The initiation of smouldering peat fire by a glowing firebrand
Shaorun Lin


A
B
Abstract
Wildfires represent a significant threat to peatlands globally, but whether peat fires can be initiated by a lofted firebrand is still unknown.
We investigated the ignition threshold of peat fires by a glowing firebrand through laboratory-scale experiments.
The oven-dried weight (ODW) moisture content (MC) of peat samples varied from 5% ODW to 100% ODW, and external wind (ν) with velocities up to 1 m/s was provided in a wind tunnel.
When MC < 35%, ignition is always achieved, regardless of wind velocity. However, if MC is between 35 and 85%, an external wind (increasing with peat moisture) is required to increase the reaction rate of the firebrand and thus heating to the peat sample. Further increasing the MC to be higher than 85%, no ignition could be achieved by a single laboratory firebrand. Finally, derived from the experimental results, a 90% ignition probability curve was produced by a logistic regression model.
This work indicates the importance of maintaining a high moisture content of peat to prevent ignition by firebrands and helps us better understand the progression of large peat fires.
Keywords: ember, firebreak, ignition, peatland, smouldering, spotting ignition, wildfire, wind.
Introduction
Fire is a key disturbance to peatlands globally, driven primarily by smouldering, leading to one of the most persistent types of fire phenomena on Earth (Rein 2013; Lin et al. 2021a). Smouldering is a heterogeneous reaction sustained by exothermic oxidation in the solid phase, often characterised as a sluggish, flameless, and low-temperature combustion process (Ohlemiller 1986; Lin et al. 2021c). Although peatlands only cover 2–3% of the global land surface, they store over 25% of terrestrial organic carbon, which is approximately equal to that stored in the atmosphere or living plants (Lehmann and Joseph 2015; Kohlenberg et al. 2018). Once ignited, peat fires may burn for weeks or even months, regardless of natural precipitations, weather changes, or fire-fighting attempts (Lin et al. 2020). As a result, global estimates for carbon emissions from peatland fires are equivalent to 15% of man-made emissions from burning fossil fuels, which is comparable to the anthropogenic carbon emissions from the entire European Union (Poulter et al. 2006; Rein and Huang 2021).
Smouldering peatland fires were brought to the forefront of scientific debate following the 1997 extreme haze event in south-east Asia from burning peatlands during a particularly strong El Nino season (Page et al. 2002). Following this event, many research studies have targeted understanding the characteristics of smouldering peat fires, including combustion chemistry (Huang and Rein 2016), ignition (Lin et al. 2019; Cui et al. 2022; Santoso et al. 2022), fire spread (Prat et al. 2015; Yang and Chen 2018; Huang and Rein 2019; Qin et al. 2022a, 2022b; Zhang et al. 2024), emissions (Hu et al. 2018; Kohlenberg et al. 2018; Lin et al. 2021b), detection (Atwood et al. 2016; Rein et al. 2017), and suppression (Ramadhan et al. 2017; Lin and Huang 2020; Lin et al. 2020; Santoso et al. 2021; Mulyasih et al. 2022). A recent review paper provided a comprehensive overview of smouldering wildfires, together with their associated environmental and health issues, as well as the challenges in prevention and mitigation (Rein and Huang 2021). However, of the studies addressing ignition thresholds of peat, the peat sample was either heated using a conical heater (uniform radiation) (Lin et al. 2019) or a linear electrical coil heater (conduction) (Frandsen 1987; Lin et al. 2021b), which differs in configuration and mechanisms from spotting ignition by firebrands.
Firebrands are generated from burning wildland fuels (e.g. grasses, shrubs, trees) and wooden structures (e.g. structural members, shakes, shingles) (Manzello et al. 2020). These combustible fragments are produced when burning debris loses its structural integrity and breaks into smaller pieces. Drag forces from surrounding airflows cause fracture as materials degrade, resulting in smouldering or flaming fragments which are lofted or blown long distances by external winds or buoyant fire-induced plumes (Manzello et al. 2007a). These firebrands then potentially ignite structures or vegetative fuels far ahead of the fire front (Manzello et al. 2006; Ellis 2015; Fernandez-Pello et al. 2015; Wang et al. 2017; Urban et al. 2018, 2019). Spotting ignition by burning firebrands is a significant fire spread pathway both in wildlands and at the wildland-urban interface, contributing to significant loss of life and property (Manzello et al. 2008; Caton et al. 2017; Wang et al. 2017). Due to its critical hazard, many research efforts have been taken to understand the mechanisms of firebrands, including generation (Manzello et al. 2007a; Suzuki and Manzello 2016; Thomas et al. 2017; Caton-Kerr et al. 2019; Ju et al. 2023), lofting (Tohidi and Kaye 2017), burning (Manzello et al. 2007b; Song et al. 2017), transport (Wadhwani et al. 2022), deposition (De Beer et al. 2023), and ignition of other fuels (Yin et al. 2014; Manzello et al. 2020; Tao et al. 2021; Zhu and Urban 2023). For example, Urban et al. (2019) investigated the ignition of a moist fuel bed (sawdust) by glowing firebrands of different sizes and found a minimum firebrand size of 3.17 mm in diameter and maximum fuel moisture content of 40% (wet-mass base) to achieve smouldering ignition. Salehizadeh et al. (2021) deposited firebrand piles on the dense woody fuels and found that ignition is sensitive to the fuel density and flaming ignition was additionally dependent on the wind speed. Richter et al. (2022) also reported that the propensity for ignition increases with wind speed, and a crevice geometry also significantly increases the possibility of ignition.
In south-east Asia, land clearance activities such as slash and burn practices by local communities are the primary contributors to peatland fire initiation and spread to forest reserves (Rein 2013). To limit fire spread, the construction of a firebreak is often employed to prevent fires from escaping existing burning areas (Lin et al. 2021a). An example of such a firebreak, constructed in Dungau, Beaufort, Malaysia by the local government in 2018 is shown in Fig. 1a. The firebreak here is designed like a water canal to effectively prevent flame and smouldering fires from spreading across the break. However, the distance may not be enough to prevent propagation via spotting ignition by lofted or blown firebrands that can travel for hundreds of metres. When a flaming surface fire is ignited on the peatland, the generated firebrands may fly over the firebreak and land on unburnt peatland to trigger smouldering peat fires or even potentially flaming fires (Fig. 1b and c). The ability of a firebrand to ignite the peat layer; however, has not been studied and confirmed yet.
(a) A peatland firebreak at Dungau, Beaufort, Malaysia constructed in 2018 (Credit: Sabah Forestry Department). (b) An ineffective firebreak during a Beaufort peatland fire, most likely because of spotting ignition by firebrands (Credit: Sabah Forestry Department), and (c) a schematic diagram showing how firebrands could fly over a firebreak and land on peatland to ignite a fire.
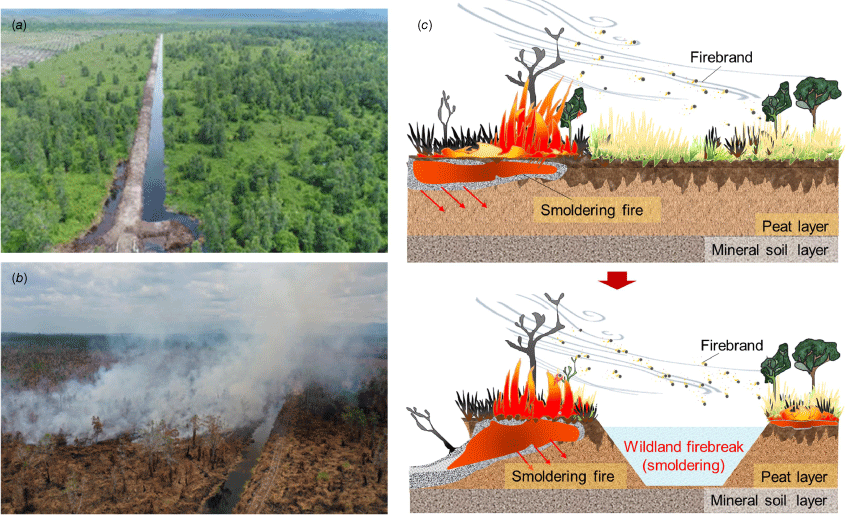
In this work, we explore the possibility of peat fire initiation by a smouldering (glowing) firebrand through laboratory-scale experiments in a wind tunnel. The oven-dried weight (ODW) moisture content (MC) of peat samples is varied from 5% ODW to 100% ODW and external winds with velocities (ν) up to 1 m/s are provided over the fuel surface. Simulated firebrands made from cut cylinders of commercially available poplar dowels are generated and deposited on the peat sample. A logistic regression is performed to quantify the ignition probability. This work helps understand the spread of peatland fires and re-think the firefighting strategies in peatland.
Materials and methods
Experiments were performed in a small-scale wind tunnel (Fig. 2a) that has been widely used in previous studies of smouldering combustion (Urban et al. 2019; Cobian-Iñiguez et al. 2022). The dimensions of the test section of the wind tunnel were 55 cm (L) × 13 cm (W) × 8 cm (H), where a lateral side was made of transparent glass to facilitate recording and observation of the burning phenomena inside the wind tunnel. A bed of peat sample (13 cm (L) × 8 cm (W) × 4 cm (H)) was filled in a reactor made of 1-cm ceramic insulation boards, and it was mounted horizontally along the floor of the tunnel test section (Fig. 2a). The top of the test section was open so that glowing firebrands could be dropped onto the targeted fuel.
(a) Schematic diagram of the experimental setup, and photos of (b) organic peat soil and (c) wooden dowel rods used to produce firebrands.
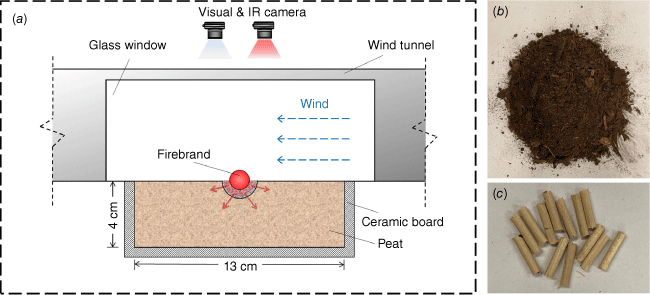
The peat soil tested in these experiments was commercial peat without additives (Fig. 2b) from the United States, made of ~100% organic all-natural moss peat with a uniform density, particle size, and organic content; thus, ensuring decent repeatability of fire experiments (Lin et al. 2020). Moss peat, also known as sphagnum peat or peat moss, is composed primarily of sphagnum moss and other plant material that has undergone partial decomposition under waterlogged conditions. Before tests, the peat was first oven-dried at 90oC for at least 48 h, and its dry bulk density was measured to be around 160 kg/m3. Although the hydrophilicity or hydrophobicity of peat soils may change during the heating process, the extent of their change is a function of initial soil properties and heating conditions (Wu et al. 2020). In this work, heating was performed at lower temperatures than that in Debano (2000) cited as being problematic, and the method we used to achieve peat soils with different moisture contents has been widely used and validated in the literature (Prat et al. 2015; Huang et al. 2016a; Huang and Rein 2017; Hu et al. 2019; Lin et al. 2019; Christensen et al. 2020; Lin et al. 2021a, 2021b; Cui et al. 2022).
The oven-dried weight (ODW) moisture content (MC) of the peat sample was calculated as the weight of water (initial weight of the peat sample minus the oven-dry weight) divided by the oven-dried weight. Once the oven-dried peat was exposed to ambient air, it quickly absorbed ambient moisture and reached a new equilibrium MC of around 5% ODW (Huang and Rein 2017, 2019). To obtain other desired MCs of up to 100% ODW, the oven-dried peat was mixed with the appropriate amount of pure water (Lin et al. 2019). Afterwards, the sample was left in a sealed box for equilibrium and homogeneity for another 48 h. Before the fire tests, a subsample (about 5 g) was collected from the sealed box to measure the moisture content using a moisture content analyser; thus, ensuring the variation from the desired value was smaller than 5%. During the water-absorbing process, the volume of the peat sample tended to expand naturally (Huang and Rein 2017). To avoid this issue, after being filled into the reactor, moist samples were compressed manually to ensure the dry bulk density of peat was fixed at 160 kg/m3 (Lin et al. 2019). Therefore, as the moisture content of peat increases from 0% ODW to 100% ODW, the bulk density of peat increases from 160 to 320 kg/m3.
Firebrands were generated by cutting sections of commercially available dowel rods made of poplar wood with a diameter (d) of 6 mm and a length (l) of 3 cm (Fig. 2c). This dimension is within the range of firebrands reported in experiments and actual fire scenarios (Manzello et al. 2007a, 2009a; Caton et al. 2017). Before tests, the poplar dowels were first dried in an oven at 90oC for 48 h, and their dry bulk density was measured to be around 550 kg/m3. To generate firebrands, dowels were ignited by a propane torch for around 5 s, until a self-sustained flame fully covering the entire sample surface was achieved. The firebrand was then allowed to burn freely until self-extinction of flame occurred and self-sustaining smouldering commenced (Hakes et al. 2019; Salehizadeh et al. 2021). The smouldering firebrand was then dropped onto the fuel bed in the wind tunnel.
(a) Snapshots of spotting ignition of peat fire by a firebrand, where the original video (together with the IR video) could be found in the Supplementary Materials.
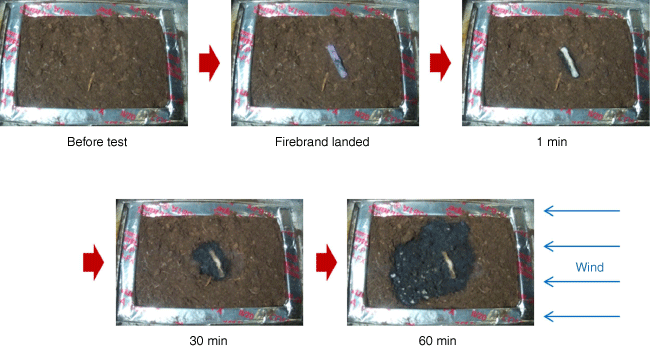
Successful smouldering ignition of the peat was defined as the observation of a blackened smouldering front propagating outwards from the firebrand to burn out the peat sample (mass loss ≥80%). In previous experiments (Salehizadeh et al. 2021; Tao et al. 2021), both the heat flux and temperature of firebrand were found to increase as the wind velocity (up to 1.4 m/s) increased, favouring the occurrence of ignition of recipient fuels. For example, for a 4-g firebrand pile (fabricated from cylindrical birch wooden dowels with similar geometry and size of the firebrand used in this work), as the wind speed increased from 0.5 to 1.4 m/s, the peak heat flux and peak temperature increased from around 17 kW/m2 and 300°C to around 22 kW/m2 and 400°C, respectively (Salehizadeh et al. 2021). However, the effective heating duration decreased from approximately 1500 s to 1000 s, as the firebrand will burn out faster under a stronger external wind. Nevertheless, if smouldering ignition was not achieved, a new test with a fresh sample was performed again but a cross airflow, ν (up to 1 m/s) was applied to increase the oxygen supply. Afterwards, the MC of peat samples was varied to explore its effect on ignition thresholds. For each scenario, at least 4–5 replicated tests were conducted to determine experimental uncertainties. During the tests, the ambient temperature was about 25 ± 2°C, the relative humidity was about 50 ± 5%, and the ambient pressure was 101 ± 1 kPa.
The experimental results were analysed using the logistic regression method to find an ignition boundary. The cases where the firebrand successfully induced smouldering ignition were labelled as 1, and those without ignition were labelled as 0. The results (i.e. 1 and 0) together with associated test conditions (i.e. MC and ν) were utilised to determine the logistic regression with a linear formula structure: f(MC,v) = a × MC + b × v + c, in which a, b, and c are parameters to be fitted. Another linear activation function is then used to map the computational value f(MC,v) to the ignition probability Pig within the range of 0–1 (Eqn 1):
The model used the logarithmic likelihood function as the loss function. The gradient descent method was adopted to update the weights (a and b) and bias term (c) at each iteration step. To make the classification results more conservative, the penalty weight for the wrong prediction of positive samples was set to twice that of negative samples. Finally, the overall model performance was evaluated concerning its classification accuracy, ROC (Receiver Operating Characteristics) curve and AUC (Area Under the Curve) value.
Results and discussion
Fire phenomena
Fig. 3 shows an example of a successful smouldering ignition by a firebrand with a cross airflow of 0.6 m/s, where the original video (together with the IR video) can be found in the Supplementary Materials. Once the firebrand landed on a peat sample, a strong glowing process could be observed, where an ash layer was formed on the exterior surface of the firebrand which then gradually expanded towards its interior. During this process, the heat released from the smouldering firebrand continuously transferred to the peat sample, assumed to primarily occur through conduction and radiation. Afterwards, some smoke was released from the surface of the peat sample that was directly heated by the firebrand, likely a combination of condensed water vapour and pyrolysis gases. After some time, as the peat sample started to pyrolyse and smoulder, the surface layer close to the firebrand turned black (or charred), and the black spot gradually expanded outwards with a higher speed in the concurrent direction. Eventually, the peat sample was completely burnt out and turned into ash with a mass loss larger than 80%. Therefore, we can conclude that peat fires could be initiated by a firebrand, which could fly over a firebreak and escape existing burning regions to accelerate fire spread. In practice, maintaining the water table at a certain level can help sustain high moisture content to prevent ignition, which aligns with recommended protective measures.
Spotting ignition thresholds
The experimental outcomes are summarised in Fig. 4, where the orange circles represent successful ignition, while the blue crosses represent no ignition. Note that there is some overlap in Fig. 4 between some symbols, indicating different results from repeated tests under the same specific experimental conditions. When the moisture content was below 35 ± 5%, spotting ignition was always achieved, regardless of external airflow velocity (Fig. 4). However, no spotting ignition was achieved when the moisture content of peat soil was above 85 ± 5%, even when the wind speed was higher than 1 m/s. Further increasing the wind velocity (which may intensify heating but shorten the heating duration because the firebrand can burn out faster; Salehizadeh et al. 2021) still cannot achieve ignition. In the literature, peat fire thresholds in terms of moisture contents were widely investigated (Frandsen 1997, 2011; Rein et al. 2008; Huang et al. 2016b; Huang and Rein 2017; Hu et al. 2019; Lin et al. 2019; Palamba et al. 2020). For example, self-sustaining peat fire may not be initiated by a coil heater at 100 W for 30 min if the peat moisture content is higher than ~160% (Rein et al. 2008; Huang et al. 2016b; Huang and Rein 2017; Hu et al. 2019). Frandsen (1997) found a critical moisture content of around 110% to achieve self-sustaining smouldering peat fire using a similar ignition protocol. Frandsen (1987) also applied a glowing hot coil (which could be regarded as an ideal firebrand) with a projected area of 1 cm × 3 cm to heat the peat moss for 3 min and found a critical moisture content of around 90% to achieve smouldering ignition. Therefore, as long as the peat moisture content is above an ignition threshold (i.e. sufficiently wet), ignition can be effectively prevented. However, as peat becomes warmer and drier due to climate change, fires may become much easier to ignite and more easily spread out of control (Rein and Huang 2021).
Summary of the experimental results, where the orange circles and the blue crosses represent successful ignition and no ignition respectively, and the black shadowed region represents a 90% confidence interval.
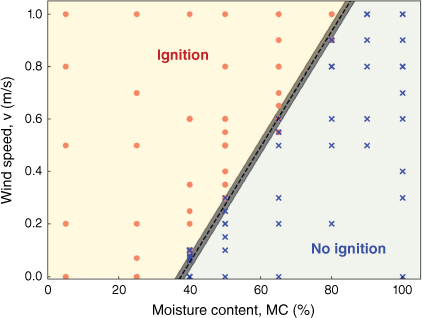
When a moderate moisture content was used, between 35 ± 5% and 85 ± 5%, the required minimum wind velocity to sustain an ignition increased as the moisture content increased. For example, as the moisture content of the peat sample increased from 40% to 80%, the required minimum wind velocity increased from approximately 0.1 m/s to around 0.9 m/s. As the moisture content increases, more heat is needed to dry the fuel before ignition, thus a higher wind velocity is required to improve the oxygen supply and accelerate the smouldering reaction rate, generating enough heat to dry and ignite the peat sample (Salehizadeh et al. 2021; Tao et al. 2021; Qin et al. 2024). A linear correlation for the ignition boundary was obtained from the logistic regression model, where the black shadowed region represents a 90% confidence interval (Fig. 4). The performance of the proposed function was evaluated (where the AUC value is 0.97), which could be found in the Appendix. Based on the experimental results and the statistical analysis, the ignition probability could be summarised as Eqn 2:
Heat transfer analysis and implications
To better understand the relationship between external wind velocity and peat moisture content for spotting ignition, an approximate and simplified heat transfer analysis based on the energy conservation equation can be used following Wang et al. (2022) and Lin et al. (2021a). As shown in Fig. 5, at the spotting ignition limit, the minimum ignition energy (Eig) should not only heat the fuel to its ignition temperature (TSM), but also overcome the heat loss due to evaporation, ambient convective and radiant cooling , and lateral conduction to the surrounding fuel during the heating duration t, as:
where ρ is the density of peat, δT is the thermal penetration depth, cp is the specific heat of peat, T∞ is ambient temperature, ΔHev is the overall heat of evaporation for water (including heat due to the moisture temperature increase from ambient to an evaporation temperature), and d and l are the diameter and the length of the firebrand respectively. The required minimum ignition energy per unit area can then be approximated as:
Further rearranging Eqn 4, we have:
where the heat from the firebrand to the peat sample can be further described as:
where α is the ratio of heat transferred from the burning firebrand to the peat sample, is the burning rate per unit area of firebrands, ΔHsm is the heat of smouldering combustion, is the rate of oxygen supply, γ is the stoichiometric factor, ρa is the density of air, andΥox is the mass fraction of oxygen in the air.
From Eqns 5 and 6, as the MC of peat increases, a larger is required to overcome heat loss due to water evaporation. Therefore, a larger ν is required to intensify the reaction of the firebrand and thus heat to the peat sample (Salehizadeh et al. 2021; Tao et al. 2021), agreeing well with the experimental results and linear correlation (35% ≤ MC ≤ 85%).
In contrast, as the size of the firebrand (d or l) decreases, the conductive heat loss to the surrounding fuel will increase (Eqn 5). Therefore, a larger or ν is required to trigger ignition. This is consistent with the finding from our previous work on spotting ignition by sunlight spots, where the lateral conductive heat loss to the virgin fuel increases as the spot diameter decreases (Wang et al. 2022). If the size of the firebrand continuously decreases, eventually there is a minimum size below which the firebrand can no longer trigger ignition, as observed by Urban et al. (2019) and summarised by Manzello et al. (2020).
Note that the outcomes drawn from this work are limited to spotting ignition driven by a single laboratory firebrand. In real fire scenarios, firebrands may deposit and accumulate to form a firebrand pile that may increase the heating area and intensity (Manzello et al. 2009b; Richter et al. 2022). As a result, the ignition boundary may be altered and fire risk can be further increased, requiring more in-depth laboratory-scale or field-scale experiments. However, this work is the first step to demonstrate that the peat fires could be initiated by lofted firebrands that may easily cross most firebreaks, accelerating the fire spread and worsening the fire scenarios. Therefore, for peatland managers and firefighters, different layers of fire protection measures should be considered to prevent spotting ignition. Large-scale peat fire experiments in the field and more fire-fighting practices (e.g. rewetting) are necessary to develop scientific guidelines.
Conclusions
In this work, we evaluated the propensity for spotting ignition of peat soil with moisture contents of up to 100% by a single glowing firebrand. We found that smouldering peat fires can be easily initiated by a firebrand which may be lofted or blown over a firebreak and escape existing burning regions to accelerate fire spread in real fire scenarios.
When the moisture content of peat is lower than 35%, ignition is always achieved with a single firebrand, regardless of wind velocity. However, if the moisture content of peat is greater than 35% but less than 85%, an external wind (increasing with peat moisture content) was required to strengthen the reaction of the firebrand and the heating to the peat sample. A 90% ignition probability curve produced by a logistic regression agrees well with the experimental results with an AUC value of 0.97. In contrast, no ignition could be achieved if the peat moisture content is higher than 85%, indicating the importance of maintaining a high moisture content of peat to prevent ignition. In future work, experiments may be conducted to quantify the ignition threshold of peat fires by numerous firebrands and piles. Large-scale peat fire experiments in the field and more studies on fire-fighting practices may also be necessary to develop scientific guidelines to prevent spotting ignition of peat fires.
Data availability
The data that supports this study will be shared upon reasonable request to the corresponding author.
Conflicts of interest
The authors declare that there is no conflict of interest between each other. Dr Xinyan Huang is an Associate Editor of the International Journal of Wildland Fire, and Dr Michael J. Gollner is on the journal’s Editorial Advisory Committee. To mitigate this potential conflict of interest, they were blinded from the review process and were not involved at any stage in the editing of this manuscript.
Declaration of funding
This work was funded by the National Institute of Standards and Technology Fire Research Grant (70NANB19H053), the Powley Fund at the University of California, Berkeley, and the National Natural Science Foundation of China (No. 52322610).
References
Atwood EC, Englhart S, Lorenz E, Halle W, Wiedemann W, Siegert F (2016) Detection and characterization of low temperature peat fires during the 2015 fire catastrophe in Indonesia using a new high-sensitivity fire monitoring satellite sensor (FireBird). PLoS one 11, e0159410.
| Crossref | Google Scholar | PubMed |
Caton SE, Hakes RSP, Gorham DJ, Zhou A, Gollner MJ (2017) Review of pathways for building fire spread in the wildland urban interface Part I: Exposure conditions. Fire Technology 53, 429-473.
| Crossref | Google Scholar |
Caton-Kerr SE, Tohidi A, Gollner MJ (2019) Firebrand generation from thermally-degraded cylindrical wooden dowels. Frontiers in Mechanical Engineering 5, 1-12.
| Crossref | Google Scholar |
Christensen EG, Fernandez-Anez N, Rein G (2020) Influence of soil conditions on the multidimensional spread of smouldering combustion in shallow layers. Combustion and Flame 214, 361-370.
| Crossref | Google Scholar |
Cobian-Iñiguez J, Richter F, Camignani L, Liveretou C, Xiong H, Stephens S, Finney M, Gollner M, Fernandez-Pello C (2022) Wind effects on smoldering behavior of simulated wildland fuels. Combustion Science and Technology 195, 3212-3229.
| Crossref | Google Scholar |
Cui W, Hu Y, Rein G (2022) Experimental study of the ignition conditions for self-sustained smouldering in peat. Proceedings of the Combustion Institute 39, 4125-4133.
| Crossref | Google Scholar |
Debano LF (2000) The role of fire and soil heating on water repellency in wildland environments: a review. Journal of Hydrology 231–232, 195-206.
| Crossref | Google Scholar |
De Beer JA, Alascio JA, Stoliarov SI, Gollner MJ (2023) Analysis of the thermal exposure and ignition propensity of a lignocellulosic building material subjected to a controlled deposition of glowing firebrands. Fire Safety Journal 135, 103720.
| Crossref | Google Scholar |
Ellis PFM (2015) The likelihood of ignition of dry-eucalypt forest litter by firebrands. International Journal of Wildland Fire 24, 225-235.
| Crossref | Google Scholar |
Fernandez-Pello AC, Lautenberger C, Rich D, Zak C, Urban J, Hadden R, Scott S, Fereres S (2015) Spot fire ignition of natural fuel beds by hot metal particles, embers, and sparks. Combustion Science and Technology 187, 269-295.
| Crossref | Google Scholar |
Frandsen WH (1987) The influence of moisture and mineral soil on the combustion limits of smouldering forest duff. Canadian Journal of Forest Research 16, 1540-1544.
| Crossref | Google Scholar |
Frandsen WH (1997) Ignition probability of organic soils. Canadian Journal of Forest Research 27, 1471-1477.
| Crossref | Google Scholar |
Frandsen WH (2011) Ignition probability of organic soils. Canadian Journal of Forest Research 27, 1471-1477.
| Crossref | Google Scholar |
Hakes RSP, Salehizadeh H, Weston-Dawkes MJ, Gollner MJ (2019) Thermal characterization of firebrand piles. Fire Safety Journal 104, 34-42.
| Crossref | Google Scholar |
Hu Y, Fernandez-Anez N, Smith TEL, Rein G (2018) Review of emissions from smouldering peat fires and their contribution to regional haze episodes. International Journal of Wildland Fire 27(5), 293-312.
| Crossref | Google Scholar |
Hu Y, Christensen EG, Amin HMF, Smith TEL, Rein G (2019) Experimental study of moisture content effects on the transient gas and particle emissions from peat fires. Combustion and Flame 209, 408-417.
| Crossref | Google Scholar |
Huang X, Rein G (2016) Thermochemical conversion of biomass in smouldering combustion across scales: the roles of heterogeneous kinetics, oxygen and transport phenomena. Bioresource Technology 207, 409-421.
| Crossref | Google Scholar | PubMed |
Huang X, Rein G (2017) Downward spread of smoldering peat fire: the role of moisture, density and oxygen supply. International Journal of Wildland Fire 26, 907-918.
| Crossref | Google Scholar |
Huang X, Rein G (2019) Upward-and-downward spread of smoldering peat fire. Proceedings of the Combustion Institute 37, 4025-4033.
| Crossref | Google Scholar |
Huang X, Restuccia F, Gramola M, Rein G (2016a) Experimental study of the formation and collapse of an overhang in the lateral spread of smouldering peat fires. Combustion and Flame 168, 393-402.
| Crossref | Google Scholar |
Huang X, Restuccia F, Gramola M, Rein G (2016b) Experimental study of the formation and collapse of an overhang in the lateral spread of smouldering peat fires. Combustion and Flame 168, 393-402.
| Crossref | Google Scholar |
Ju X, Conkling M, Hajilou M, Lin S, Mostafa F, Ayyar A, McDowell A, Lisano M, Gollner MJ (2023) Laboratory quantification of firebrand generation from WUI fuels for model development. Fire Safety Journal 141, 103921.
| Crossref | Google Scholar |
Kohlenberg AJ, Turetsky MR, Thompson DK, Branfireun BA, Mitchell CPJ (2018) Controls on boreal peat combustion and resulting emissions of carbon and mercury. Environmental Research Letters 13, 035005.
| Crossref | Google Scholar |
Lin S, Huang X (2020) An experimental method to investigate the water-based suppression of smoldering peat fire. MethodsX 7, 100934.
| Crossref | Google Scholar |
Lin S, Sun P, Huang X (2019) Can peat soil support a flaming wildfire? International Journal of Wildland Fire 28, 601-613.
| Crossref | Google Scholar |
Lin S, Cheung YK, Xiao Y, Huang X (2020) Can rain suppress smoldering peat fire? Science of the Total Environment 727, 138468.
| Crossref | Google Scholar | PubMed |
Lin S, Liu Y, Huang X (2021a) How to build a firebreak to stop smouldering peat fire: insights from a laboratory-scale study. International Journal of Wildland Fire 30, 454-461.
| Crossref | Google Scholar |
Lin S, Liu Y, Huang X (2021b) Climate-induced Arctic-Boreal Peatland Fire and Carbon Loss in the 21st Century. Science of the Total Environment 796, 148924.
| Crossref | Google Scholar |
Lin S, Chow TH, Huang X (2021c) Smoldering propagation and blow-off on consolidated fuel under external airflow. Combustion and Flame 234, 111685.
| Crossref | Google Scholar |
Manzello SL, Cleary TG, Shields JR, Yang JC (2006) Ignition of mulch and grasses by firebrands in wildland–urban interface fires. International Journal of Wildland Fire 15(3), 427-431.
| Crossref | Google Scholar |
Manzello SL, Maranghides A, Mell WE (2007a) Firebrand generation from burning vegetation. International Journal of Wildland Fire 16, 458-462.
| Crossref | Google Scholar |
Manzello SL, Maranghides A, Shields JR, Mell WE, Hayashi Y, Nii D (2007b) Measurement of firebrand production and heat release rate (HRR) from burning Korean pine trees. Fire Safety Science 7, 108.
| Google Scholar |
Manzello SL, Cleary TG, Shields JR, Maranghides A, Mell W, Yang JC (2008) Experimental investigation of firebrands: generation and ignition of fuel beds. Fire Safety Journal 43, 226-233.
| Crossref | Google Scholar |
Manzello SL, Maranghides A, Shields JR, Mell WE, Hayashi Y, Nii D (2009a) Mass and size distribution of firebrands generated from burning Korean pine (Pinus koraiensis) trees. Fire and Materials 33, 21-31.
| Crossref | Google Scholar |
Manzello SL, Park S-H, Cleary TG (2009b) Investigation on the ability of glowing firebrands deposited within crevices to ignite common building materials. Fire Safety Journal 44, 894-900.
| Crossref | Google Scholar |
Manzello SL, Suzuki S, Gollner MJ, Fernandez-Pello AC (2020) Role of firebrand combustion in large outdoor fire spread. Progress in Energy and Combustion Science 76, 100801.
| Crossref | Google Scholar | PubMed |
Mulyasih H, Akbar LA, Ramadhan ML, Cesnanda AF, Putra RA, Irwansyah R, Nugroho YS (2022) Experimental study on peat fire suppression through water injection in laboratory scale. Alexandria Engineering Journal 61, 12525-12537.
| Crossref | Google Scholar |
Page SE, Siegert F, Rieley JO, Boehm H V, Jaya A, Limin S (2002) The amount of carbon released from peat and forest fires in Indonesia during 1997. Nature 420, 61-65.
| Crossref | Google Scholar | PubMed |
Palamba P, Allo R, Kosasih E, Nugroho Y (2020) Effect of surface dry layer thickness on the smoldering combustion of a stratified moisture content peat layer. International Journal of Advanced Science and Technology 29, 2117-2139.
| Google Scholar |
Poulter B, Christensen NL, Halpin PN (2006) Carbon emissions from a temperate peat fire and its relevance to interannual variability of trace atmospheric greenhouse gases. Journal of Geophysical Research Atmospheres 111, 1-11.
| Crossref | Google Scholar |
Prat N, Belcher CM, Hadden RM, Rein G, Yearsley JM (2015) A laboratory study of the effect of moisture content on the spread of smouldering in peat fires. Flamma 6, 35-38.
| Google Scholar |
Qin Y, Chen Y, Lin S, Huang X (2022a) Limiting oxygen concentration and supply rate of smoldering propagation. 245,.
| Crossref | Google Scholar |
Qin Y, Musa DNS, Lin S, Huang X (2022b) Deep peat fire persistently smouldering for weeks: a laboratory demonstration. International Journal of Wildland Fire 32(1), 86-98.
| Crossref | Google Scholar |
Qin Y, Zhang Y, Chen Y, Lin S, Huang X (2024) Minimum oxygen supply rate for smouldering propagation: effect of fuel bulk density and particle size. Combustion and Flame 261, 113292.
| Crossref | Google Scholar |
Ramadhan ML, Palamba P, Imran FA, Kosasih EA, Nugroho YS (2017) Experimental study of the effect of water spray on the spread of smoldering in Indonesian peat fires. Fire Safety Journal 91, 671-679.
| Crossref | Google Scholar |
Rein G (2013) Smouldering fires and natural fuels. In ‘Fire Phenomena and the Earth System: An Interdisciplinary Guide to Fire Science’. (Ed. CM Belcher) pp. 15–33. 10.1002/9781118529539.ch2
Rein G, Huang X (2021) Smouldering wildfires in peatlands, forests and the Arctic: challenges and perspectives. Current Opinion in Environmental Science & Health 24, 100296.
| Crossref | Google Scholar |
Rein G, Cleaver N, Ashton C, Pironi P, Torero JL (2008) The severity of smouldering peat fires and damage to the forest soil. Catena 74, 304-309.
| Crossref | Google Scholar |
Rein G, Huang X, Restuccia F, McArdle T (2017) Detection of landmines in peat soils by controlled smouldering combustion: experimental proof of concept of O-Revealer. Experimental Thermal and Fluid Science 88, 632-638.
| Crossref | Google Scholar |
Richter F, Bathras B, Barbetta Duarte J, Gollner MJ (2022) The propensity of wooden crevices to smoldering ignition by firebrands. Fire Technology 58, 2167-2188.
| Crossref | Google Scholar |
Salehizadeh H, Hakes RSP, Gollner MJ (2021) Critical ignition conditions of wood by cylindrical firebrands. Frontiers in Mechanical Engineering 7, 1-13.
| Crossref | Google Scholar |
Santoso MA, Cui W, Amin HMF, Christensen EG, Nugroho YS, Rein G (2021) Laboratory study on the suppression of smouldering peat wildfires: effects of flow rate and wetting agent. International Journal of WIldland Fire 30, 378-390.
| Crossref | Google Scholar |
Santoso MA, Christensen EG, Amin HMF, Palamba P, Hu Y, Purnomo DMJ, Cui W, Pamitran A, Richter F, Smith TEL, Nugroho YS, Rein G (2022) GAMBUT field experiment of peatland wildfires in Sumatra: from ignition to spread and suppression. International Journal of Wildland Fire 31, 949-966.
| Crossref | Google Scholar |
Suzuki S, Manzello SL (2016) Firebrand production from building components fitted with siding treatments. Fire Safety Journal 80, 64-70.
| Crossref | Google Scholar | PubMed |
Song J, Huang X, Liu N, Li H, Zhang L (2017) The wind effect on the transport and burning of firebrands. Fire Technology 53, 1555-1568.
| Crossref | Google Scholar |
Tao Z, Bathras B, Kwon B, Biallas B, Gollner MJ, Yang R (2021) Effect of firebrand size and geometry on heating from a smoldering pile under wind. Fire Safety Journal 120, 103031.
| Crossref | Google Scholar |
Thomas JC, Mueller E V, Santamaria S, Gallagher M, El Houssami M, Filkov A, Clark K, Skowronski N, Hadden RM, Mell W, Simeoni A (2017) Investigation of firebrand generation from an experimental fire: development of a reliable data collection methodology. Fire Safety Journal 91, 864-871.
| Crossref | Google Scholar |
Tohidi A, Kaye NB (2017) Comprehensive wind tunnel experiments of lofting and downwind transport of non-combusting rod-like model firebrands during firebrand shower scenarios. Fire Safety Journal 90, 95-111.
| Crossref | Google Scholar |
Urban JL, Zak CD, Fernandez-Pello C (2018) Spot fire ignition of natural fuels by hot aluminum particles. Fire Technology 54, 797-808.
| Crossref | Google Scholar |
Urban JL, Song J, Santamaria S, Fernandez-pello C (2019) Ignition of a spot smolder in a moist fuel bed by a firebrand. Fire Safety Journal 108, 102833.
| Crossref | Google Scholar |
Wadhwani R, Sullivan C, Wickramasinghe A, Kyng M, Khan N, Moinuddin K (2022) A review of firebrand studies on generation and transport. Fire Safety Journal 134, 103674.
| Crossref | Google Scholar |
Wang S, Huang X, Chen H, Liu N (2017) Interaction between flaming and smouldering in hot-particle ignition of forest fuels and effects of moisture and wind. International Journal of Wildland Fire 26, 71-81.
| Crossref | Google Scholar |
Wang S, Lin S, Liu Y, Huang X, Gollner MJ (2022) Smoldering ignition using a concentrated solar irradiation spot. Fire Safety Journal 129, 103549.
| Crossref | Google Scholar |
Wu Y, Zhang N, Slater G, Waddington JM, de Lannoy CF (2020) Hydrophobicity of peat soils: characterization of organic compound changes associated with heat-induced water repellency. Science of the Total Environment 714, 136444.
| Crossref | Google Scholar | PubMed |
Yang J, Chen H (2018) Natural downward smouldering of peat: effects of inorganic content and piled bed. Fire Technology 54, 1219-1247.
| Crossref | Google Scholar |
Yin P, Liu N, Chen H, Lozano JS, Shan Y (2014) New correlation between ignition time and moisture content for pine needles attacked by firebrands. Fire Technology 50, 79-91.
| Crossref | Google Scholar |
Zhang Y, Shu Y, Qin Y, Chen Y, Lin S, Huang X, Zhou M (2024) Resurfacing of underground peat fire: smoldering transition to flaming wildfire on litter surface. International Journal of Wildland Fire 33, WF23128.
| Crossref | Google Scholar |
Zhu L, Urban JL (2023) Cooperative spot ignition by idealized firebrands: impact of thermal interaction in the fuel. Fire Safety Journal 135, 103701.
| Crossref | Google Scholar |
Appendix
To evaluate the performance of the logistic regression, the AUC-ROC curve is applied here, which measures how much the model is capable of distinguishing between classes based on TPR (True Positive Rate) and FPR (False Positive Rate). ROC (Receiver Operating Characteristics) is a probability curve and AUC (Area Under the Curve) represents the degree or measure of separability. If a model has an AUC higher than 0.8, it is regarded as a high-performance model with excellent or outstanding discrimination.
TPR and FPR could be calculated as
Where TP, TN, FP, FN represent True Positive, True Negative, False Positive and False Negative. As shown in Fig. A1, the simulated optimal threshold and AUC with a value of 0.97 demonstrate the outstanding discrimination of the fitting in Fig. 4.