssen-Gondelach et al. 2017), even when the assessment is made on a basis of life-cycle analysis. This gives confidence that a consistent positive set of mitigation outcomes can be achieved in grazing systems across NSW.
Simulations of NSW impacts of improved reproductive efficiency
Lean and Moate (2021) estimated that the potential scope to make improvements to reproductive efficiency in southern Australian beef production was moderate (compared with high in northern production systems). Simulations were run to assess the effect of an increase in reproductive rate on emissions and emissions intensity. Baseline NGGI values of average NSW beef cow (85%) and sheep (125%) fertility were assumed. Simulations examined the emission impacts of improving reproductive rates of sheep and beef cattle by 5% and 10%. The effects of raising the reproductive rate of breeders on NSW emissions, on the basis of the state ruminant population (ABS 2018), were modelled considering adoption rates of 50%, 60%, 70% and 80%.
The simulations were run reflecting a constant output of saleable animals, meaning that as reproductive rate increased, the number of breeding females in the state decreased. Specifically, improving fertility by 5% was predicted to result in a reduction in the State current emissions by 1.56%, 1.87%, 2.18% and 2.50% for 50%, 60%, 70% and 80% adoption rates respectively (Fig. 4). Similarly, with improving fertility by 10%, the expected CH4 abatement would be 2.97%, 3.56%, 4.16%, and 4.75% of current emissions for 50%, 60%, 70% and 80% adoption rates respectively (Fig. 5).
Sensitivity of total methane production from ruminants per year in New South Wales (NSW) to 5% and 10% fertility improvement in sheep and beef cattle, compared with the baseline methane production.
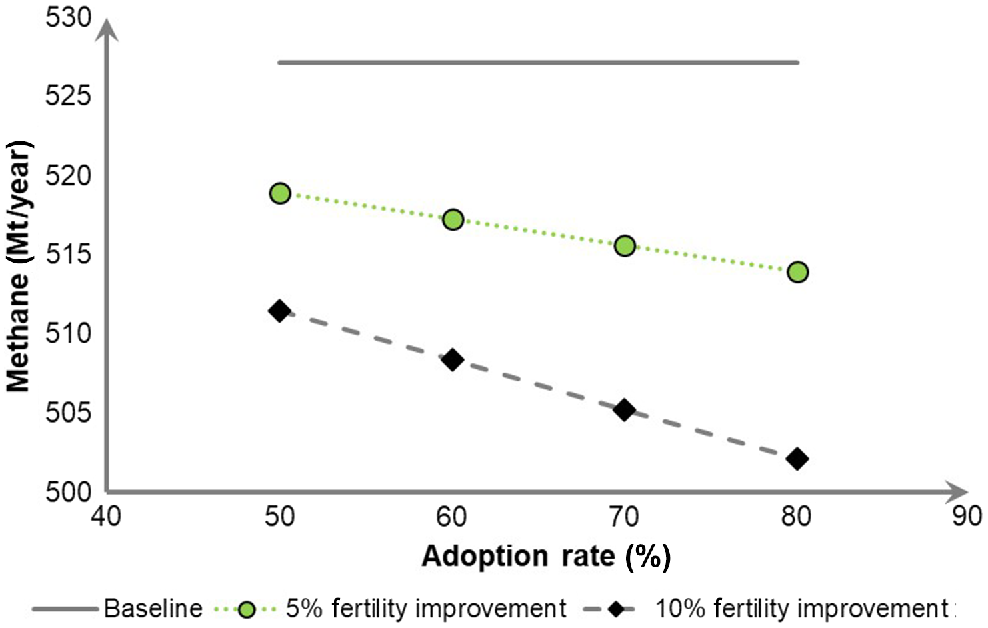
In the present simulation, the main driver in CH4 abatement (Mt/year) was the reduction in breeding females, while the product output was kept the same. Using a different approach, in a case study modelling cattle properties in dry inland regions, an improved reproduction gave a 22–28% decline in GHG emissions intensity (t CO2-e/t liveweight sold), largely due to higher weaning rates, that would likely increase enterprise CH4 production due to increased number of animals (Cullen et al. 2016). This improved farm profitability by up to AUD$62 000 due to more liveweight sold from the same number of breeding females.
Nutritional and other strategies to reduce CH4 emissions are unlikely to be adopted unless profitability can be maintained or improved, even when government or other private sector schemes offer financial reward (such as the Emissions Reduction Fund or the Carbon Farming Initiative; Alcock et al. 2015). Thus, nutritional strategies that result in proven productivity improvements may offer greater likelihood of adoption than for dietary additives that have modest or neutral productivity co-benefits, but greater CH4 yield abatement.
Reducing ewe joining age is a consistently profitable management strategy, and so offers good likelihood of adoption (Alcock et al. 2015) if prioritised in policies. Managing beef breeders to a high plane of nutrition during pregnancy and lactation is also consistently more profitable than is poor nutrition at this time (Alford et al. 2009).
Simulations of the stocking rate, gross margin and enterprise CH4-emission effects of pasture improvement in a 100 ha Merino wool enterprise showed that pasture improvement from a native pasture baseline allows for increased pasture yield/ha, and consequent increased stocking rate and decreased emissions intensity on the improved pasture. Thus enterprise CH4 emissions can be reduced by taking advantage of the increased productivity efficiency and reducing flock size and grazing a smaller area of improved pasture only (24 ha), while maintaining no change in enterprise gross margin. However, the likelihood of farm managers adopting such a strategy is small. Far more likely is that producers will wish to realise the productivity dividend of pasture improvement. Accordingly, Alcock and Hegarty (2006) simulated the gross margin impacts of partial pasture improvement (43 ha), with subsequent increase in stocking rate, productivity and emissions intensity, so that total enterprise emissions were equal to those from grazing the 100 ha of unimproved pasture with a smaller, less efficient flock. The result was an almost doubling of gross margin, for the same enterprise CH4 emissions, and less than half of the land area grazed. These simulations do not take into consideration a price on carbon or value of ungrazed land for carbon sequestration offset payments, but show that with the right balance of incentives, a win–win outcome is possible for farm income and for CH4 emissions, which will be necessary to achieve widespread adoption.
Conclusions
Changes to on-farm nutritional management of livestock and feed base provide multiple and interacting opportunities to reduce total enteric emissions, emission intensity, and either increase productivity or change the land area used and number of stock required, subject to producer priorities and financial incentives.
The systems-scale nutritional strategies to reduce methane emissions in NSW breeder herds and flocks described in this review hinge on two approaches, i.e. either increasing nutritional supply by improvements to the basal feed, or reducing nutritional demand, through reducing the contribution of non-productive females or by reducing the maintenance requirements of females to be productive. Improvements to nutrient supply, by basal-feed improvements, increased digestibility, liveweight and rate of LWG, and are assumed to increase intake, and hence actually contribute to increased CH4 emissions per animal and per enterprise, without commensurate decreases in herd or flock numbers, although they do improve emissions intensity of animal products. Management strategies targeted to reduce the proportion of the breeder herd or flock not contributing to production (reducing age at first joining, increasing reconception rate and weaned weight and rate) will result in increased emissions per animal and per enterprise, due to the increased demand for feed energy from milk production, although emissions intensity of weaners turned off will be reduced. In contrast, management strategies that reduce nutritional maintenance requirements, such as selection for smaller females with lower maintenance requirement in self-replacing herds and flocks, can reduce both emissions intensity and total enterprise emissions, without changes to animal numbers.
In recent years, prices for animal protein have become uncoupled from supply as global demand has increased to a point where export markets seem to have an almost infinite capacity to absorb Australian production in excess of domestic demand (MLA 2019). As a result, it is likely that improvements in productivity or productive efficiency (and emissions intensity) will not result in market signals to producers to reduce flock and herd sizes. Instead, producers will be incentivised to take advantage of the efficiency dividend and increase herd size, thus increasing total emissions at enterprise and regional scale. To achieve a reduction in grazing livestock contributions to State and National Inventories of GHG emissions by production-system changes, producers will need competitive economic incentives to intensify production and then re-allocate land to carbon-sequestration uses, thus reducing gross and net enterprise emissions and inventory. Further investigation is required of combinations of different strategies for CH4 mitigation by using a systemic approach, to inform policy recommendations.
References
ABS (2018) ‘Australian Bureau of Statistics.’ 7121.0 - Agricultural Commodities Australia. (Canberra, ACT, Australia). Available at https://www.abs.gov.au/ausstats/abs@.nsf/mf/7121.0 [Accessed October 2020]
Alcock D, Hegarty RS (2006) Effects of pasture improvement on productivity, gross margin and methane emissions of a grazing sheep enterprise. International Congress Series 1293, 103-106.
| Crossref | Google Scholar |
Alcock DJ, Hegarty RS (2011) Potential effects of animal management and genetic improvement on enteric methane emissions, emissions intensity and productivity of sheep enterprises at Cowra, Australia. Animal Feed Science and Technology 166–167, 749-760.
| Crossref | Google Scholar |
Alcock DJ, Harrison MT, Rawnsley RP, Eckard RJ (2015) Can animal genetics and flock management be used to reduce greenhouse gas emissions but also maintain productivity of wool-producing enterprises? Agricultural Systems 132, 25-34.
| Crossref | Google Scholar |
Alford AR, Cafe LM, Greenwood PL, Griffith GR (2009) Economic effects of nutritional constraints early in life of cattle. Animal Production Science 49, 479-492.
| Crossref | Google Scholar |
Almeida AK, Hegarty RS, Cowie A (2021) Meta-analysis quantifying the potential of dietary additives and rumen modifiers for methane mitigation in ruminant production systems. Animal Nutrition 7, 1219-1230.
| Crossref | Google Scholar | PubMed |
Arnold GW, Dudzinski ML (1967) Studies on the diet of grazing animal. III. The effect of pasture species and pasture structure on the herbage intake of sheep. Australian Journal of Agricultural Research 18, 657-666.
| Crossref | Google Scholar |
Australian Government (2020) Australian greenhouse emissions information system. Available at https://ageis-activity-table-1990-2021-agriculture-livestock-national.xlsx (2020) [Accessed October 2020]
Banchero GE, Stefanova K, Lindsay DR, Quintans G, Baldi F, Milton JTB, Martin GB (2021) Ovulation and ovulation rate in ewes under grazing conditions: factors affecting the response to short-term supplementation. Animal 15(2), 100100.
| Crossref | Google Scholar | PubMed |
Banik BK, Durmic Z, Erskine W, Ghamkhar K, Revell C (2013) In vitro ruminal fermentation characteristics and methane production differ in selected key pasture species in Australia. Crop and Pasture Science 64(9), 935-942.
| Crossref | Google Scholar |
Beukes PC, Gregorini P, Romera AJ, Levy G, Waghorn GC (2010) Improving production efficiency as a strategy to mitigate greenhouse gas emissions on pastoral dairy farms in New Zealand. Agriculture, Ecosystems & Environment 136(3–4), 358-365.
| Crossref | Google Scholar |
Boadi DA, Wittenberg KM, McCaughey W (2002) Effects of grain supplementation on methane production of grazing steers using the sulphur (SF6) tracer gas technique. Canadian Journal of Animal Science 82, 151-157.
| Crossref | Google Scholar |
Boval M, Archimède H, Cruz P, Duru M (2007) Intake and digestibility in heifers grazing a Dichanthium spp. dominated pasture, at 14 and 28 days of regrowth. Animal Feed Science and Technology 134, 18-31.
| Crossref | Google Scholar |
Bruinje TC, Rosadiuk JP, Moslemipur F, Sauerwein H, Steele MA, Ambrose DJ (2021) Differing planes of pre- and postweaning phase nutrition in Holstein heifers: II. Effects on circulating leptin, luteinizing hormone, and age at puberty. Journal of Dairy Science 104, 1153-1163.
| Crossref | Google Scholar | PubMed |
Capper JL, Cady RA, Bauman DE (2009) The environmental impact of dairy production: 1944 compared with 2007. Journal of Animal Science 87(6), 2160-2167.
| Crossref | Google Scholar | PubMed |
Charmley E, Williams SRO, Moate PJ, Hegarty RS, Herd RM, Oddy VH, Reyenga P, Staunton KM, Anderson A, Hannah MC (2016) A universal equation to predict methane production of forage-fed cattle in Australia. Animal Production Science 56, 169-180.
| Crossref | Google Scholar |
Christie KM, Harrison MT, Trevaskis LM, Rawnsley RP, Eckard RJ (2016) Modelling enteric methane abatement from earlier mating of dairy heifers in subtropical Australia by improving diet quality. Animal Production Science 56(3), 565-573.
| Crossref | Google Scholar |
Cottle DJ, Nolan JV, Wiedemann SG (2011) Ruminant enteric methane mitigation: a review. Animal Production Science 51, 491-514.
| Crossref | Google Scholar |
Cullen BR, Eckard RJ, Timms M, Phelps DG (2016) The effect of earlier mating and improving fertility on greenhouse gas emissions intensity of beef production in northern Australian herds. The Rangeland Journal 38, 283-290.
| Crossref | Google Scholar |
Doran-Browne N, Behrendt R, Kingwell R, Eckard R (2015) Modelling the potential of birdsfoot trefoil (Lotus corniculatus) to reduce methane emissions and increase production on wool and prime lamb farm enterprises. Animal Production Science 55(9), 1097-1105.
| Crossref | Google Scholar |
Durmic Z, Moate PJ, Eckard R, Revell DK, Williams R, Vercoe PE (2014) In vitro screening of selected feed additives, plant essential oils and plant extracts for rumen methane mitigation. Journal of the Science of Food and Agriculture 94(6), 1191-1196.
| Crossref | Google Scholar | PubMed |
Durmic Z, Black JL, Martin GB, Vercoe PE (2022) Harnessing plant bioactivity for enteric methane mitigation in Australia. Animal Production Science 62, 1160-1172.
| Crossref | Google Scholar |
D’Occhio MJ, Baruselli PS, Campanile G (2019) Influence of nutrition, body condition, and metabolic status on reproduction in female beef cattle: a review. Theriogenology 125, 277-284.
| Crossref | Google Scholar | PubMed |
Eckard RJ, Grainger C, de Klein CAM (2010) Options for the abatement of methane and nitrous oxide from ruminant production: a review. Livestock Science 130(1–3), 47-56.
| Crossref | Google Scholar |
Ehhalt D, Prather MJ, Dentener F, Derwent RG, Dlugokencky E, Holland E, Isaksen ISA, Katima J, Kirchhoff V, Matson P, Midgley P, Wang M (2001) Atmospheric chemistry and greenhouse gases. In ‘Climate change 2001; Working Group 1: the scientific basis’. (Eds JT Houghton, Y Ding, DJ Griggs, M Noguer, PJ van der Linden, D Xiaosu) pp. 241–287. (Cambridge University Press)
Farrell LJ, Tozer PR, Kenyon PR, Ramilan T, Cranston LM (2019) The effect of ewe wastage in New Zealand sheep and beef farms on flock productivity and farm profitability. Agricultural Systems 174, 125-132.
| Crossref | Google Scholar |
Gerssen-Gondelach SJ, Lauwerijssen RBG, Havlík P, Herrero M, Valin H, Faaij APC, Wicke B (2017) Intensification pathways for beef and dairy cattle production systems: impacts on GHG emissions, land occupation and land use change. Agriculture, Ecosystems & Environment 240, 135-147.
| Crossref | Google Scholar |
Grainger C, Beauchemin KA (2011) Can enteric methane emissions from ruminants be lowered without lowering their production? Animal Feed Science and Technology 166–167, 308-320.
| Crossref | Google Scholar |
Harrison MT, Jackson T, Cullen BR, Rawnsley RP, Ho C, Cummins L, Eckard RJ (2014) Increasing ewe genetic fecundity improves whole-farm production and reduces greenhouse gas emissions intensities: 1. Sheep production and emissions intensities. Agricultural Systems 131, 23-33.
| Crossref | Google Scholar |
Harrison MT, Cullen BR, Tomkins NW, McSweeney C, Cohn P, Eckard RJ (2016) The concordance between greenhouse gas emissions, livestock production and profitability of extensive beef farming systems. Animal Production Science 56(3), 370-384.
| Crossref | Google Scholar |
Hartmann T (2007) From waste products to ecochemicals: fifty years research of plant secondary metabolism. Phytochemistry 68, 2831-2846.
| Crossref | Google Scholar | PubMed |
Hegarty RS, Alcock D, Robinson DL, Goopy JP, Vercoe PE (2010) Nutritional and flock management options to reduce methane output and methane per unit product from sheep enterprises. Animal Production Science 50, 1026.
| Crossref | Google Scholar |
Herrero M, Henderson B, Havlík P, Thornton PK, Conant RT, Smith P, Wirsenius S, Hristov AN, Gerber P, Gill M, Butterbach-Bahl K, Valin H, Garnett T, Stehfest E (2016) Greenhouse gas mitigation potentials in the livestock sector. Nature Climate Change 6, 452-461.
| Crossref | Google Scholar |
Howden SM, White DH, Mckeon GM, Scanlan JC, Carter JO (1994) Methods for exploring management options to reduce greenhouse gas emissions from tropical grazing systems. Climatic Change 27, 49-70.
| Crossref | Google Scholar |
Hristov AN, Oh J, Firkins JL, Dijkstra J, Kebreab E, Waghorn G, Makkar HPS, Adesogan AT, Yang W, Lee C, Gerber PJ, Henderson B, Tricarico JM (2013) Mitigation of methane and nitrous oxide emissions from animal operations: I. A review of enteric methane mitigation options. Journal of Animal Science 91, 5045-5069.
| Crossref | Google Scholar | PubMed |
Le Cozler Y, Lollivier V, Lacasse P, Disenhaus C (2008) Rearing strategy and optimizing first-calving targets in dairy heifers: a review. Animal 2, 1393-1404.
| Crossref | Google Scholar | PubMed |
Lean IJ, Moate PJ (2021) Cattle, climate and complexity: food security, quality and sustainability of the Australian cattle industries. Australian Veterinary Journal 99, 293-308.
| Crossref | Google Scholar | PubMed |
Lehmann JO, Mogensen L, Kristensen T (2014) Extended lactations may improve cow health, productivity and reduce greenhouse gas emissions from organic dairy production. Organic Agriculture 4(4), 295-299.
| Crossref | Google Scholar |
Martin C, Morgavi DP, Doreau M (2010) Methane mitigation in ruminants: from microbe to the farm scale. Animal 4, 351-365.
| Crossref | Google Scholar | PubMed |
Michell PJ (1973) Digestibility and voluntary intake measurements on regrowths of six Tasmanian pasture species. Australian Journal of Experimental Agriculture 13, 158-164.
| Crossref | Google Scholar |
Minson DJ, McDonald CK (1987) Estimating forage intake from the growth of beef cattle. Tropical Grasslands 21, 116-122.
| Google Scholar |
MLA (2019) Industry projections 2019 Australian cattle. Meat & Livestock Australia. Available at https://www.mla.com.au/globalassets/mla-corporate/prices--markets/documents/trends--analysis/cattle-projections/mla-australian-cattle-industry-projections-2019.pdf
Naranjo A, Johnson A, Rossow H, Kebreab E (2020) Greenhouse gas, water, and land footprint per unit of production of the California dairy industry over 50 years. Journal of Dairy Science 103, 3760-3773.
| Crossref | Google Scholar | PubMed |
Newton JE, Brown DJ, Dominik S, van der Werf JHJ (2017) Impact of young ewe fertility rate on risk and genetic gain in sheep-breeding programs using genomic selection. Animal Production Science 57, 1653-1664.
| Crossref | Google Scholar |
Niu M, Kebreab E, Hristov AN, Oh J, Arndt C, Bannink A, Bayat AR, Brito AF, Boland T, Casper D, Crompton LA, Dijkstra J, Eugene MA, Garnsworthy PC, Haque MN, Hellwing ALF, Huhtanen P, Kreuzer M, Kuhla B, Lund P, Madsen J, Martin C, McClelland SC, McGee M, Moate PJ, Muetzel S, Munoz C, O’Kiely P, Peiren N, Reynolds CK, Schwarm A, Shingfield KJ, Storlien TM, Weisbjerg MR, Yanez-Ruiz DR, Yu Z (2018) Prediction of enteric methane production, yield, and intensity in dairy cattle using an intercontinental database. Global Change Biology 24, 3368-3389.
| Crossref | Google Scholar | PubMed |
Nkrumah JD, Okine EK, Mathison GW, Schmid K, Li C, Basarab JA, Price MA, Wang Z, Moore SS (2006) Relationships of feedlot feed efficiency, performance, and feeding behavior with metabolic rate, methane production, and energy partitioning in beef cattle. Journal of Animal Science 84(1), 145-153.
| Crossref | Google Scholar | PubMed |
Nunez-Dominguez R, Cundiff LV, Dickerson GE, Gregory KE, Koch RM (1991) Lifetime production of beef heifers calving first at two vs three years of age. Journal of Animal Science 69(9), 3467-3479.
| Google Scholar | PubMed |
Pinares-Patiño CS, Waghorn GC, Hegarty RS, Hoskin SO (2009) Effects of intensification of pastoral farming on greenhouse gas emissions in New Zealand. New Zealand Veterinary Journal 57(5), 252-261.
| Crossref | Google Scholar | PubMed |
Pitchford WS, Accioly JM, Banks RG, Barnes AL, Barwick SA, Copping KJ, Deland MPB, Donoghue KA, Edwards N, Hebart ML, Herd RM, Jones FM, Laurence M, Lee SJ, McKiernan WA, Parnell PF, Speijers EJ, Tudor GD, Graham JF (2018) Genesis, design and methods of the Beef CRC Maternal Productivity Project. Animal Production Science 58, 20-32.
| Crossref | Google Scholar |
Schillo KK, Hall JB, Hileman SM (1992) Effects of nutrition and season on the onset of puberty in the beef heifer. Journal of Animal Science 70, 3994-4005.
| Crossref | Google Scholar | PubMed |
Short RE, Bellows RA (1971) Relationships among weight gains, age at puberty and reproductive performance in heifers. Journal of Animal Science 32, 127-131.
| Crossref | Google Scholar |
Stobbs TH (1973) The effect of plant structure on the intake of tropical pastures. I. Variation in the bite size of grazing cattle. Australian Journal of Agricultural Research 24, 809-819.
| Crossref | Google Scholar |
Suybeng B, Charmley E, Gardiner CP, Malau-Aduli BS, Malau-Aduli AEO (2019) Methane emissions and the use of desmanthus in beef cattle production in Northern Australia. Animals 9(8), 542.
| Crossref | Google Scholar | PubMed |
Tocker J, Behrendt R, Raeside M, Malcolm B (2020) The impact of ewe lamb mating and different feeding strategies over summer–autumn on profit and risk: a case study in south-west Victoria. Animal Production Science 61, 1137-1150.
| Crossref | Google Scholar |
Tomkins N, Harrison M, McSweeney CS, Denman S, Charmley E, Lambrides CJ, Dalal R (2019) Greenhouse gas implications of leucaena-based pastures. Can we develop an emissions reduction methodology for the beef industry? Tropical Grasslands-Forrajes Tropicales 7(4), 267-272.
| Crossref | Google Scholar |
Walmsley BJ, Lee SJ, Parnell PF, Pitchford WS (2018) A review of factors influencing key biological components of maternal productivity in temperate beef cattle. Animal Production Science 58, 1-19.
| Crossref | Google Scholar |
White DH, Bowman PJ, Morley FHW, McManus WR, Filan SJ (1983) A simulation model of a breeding ewe flock. Agricultural Systems 10, 149-189.
| Crossref | Google Scholar |