Mercury in an Australian sclerophyll Eucalyptus forest and emissions from fuel reduction prescribed burning
James R. Taylor



A
B
C
D
E
F
Abstract
Understanding how mercury cycles through the environment is crucial for protecting ecosystems and human health. Our study is among the first to measure mercury concentrations in Eucalyptus forest soils and litter and estimate emissions from prescribed burns, addressing a significant gap in current knowledge. These new data enhance our understanding of mercury cycling in Australia and contribute to the global information on the biogeochemical cycle of mercury.
Research on mercury in Australian soils and litter is sparse. This study aims to address this knowledge gap by investigating mercury pools in soil and litter in a eucalypt forest in Victoria, Australia.
We analysed total mercury concentrations in O and A horizon soils, and twig, bark and leaf litter. Soil samples were collected from an area affected by a prescribed burn and unburned areas. Additionally, soil samples from the base of tree stems were taken in unburned areas. The organic matter content of all soil samples was also assessed.
In unburned soils, mean mercury concentrations at the base of tree stems, in the O-horizon and A horizon were 143 ± 61, 112 ± 71 and 56 ± 30 ng g−1 respectively. In burned soils, mean mercury concentrations in the O and A horizons were 91 ± 63 and 46 ± 19 ng g−1 respectively. Mercury concentrations in leaf, bark and twig litter averaged 71 ± 11, 21 ± 13 and 8 ± 4 ng g−1 respectively. The emission factor was estimated as 0.247 g Hg ha−1.
The studied sclerophyll forest represents a significant mercury reservoir. Burning did not significantly alter the mercury burden in soil; however, emissions of mercury from litter did occur. This finding underscores the need for more comprehensive research into mercury cycling in Australia and suggests that prescribed burning practices should account for potential mercury emissions.
Keywords: Australia, emissions, eucalypt, Hg, mercury, mobilisation, mycorrhizal, prescribed fire.
Introduction
Mercury (Hg) is a naturally occurring toxic element found in varying concentrations in soils worldwide (Gustin et al. 2008). Forest soil functions as a sink for atmospheric Hg and represents a major pool of global Hg (Gustin et al. 2005; Obrist 2007). Once Hg is emitted into the atmosphere, it falls onto the earth’s surface through wet and dry deposition (Lindqvist et al. 1991; Fitzgerald 1995; Wang D et al. 2003).
The dominant atmospheric Hg species, elemental Hg (Hg0), has an atmospheric lifetime of 3–6 months (Saiz-Lopez et al. 2020; Shah et al. 2021; Zhang and Zhang 2022), therefore Hg emissions can be distributed far from the point sources. Oxidation of Hg0 occurs in the atmosphere forming reactive Hg (Hg2+), which has a short residence time of days to weeks (Lin and Pehkonen 1999). Upon deposition into terrestrial and aquatic ecosystems, inorganic Hg can be methylated by microorganisms to methylmercury (CH3Hg), a neurotoxin (Basu et al. 2023) that can bioaccumulate in the food web (Obrist et al. 2018). Thus, there is great interest in understanding Hg cycling and in determining the potential for pools of Hg to enter specific ecosystems.
Forest fires may provide a significant flux of Hg from terrestrial ecosystems to the atmosphere (Biswas et al. 2007). Forest fires release Hg0 to the atmosphere from soils as well as from living and dead vegetation by volatilisation and thermal desorption (Outridge et al. 2018; Filimonenko et al. 2024). Atmospheric Hg emissions have been observed in fire smoke plumes (Artaxo et al. 2000; Howard et al. 2019). Fires also release Hg from soil to aquatic systems by reducing vegetation cover, allowing soil erosion and facilitating Hg transport to water (Amirbahman et al. 2004; Dittman et al. 2010).
One of the primary mechanisms for removing atmospheric Hg is the direct uptake of gaseous Hg0 by plants (Wang X et al. 2016a; Jiskra et al. 2018; Obrist et al. 2021; Zhou and Obrist 2021). The Hg is transferred to soils as litterfall during the turnover of plant biomass components as they shed leaves, twigs, bark and fruit, as well as from throughfall when rain washes deposited Hg off the plant surfaces. As such, Hg in litterfall and throughfall have been used as proxies to measure Hg dry deposition to terrestrial environments (Fostier et al. 2015).
Globally, the terrestrial environment is estimated to receive ~3600 Mg year−1 of atmospheric Hg deposition (Outridge et al. 2018), from which 2276 Mg year−1 (63%) would be due to Hg0 dry deposition according to the most recent estimates (Feinberg et al. 2022). From this amount, 73% would be taken up by forest vegetation and the rest by cropland, grassland, and shrubland (Zhou and Obrist 2021). Thus, Hg concentration measurements of litterfall are of great importance to assess Hg deposition in forest areas.
Litter decomposes and Hg becomes complexed with reduced sulfur compounds in the organic soil (Lindqvist et al. 1991; Skyllberg et al. 2003). Soils typically have higher Hg concentrations than litter because carbon is lost at a greater rate than Hg during the mineralisation of organic matter (Grigal 2003; Hall and St Louis 2004; Pokharel and Obrist 2011 in Gómez-Armesto et al. 2020). The rate of deposition in soil is spatially variable, depending on the vegetation type, coverage and growth rates, and atmospheric Hg concentrations at a given site (Johnson and Lindberg 1995; Ericksen and Gustin 2004; Cobbett and Van Heyst 2007).
In Australia, research on Hg concentrations in natural soils and litter is limited. A study on natural Hg in soils collected from Pumphouse, central Victoria, reported mean concentrations of 29.4 ng g−1 in the topsoil (0–2 cm) and 25.3 ng g−1 in lower soil (5–10 cm) (Howard et al. 2019). The same study reported mean Hg concentrations in leaf, bark and twig litter as 47.8, 24.5 and 11.2 ng g−1 respectively.
A study conducted in Victoria’s Latrobe Valley analysed five topsoil samples and reported an average Hg concentration of 53.5 ng g−1, with a range from 23.2 to 93.5 ng g−1 (Schneider et al. 2021). In a preliminary study in Victoria and south-eastern New South Wales, Packham et al. (2009) reported Hg concentrations of 90, 110 and 140 ng g−1.
Similarly to Hg concentrations in soils, there is limited understanding of Hg emissions from fires in Australia. Although biomass burning (both anthropogenic and wildfires) has been recognised as a major source of Hg release into the atmosphere (Friedli et al. 2009; Shi et al. 2019; Francisco López et al. 2022), estimates of Hg emissions from biomass burning in Australia remain highly uncertain (Fisher et al. 2023). This could be relevant as prescribed burning is a widely used form of land management in Australia. Prescribed fires are planned with respect to weather conditions, fuel loads and ignition techniques; they are contrasted to wildfires (or ‘bushfires’ in Australia), which are not planned (Morgan et al. 2020).
In this study, we analysed the Hg pool in a wet sclerophyll forest in Victoria. We examined the in-depth distribution of Hg between organic and mineral soil layers and at the base of tree stems (referred to as ‘base of tree’ throughout this study). Additionally, Hg concentrations were measured in leaves, bark and twigs in the litter layer to provide a comprehensive understanding of the Hg pool within the study site. Taking advantage of a prescribed burn at our study site, we measured Hg levels in both burned and unburned areas and calculated the estimated Hg emissions to determine Hg losses due to the burn. Specifically, we tested the following null hypotheses: (i) Hg concentrations are the same in organic topsoils and mineral A horizon soil; (ii) there is a relationship between Hg concentration and organic matter content in soils; (iii) soils subject to the prescribed burn have the same concentrations of Hg as unburned soil; and (iv) the leaf component of litter contributes the same portion of Hg among litter types. Finally, we identified the forest compartment (soil horizons and litter components) most affected by prescribed burning in relation to Hg emissions within the Australian sclerophyll forest ecosystem, and we provide recommendations for future research in this area.
Experimental
Site description
The study area (38°03′S, 146°25′E) is classified ecologically as lowland wet sclerophyll forest in the Highlands – Southern Fall bioregion of Gippsland, Victoria (EVC 16). Located in the Boola Boola State Forest between the townships of Walhalla and Tyers (Fig. 1), the forest overstorey is dominated by silvertop ash (Eucalyptus sieberi). Messmate–stringybark (E. obliqua), narrow leaf peppermint (E. radiata) and broad-leaved peppermint (E. dives) comprise the rest of the overstorey.
Map of the study area (right panel) highlighting collection points with blue markers for unburned sites and brown markers for burned sites. The hatched area indicates the extent of the planned burn; however, burning extended beyond these boundaries, accounting for the presence of brown markers outside the shaded region. The extent of spread outside the planned area is unknown. The lower left panel presents the study site’s position relative to the towns of Walhalla and Tyers. The upper left panel provides a broader view of the study site’s location within Australia. The map was created using ArcGIS Pro (ver. 3.1.3, Environmental Systems Research Institute, Redlands, CA, USA).
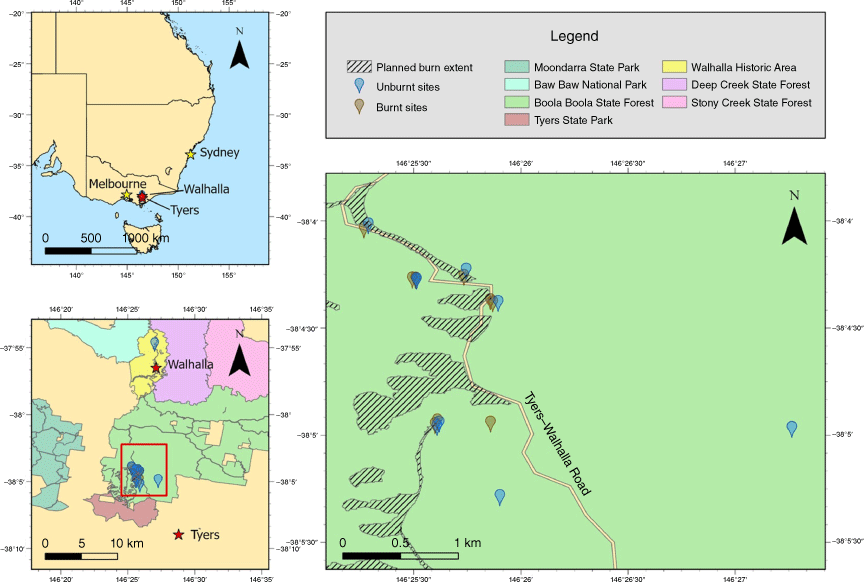
Sclerophyll forests are characterised by trees with hard, leathery, evergreen foliage specially adapted to long periods of dryness and heat and for prevention of moisture loss (Heise-Pavlov and Procter-Gray 2021). The study site climate is temperate (type Cfb according to Köppen), cool-wet, with average annual precipitation 803 mm and temperature 11.8°C (Climate Data 2024). The area is exposed to moderate to high rainfall and weathering of tertiary sediments, resulting in moderately to strongly acidic, leached soils with well-developed horizons (Aldrick et al. 1992). The area is characterised by Yellow Dermosols (code DEAC in the Australian Soil Classification), that is, soils lacking a strong contrast between the A and B horizons (Isbell and National Committee on Soil and Terrain 2021). In this study, we defined the O horizon as the visible organic layer with distinct darker brown colouration and the A horizon as the 3-cm layer immediately below the O horizon.
The study site underwent a prescribed burn in March 2022, predominantly on the western side of Tyers–Walhalla Road (Fig. 1). This has offered a unique opportunity to conduct a paired study of total Hg concentrations in soil from inside and outside of the prescribed burn. An area of ~290 ha was burned as part of this March 2022 prescribed burning (the planned burn extent is marked on the map in Fig. 1 by the hatched area).
Prior to this 2022 prescribed burn, the most recent burn in the area occurred in autumn 2004, conducted as a prescribed burn by the Victorian Department of Energy, Environment and Climate Action (DEECA).
Sample collection and processing
Field sampling was conducted over two winter campaigns. Soil and leaf litter samples were collected in July 2022, whereas bark and twig samples were collected in June 2024. The second campaign was necessary because bark and twigs were not collected during the initial campaign, and it was subsequently determined that these data are essential in calculating Hg emissions from the burning of biomass.
This work was conducted under permit AA-0001030 on land managed by Parks Victoria.
Soil sampling was conducted using a plastic hand trowel, cleaned with ethanol between each collection. Ten bulk soil samples were collected from unburned areas and an additional 10 from burned areas, with samples taken from both the O and A horizons. Owing to the considerable variability in the thickness of the O horizon between sites, a uniform O horizon sampling depth was deemed unsuitable for this study. Instead, the depth of each O horizon sample was recorded for each soil sample, and 3 cm of each underlying A horizon was collected.
To improve our understanding of Hg distribution in Australian soils, we collected soil from the O horizon at the base of tree stems at each unburnt site. This horizon results from the accumulation of litter and the transfer of material deposited on canopies from throughfall and stemflow. The base of tree stems is recognised as an area rich in organic matter (OM), which may facilitate Hg methylation, resulting in higher concentrations of Hg (Sun et al. 2011; Wang J et al. 2022). A total of 50 soil samples were collected for analysis.
Soil samples were stored in 50-mL plastic vials and transported inside a cooler at 4°C to the Paleoworks Laboratory in the Department of Archaeology and Natural History, Australian National University, Canberra. Samples were freeze-dried over 48 h using a Christ Alpha 1–2 LD plus lyophiliser. Following drying, soils were disaggregated and sieved to 63 µm using a stainless-steel laboratory sieve and a Fritsch Analysette Model 3 Spartan – vibratory sieve shaker. The <63- and >63-µm fractions were stored individually. Only the <63-µm fractions were analysed for Hg and OM.
During the July 2022 campaign, litter was collected from the surface layer of the forest floor within the study area, focusing on obtaining a representative sample of the organic material present. Sampling was conducted in 10 randomly selected 1-m2 quadrats at unburned sites, and another 10 1-m2 quadrats were selected at burned sites. In each quadrat, leaf litter was carefully gathered using gloves. The June 2024 campaign followed the same protocol, specifically to collect twigs and bark from 1-m2 quadrats. Twigs were collected in a range of sizes within <6 mm in diameter.
The leaf litter samples were placed in aluminium trays and dried in front of a gas heater over 2 days. The dried samples were placed in pre-labelled Ziplock bags and transported to the Palaeoworks Laboratory in the Department of Archaeology and Natural History, Australian National University (ANU) in Canberra. Twigs and bark were transported to the ANU immediately after collection, frozen, and then freeze-dried for 48 h using a Christ Alpha 1–2 LDplus lyophiliser. The various litter materials – leaves, twigs and bark – from each site were individually homogenised using an IKA A 11 basic analytical mill. After milling, the litter samples were sieved to <500 µm with a Fritsch Analysette Model 3 Spartan Vibratory Sieve Shaker, using a stainless-steel sieve, to remove wood particles and ensure analytical replicability. Ground twig and bark material were not sieved, as their homogeneity was sufficient for replicability. All fractions were individually stored and analysed.
Mercury analyses
For Hg analyses, 100 ± 20 mg of the sample was weighed into nickel boats and analysed using a Milestone Direct Mercury Analyser (DMA-80 Tricell; Milestone, Bergamo, Italy), following USEPA method 7473 (United States Environmental Protection Agency 1998). The DMA-80 thermally decomposes all species to Hg0, using amalgamation and then quantifies total Hg in solid samples by atomic absorption spectrometry. A pair of blanks and a pair of certified reference materials (SRM 2706, New Jersey soil, from the National Institute of Standards and Technology, USA) were analysed for every 36 soil samples. For litter samples, SRMs 1515 (apple leaves) and 1575a (pine needles) were used as reference materials. Care was taken to analyse additional SRM pairs with concentrations falling in the same spectrometer cell of the DMA-80. The results of the standard reference material are provided in Supplementary Table S1.
Duplicate samples were run for every five samples, results for these pairs varied less than 10% and were averaged and reported as the mean. When Hg concentration varied by greater than 10% for a duplicate pair, a triplicate was analysed.
Organic matter analyses of soil
Organic matter was analysed using the loss on ignition analysis of organic matter content described by Wang Q et al. (2011), which comprises heating ~1 g of sample in a muffle furnace (LABEC, model CEMLL) at 550°C for 8 h. Samples were left in the furnace to cool for 4 h and then weighted. The difference between initial and final weight was used to calculate the percentage of organic matter loss.
Emission factor and biomass combustion calculation
The Hg emission factor (EF) from biomass combustion in each compartment (i) (EFi, g Hg ha−1) was calculated according to Eqn 1:
where DBi is the dry biomass in a specific compartment (leaf, bark or twig) (Mg biomass ha−1), CHgi is the Hg concentration (ng g−1) in the biomass of the compartment i, and CC is the combustion completeness, which is defined as the ratio of fuel consumed by fire to total available fuel in the biomass category.
The dry litter biomass for the sclerophyll forest was estimated from published and unpublished studies of litter and combustion in prescribed fires (Keith 1991; Volkova and Weston 2015; Neumann et al. 2021). The total aboveground litter dry mass for E. sieberi foothill forest in Victoria was 13.5 Mg ha−1 (Volkova and Weston 2015). The range in litter mass for sclerophyll open forest in south-east Australia includes 11–19 Mg ha−1 (Keith 1991; Neumann et al. 2021). Based on the range of available data, we used a conservative estimate of litter mass of 12.5 Mg ha−1. The overall EF from the biomass combustion (EFbio, g Hg ha−1) was then calculated according to Eqn 2:
The EFbio (ng Hg g−1) for each compartment i, was also calculated as the mass of Hg emitted per unit of dry biomass burned (Andreae and Merlet 2001) according to Eqn 3:
where CMi corresponds to the component of the combustible material that makes up the litter. The overall EFbio (ng Hg g−1) was then calculated with Eqn 4:
In specific research studies, combustion factors for litter in prescribed fires have been reported as 60% for forests and woodlands in Victoria (Volkova et al. 2019), 70% in E. sieberi open forest in Victoria (Volkova and Weston 2015), 66–84% in E. sieberi forest in coastal Victoria (H. Keith, unpubl. data) and 68% in E. pauciflora forest in the the Australian Capital Territory (Keith 1991). We estimated an average combustion efficiency of 66%.
Our study site is classified as a cool wet forest based on the forest litter type categorisation by Neumann et al. (2021). To calculate Hg emissions, we used data from Neumann et al. (2021), which was gathered from cool wet climates in the south-eastern mountains of Australia. Additionally, we incorporated field data from alpine ash (E. delegatensis, a species closely related to silvertop ash) in the southern tablelands of New South Wales. The litter composition was estimated as 20% leaves, 30% twigs, 20% bark and 30% other materials, with the ‘other’ category referring to unidentified fragmented material present in the lower layers of the litter.
Prescribed fire burns all fine litter components, including bark and twig components (NSW Rural Fire Service 2005). For Hg emission calculations, all components are included in the percentage of burned fuel. The proportion of other unidentified fragmented material was reallocated to the leaves, twigs and bark categories to give proportions of the burned material, estimated to be 29% leaves, 43% twigs and 28% bark. It is important to note that only fallen leaves were sampled as the canopy should not burn during a prescribed fire, provided the burn is conducted according to the prescribed guidelines.
Statistical analysis
All statistical analyses were performed using the R statistical package (ver. 4.4.0, R Foundation for Statistical Computing, Vienna, Austria, see https://www.r-project.org/). Prior to analyses, all Hg concentration and OM percentage data were log-transformed to meet the assumption of normality. Where this assumption was not met, non-parametric analyses were used. An alpha level of 0.05 was applied for significance in all statistical tests. The full results of all statistical tests are presented in the supplementary data.
Two Analysis of Variance (ANOVA) tests were conducted on unburned soils: one totest for differences in Hg concentrations between the different soil types (‘base of tree’, O and A horizon soils), and another to test for differences in organic matter content in the same soils. Post hoc tests were carried out using Tukey’s Honest Statistical Difference (HSD) test.
Pearson correlations were calculated for mercury and organic matter concentrations in the following categories: combined burned soils, combined unburned soils, soils from the base of trees, unburned O and A horizon soils, and burned O and A horizon soils.
Two linear mixed models were employed to analyse the impact of burning and differences between O and A horizon data regarding organic matter and Hg concentration. The R packages lme4 (ver. 1.1.35.5, see https://CRAN.R-project.org/package=lme4; Bates et al. 2015) and lmertest (ver. 3.1.3, see https://CRAN.R-project.org/package=lmerTest; Kuznetsova et al. 2017) were used in model construction. Site was included as a random effect in both models to account for potential data variability not attributable to the fixed effects. The models were fitted using restricted maximum likelihood (REML). Pairwise comparisons of estimated marginal means were conducted where post hoc testing was necessary using the R package emmeans (ver. 1.10.3, see https://cran.r-project.org/package=emmeans).
A Kruskal–Wallis test was used to test for a significant difference between the leaf, bark and twig litter. The dunn.test package (ver. 1.3.6, see https://cran.r-project.org/package=dunn.test) was used to carry out post hoc pairwise comparisons.
Results
Results are presented in the following sub-sections; unburned soils, followed by samples affected by the burn and the results from litter analysis. Calculated Hg emissions are presented last. Mercury concentrations and OM percentages in soil samples are presented in Table 1, and in litter samples in Table 2. All Hg concentration and OM content data are presented in Supplementary Tables S2–S5.
Tree base | O Horizon | A Horizon | |||||||||
---|---|---|---|---|---|---|---|---|---|---|---|
Hg (ng g−1) | OM (%) | Depth (cm) | Hg (ng g−1) | OM (%) | Depth (cm) | Hg (ng g−1) | OM (%) | Depth (cm) | |||
Combined (unburned + burned) | Mean | 143 | 50 | 3 | 101 | 23 | 2.9 | 50.7 | 9 | 7 | |
s.d. | 61.1 | 29 | 3 | 65.4 | 14 | 2.7 | 24.5 | 4 | 3 | ||
Median | 144 | 49 | 3 | 77.1 | 22 | 2 | 42.5 | 8 | 5 | ||
Max. | 241 | 90 | 3 | 269 | 65 | 8 | 113 | 21 | 13 | ||
Min. | 59.5 | 13 | 3 | 37.9 | 3 | 1 | 21.2 | 6 | 4 | ||
n | 10 | 19 | 19 | ||||||||
Unburned | Mean | 143 | 50 | 3 | 112 | 28 | 4.1 | 56.2 | 9 | 8 | |
s.d. | 61.1 | 29 | 3 | 70.5 | 18 | 3.1 | 29.6 | 2 | 3 | ||
Median | 144 | 49 | 3 | 89.8 | 24 | 3 | 42.5 | 9 | 7 | ||
Max. | 241 | 90 | 3 | 269 | 65 | 8 | 113 | 12 | 13 | ||
Min. | 59.5 | 13 | 3 | 42.4 | 3 | 1 | 27.1 | 6 | 4 | ||
n | 10 | 9 | 9 | ||||||||
Burned | Mean | 91.0 | 19 | 1.4 | 45.7 | 10 | 4 | ||||
s.d. | 62.7 | 9 | 0.8 | 19.0 | 5 | 1 | |||||
Median | 72.3 | 21 | 1 | 43.3 | 8 | 4 | |||||
Max. | 257 | 30 | 3 | 83.2 | 21 | 6 | |||||
Min. | 37.9 | 6 | 1 | 21.2 | 6 | 4 | |||||
n | 10 | 10 |
Depth is recorded at the base of the sample. s.d., standard deviation (2σ).
Concentration (ng g−1) | |||||
---|---|---|---|---|---|
Leaf | Bark | Twigs | |||
Combined | Mean | 71.0 | 21.2 | 7.5 | |
s.d. | 11.1 | 13.1 | 3.9 | ||
Median | 69.4 | 15.9 | 6.3 | ||
Min. | 92.1 | 7.9 | 4.3 | ||
Max. | 52.8 | 62.9 | 21.8 | ||
n | 19 | 35 | 35 |
The soil horizon profile in the studied sclerophyll forest exhibited significant variability in depth (Table 1 and Supplementary Table S2). The O horizon ranged from 1 to 8 cm, with a mean depth of 2.9 ± 2.7 cm. This considerable variability highlights the importance of sampling soils based on the actual length of the O horizon, rather than using a fixed sampling depth. Relying on a fixed depth may not consistently capture the boundary between the O and A horizons, indicating the need for site-specific sampling methods.
Soil
Mean Hg concentrations in unburned soils were highest in samples collected from the base of trees, followed by O horizon soils, and A horizon soils (143 ± 61, 112 ± 71, 56 ± 30 ng g−1 respectively) (Table 1, see Fig. 2 for medians). Soil organic matter is highest at the base of tree stems due to the accumulation of litterfall and consequently high microbial bacterial activity (Ryan and McGarity 1983). Therefore, Hg concentrations from the base of trees were analysed only in unburned sites to characterise soil Hg levels in the sclerophyll forest, rather than to assess emissions from burning. These high Hg levels are important to measure and record because they contribute to the total Hg load in the soil.
Median Hg concentrations in tree base, A and O horizon soils. A and O horizon soils are presented for both burned and unburned sites. Note that soil samples from the tree base were collected only from unburned sites. The central line within each box represents the median Hg concentration, whereas the edges of the box denote the first and third quartiles (Q1 and Q3). The whiskers extend to the smallest and largest values within 1.5 times the interquartile range (IQR). Outliers beyond this range are marked as individual points.
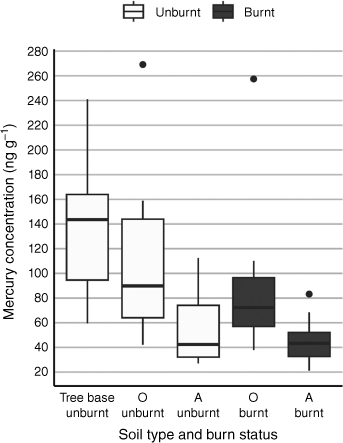
One-way ANOVA indicated a significant main effect of soil layer (unburned O and A horizons, and base of the tree) on Hg concentration (F2, 45 = 16.45, P < 0.0001) (Fig. 2, Supplementary Table S6). Tukey’s HSD post hoc testing revealed statistically significant differences between the unburned O and A horizons (P < 0.005) and soils from the base of trees and the unburned A horizon (P < 0.0001) (Fig. 2, Supplementary Table S6). No significant difference was found between unburned soil from the O horizon and the base of trees (P = 0.0936) (Supplementary Table S6).
Mean OM content in unburned soils was highest in samples collected from the base of trees, followed by O horizon soils, and A horizon soils (50 ± 29, 28 ± 18, 9 ± 2% respectively) (Table 1). Median OM content followed the same pattern (49, 24, 9%) (Table 1, Fig. 3). This difference is statistically significant, as indicated by the Welch’s ANOVA (F2, 19.445 = 23.699, P < 0.0001) (Supplementary Table S6). Post hoc testing with the Holm-adjusted Games–Howell test yielded significant differences between OM concentrations in O and A horizon soils (P < 0.05), tree base soils and A horizon soils (P < 0.005), and between tree base soils and O horizon soils (P < 0.005) (Supplementary Table S6).
Median percentage of organic matter (OM) in soil across different locations: tree base, organic horizon (O) and A horizon (A). For the O and A horizons, data are presented for both burned and unburned areas. Note that soil samples from the tree base were collected from unburned sites exclusively. The central line within each box represents the median OM concentration, whereas the edges of the box denote the first and third quartiles (Q1 and Q3). The whiskers extend to the smallest and largest values within 1.5 times the interquartile range (IQR). Outliers beyond this range are marked as individual points.
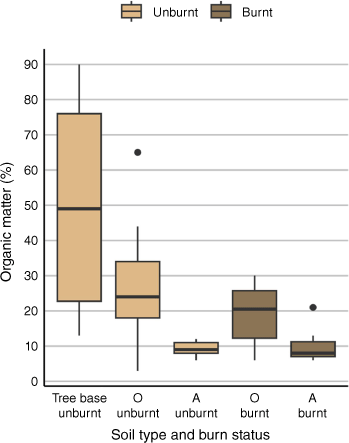
The pattern of Hg distribution in burned soils followed the same pattern as in unburned soils. Mean total Hg concentrations in burned soils were highest in the O horizon soils, followed by A horizon soils, (91 ± 63 and 46 ± 19 ng g−1 respectively) (Table 1). A mixed linear model was used to test for significant differences in Hg concentrations between burned and unburned soil horizons (Supplementary Table S7). This model found no statistically significant difference in Hg concentration between burned and unburned soils, or for the interaction between burning and soil horizon type, but it did find a significant difference between Hg concentrations in O and A horizon soils (t22.93 = 1.06, t15.95 = −0.15, t15.46 = 5.25) (Supplementary Table S7). Post hoc pairwise comparison testing with estimated marginal means revealed a significant difference between burned O (91 ± 63 ng g−1) and A (46 ± 19 ng g−1) horizon soils (t16.0 = −5.251, P < 0.0005). A significant difference was also revealed between unburned O (112 ± 71 ng g−1) and A (56 ± 30 ng g−1) horizon soils (t16.9 = −4.550, P < 0.005) (Supplementary Table S7). No significant difference was found between burned and unburned soils collected from the same horizon (Supplementary Table S7).
Similar to Hg, fire had no effect on the OM content in soil. Overall, O horizon soils from both burned and unburned areas had a higher mean OM content (19 ± 9 and 28 ± 18% respectively) than A horizon soils (10 ± 5 and 9 ± 2% respectively) (Table 1). Median OM content followed the same pattern (24, 21, 9, 8% respectively) (Table 1, Fig. 3). A linear mixed effects model was used to test for significant differences between soil horizons by treatment (Supplementary Table S7). The model revealed a significant effect for soil horizon, but no effect for burning or for the interaction between burning and horizon (t16.78 = 2.86, t32.43 = 0.06, t17.76 = 0.84) (Supplementary Table S7). Post hoc pairwise testing with estimated marginal means revealed significant differences in OM content between burned O horizon soils and burned A horizon soils (t16.3 = −2.861, P < 0.05), and unburned O horizon soils and unburned A horizon soils (t18.1 = −3.797, P < 0.05) (Supplementary Table S7).
Pearson correlations were calculated between organic matter content and mercury concentration for all soil types. Since no significant difference in Hg concentrations was observed between burned and unburned sites, correlation analyses were performed using all soil samples combined (burned and unburned) to increase the statistical power of the analysis.
A positive correlation was observed between OM and Hg concentrations across the study sites. The regression analysis yielded a significant relationship (P < 0.05, Fig. 4, Supplementary Table S8), with the model for all samples combined explaining 60% of the variance in Hg concentrations (R2 = 0.6). This indicates that sites with higher OM content tend to have higher Hg concentrations.
Mercury concentrations in litter
The litter samples collected from both burned and unburned sites consisted of unburned leaves, twigs and bark that either remained unburned or had fallen after the fire. Consequently, all litter samples from burned and unburned sites in this study were combined, and no statistical analyses were performed to differentiate between the two prescribed burning contexts.
Mean Hg concentrations in litter were highest in leaves, followed by bark and twigs (71 ± 11, 21 ± 13, 7.5 ± 3.9 ng g−1 respectively). Median Hg concentrations followed the same pattern (69, 16, 6.3 ng g−1 respectively) (Table 2, Fig. 5).
Median Hg concentrations (ng g−1) in bark, leaf and twig litter components. The central line within each box represents the median Hg concentration, whereas the edges of the box denote the first and third quartiles (Q1 and Q3). The whiskers extend to the smallest and largest values within 1.5 times the interquartile range (IQR). Outliers beyond this range are marked as individual points.
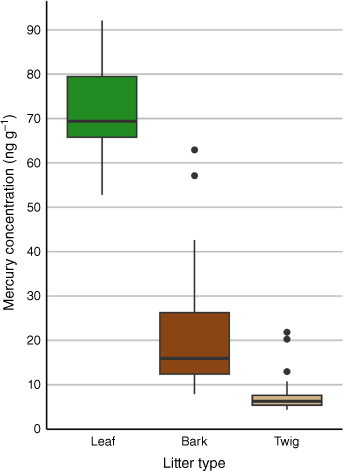
A significant difference in Hg concentrations between litter types was identified using a Kruskall–Wallis test (χ2(2) = 69.6, P < 0.0001). Post hoc Dunn testing found significant differences between bark and leaf litter, bark and twig litter, and leaf and twig litter (Z = −3.8, P ≤ 0.0001; Z = 5.1, P ≤ 0.0001; Z = 8.1, P ≤ 0.0001 respectively) (Supplementary Table S9).
Calculated emissions from the prescribed burn
The Hg burden in litter in unburned areas is 374 g Hg ha−1 (Table 3). The emission factors for biomass burning (EFbio), calculated for each type of biomass, are shown in Table 3. Soil Hg emissions were not calculated, as the results showed no significant difference in Hg concentrations between burned and unburned areas. Based on the estimated total burned area of 290 ha, the EFbio was calculated as 0.25 g Hg ha−1 for the entire burned area.
Litter type | DB (Mg ha−1) | CHg (ng g−1) | Mb (g ha−1) | CC (%) | EFbio (ng g−1) burned biomass | EFbio (g ha−1) | EFbio (g/290 ha) | |
---|---|---|---|---|---|---|---|---|
Leaf | 3.62 | 71 | 257 | 66 | 13.6 | 0.170 | 49.3 | |
Bark | 3.5 | 21 | 73.5 | 66 | 3.9 | 0.049 | 14.2 | |
Twig | 5.38 | 8 | 43.0 | 66 | 2.3 | 0.028 | 8.1 | |
Total | 12.5 | 374 | 19.8 | 0.247 | 71.6 |
The Hg emission factor for the total area burned is also shown. For Mb, the total area burned was 290 ha and Mb = DB × CHg.
Discussion
This study primarily aimed to understand the Hg pool in a sclerophyll forest in Victoria, Australia. Additionally, it opportunistically examined the impact of a prescribed burn, offering a general overview of Hg emissions to contribute to the limited knowledge of Hg release from prescribed forest burning in Australia.
The results of Hg emissions from burning in this study should be interpreted with caution due to the following limitations:
Ash samples post-burning were not collected, requiring the assumption that all Hg present in the samples was emitted.
Mercury emissions may have been underestimated, as calculations were based on the loss of litter mass, potentially missing Hg released from remaining material due to heat exposure since biomass calculations included ash as part of the non-combusted material.
Biomass estimates were derived from similar forests within the same geographic context, but not from the exact forest in this study, introducing a possible margin of error into the calculations.
Mercury concentrations in bulk soil and near trees of sclerophyll forest
Our study revealed a wide range of Hg concentrations in soils of sclerophyll forest, with 143 ± 62 ng g−1 measured at the base of trees, 100 ± 65 ng g−1 in the organic layer and 51 ± 25 ng g−1 in the mineral layer. These concentrations are among the highest recorded in Victoria (Table 4). For comparison, soil Hg concentrations in Pumphouse, central Victoria, were measured at 29.4 and 25.3 ng g−1 in the topsoil (0–2 cm) and lower (5–10 cm) soil layers respectively (Howard et al. 2019). In the Latrobe Valley, located south of our study site, the mean soil Hg concentration was reported as 53.5 ± 25.7 ng g−1 (Schneider et al. 2021). Similarly, in a study conducted in Victoria, a comparable mercury concentration of 90 ng g−1 was documented (Packham et al. 2009).
Depth (cm) | Hg (ng g−1) | Study | Vegetation type | Sample treatment | Analytical method | |
---|---|---|---|---|---|---|
Queensland | ||||||
0–10 | 42 ± 30 | Barry (1997) | Vegetable paddock – horticulture | Sieved to <2000 µm then ground to <50 µm | Cold vapour (CV) atomic absorption spectroscopy (AAS) | |
10–20 | 69 ± 33 | |||||
20–30 | 68 ± 32 | |||||
0–100 | 64 ± 76 | Turull et al. (2018) | Basin soils – vegetation not mentioned | Not sieved | AAS (using a AMA-254 instrument) | |
100–200 | 77 ± 104 | |||||
200–300 | 52 ± 42 | |||||
New South Wales | ||||||
0–10 | 80 ± 30 | Rayment et al. (1998) | Grassed paddock | Not sieved | CV-AAS (following HCl/HNO3 digest) | |
0–25 | 80 ± 40 | |||||
25–30 | 60 ± 10 | |||||
0–5 | 18.2 ± 1.1 | Schneider et al. (2021) | Sclerophyll forest | Not sieved | AAS (using a DMA-80 instrument) | |
Victoria | ||||||
0–10 | 61 ± 80 | Reimann and de Caritat (2017) | Mixed | Sieved to <75 µm | Inductively coupled plasma (ICP) mass spectroscopy (MS) (following aqua regia digest) | |
60–80 | 34 ± 34 | Sieved to <75 µm | ||||
Not reported | 90 | Packham et al. (2009) | Not reported | Not reported | CV-ICP-Optical emission spectroscopy | |
0–5 | 53.5 ± 25.7 | Schneider et al. (2021) | Sclerophyll forest | Not sieved | AAS (DMA-80) | |
0–2 | 29.4 ± 17.7 | Howard et al. (2019) | Dry Sclerophyll | Not reported | AAS (DMA-80) | |
5–10 | 25.3 ± 11.8 | |||||
O horizon | 100 ± 65 | This study | Wet Sclerophyll | Sieved to <63 µm | AAS (DMA-80) | |
A horizon | 51 ± 25 | |||||
Tasmania | ||||||
0–10 | 366 ± 1301 | Reimann and de Caritat (2017) | Mixed | Sieved to <75 µm | Same as Reimann and de Caritat (2017) above | |
60–80 | 69 ± 124 |
Reimann and de Caritat (2017) sampled across Australia; however, only the results for Victoria and Tasmania are given here.
Soils representing environmental background Hg concentrations were sampled around Glenbawn Lake in New South Wales, measuring at 18.2 ± 1.1 ng g−1 (Schneider et al. 2021). By comparison, background Hg concentrations in coastal soils from Queensland and New South Wales have been reported to range from 10 to 50 ng g−1 (Rayment et al. 1998). When comparing our findings to a broader study encompassing the entirety of Australia, our results are consistent with those of Reimann and de Caritat (2017), who documented the highest median Hg concentrations (~40–50 ng g−1) in Victoria and Tasmania relative to other Australian states. However, caution should be exercised when comparing our data with other studies in Australia, as different analytical methods were employed. Additionally, our study focused on the <63-µm soil fraction, whereas other studies analysed bulk soil (Table 4).
Another important consideration regarding the higher concentrations found in this study is the forested area and type of soil where the samples were collected. In forest ecosystems, soil Hg concentrations are generally higher than in open terrestrial ecosystems due to the efficiency of forest canopies in trapping atmospheric Hg (Wang X et al. 2019; Zhou et al. 2021). Broadleaf evergreen forests have been shown to be particularly effective at transferring atmospheric Hg (in part due to the long lifetime of leaves), and consequently at transferring atmospheric Hg to the soil through litterfall deposition, resulting in higher soil Hg concentrations compared to deciduous forests (Wang X et al. 2016b; Chen et al. 2024). Although the role of wet sclerophyll forests in the Hg cycle has been understudied, it is likely that forest structure similarly contributes to increased atmospheric Hg uptake, thereby elevating Hg concentrations in the soil. Furthermore, the soils in the wet sclerophyll forests of Victoria are predominantly loamy (Gullan 2017), a soil type known for its high efficiency in retaining Hg. This is because Hg, like other trace elements, tends to concentrate on the surface of finer particles, such as silt and clay (Ravichandran et al. 1995; Boszke et al. 2004).
The Hg concentrations observed in this study exceed previously published data for Australian soils (Table 4), yet they are consistent with the typical range found in uncontaminated podzol–spodozol soils from temperate and boreal regions (Grigal 2003; Obrist et al. 2011; Kronberg et al. 2016; Gómez-Armesto et al. 2020). The wide range of variance in Hg concentrations across the sites within the sclerophyll forest is likely attributable to variations in environmental factors that influence soil organic matter content and atmospheric Hg deposition rates.
Vertical mercury distribution in soils
The vertical distribution of Hg in sclerophyll forest soils in this study closely resembles the patterns observed in temperate and boreal forests, with greater accumulation in the surface layer where Hg is predominantly bound to OM (Schwesig and Matzner 2000; Grigal 2003; Obrist et al. 2016; Landis et al. 2024). The positive correlation between the Hg and OM content supports this hypothesis. The higher Hg concentrations observed in the O horizon compared to the A horizon can therefore be directly linked to the elevated OM content in the surface organic layer. In forest ecosystems, the decomposition of litterfall enriches the superficial soil in OM. The thickness and composition of the O horizon is driven by multiple factors including climate, soil properties, microtopography, pedoturbation and decomposition of large biomass components (Obrist et al. 2016; Wang X et al. 2019; Landis et al. 2024).
In the studied sclerophyll forest, the combination of high humidity during winter and relatively low temperatures facilitates the accumulation of OM in the surface soil. Wet sclerophyll forests typically have deep litter layers and very large trees (Ashton 1975) compared to other Australian forest types. The litter on the forest floor is made up of portions of fern fronds, large leathery leaves from shrubs and eucalypts, twigs, branches and strips of bark. None of these break down quickly, despite the usually moist conditions at ground level, so the litter and the organic soil beneath it becomes very deep and friable.
This indicates that sites with higher OM content tend to have higher Hg concentrations, indicating that OM plays a key role in Hg retention and accumulation in soils of sclerophyll forest in Victoria. The positive correlation between Hg and OM in our study is likely a result of Hg’s strong affinity for OM due to its ability to form stable complexes with organic ligands (Gabriel and Williamson 2004, Ravichandran 2004). The binding of Hg to OM is a well-documented process that facilitates its retention in soil and sediment matrices (Grigal 2003; Miretzky et al. 2005; Skyllberg 2010). The vertical distribution profiles of Hg and OM are often closely linked, with higher concentrations typically found in surface soil. This relationship is commonly observed in podzol soils of boreal and temperate regions where the highest Hg concentrations generally occur in the uppermost soil horizon (Grigal 2003; Obrist et al. 2016) – a pattern that is also evident in our study. The relationship between Hg and OM is often used to assess Hg dynamics in soil (Grigal 2003).
In our study, the amount of Hg in OM was calculated as 0.28 µg Hg g−1 OM at the tree base, 0.45 µg Hg g−1 OM in the O horizon, and 0.56 in the A horizon. For surface organic soils of temperate and boreal forests an average of 0.22 µg Hg g−1 OM was reported by Grigal (2003). An increasing stoichiometry is also frequently observed with depth, which is attributed to less loss of Hg than C during the mineralisation of OM (Grigal 2003).
Mercury in soil at the base of trees
Mercury concentrations at the base of trees were significantly higher than in soil samples taken in between trees. This difference is likely related to the higher OM content also observed in the tree base region. Both the increased OM content and elevated Hg concentrations near the tree base that has high root density have been linked to the activity of soil fungi mycelium (Read and Perez-Moreno 2003). Previous studies have demonstrated that arbuscular mycorrhizal fungi can enhance Hg sorption in soil, suggesting that mycorrhizal associations may play a role in the higher Hg accumulation observed at tree bases (Liu et al. 2010).
Further work is needed to explore the extent of regions with elevated OM and Hg concentrations around the base of trees in sclerophyll forests of Australia. Not only is this information needed for accurately estimating Hg emissions from prescribed burns, but it is also integral in assessing wildfire emissions, which may significantly affect Hg concentrations in soils.
Mercury concentrations in litter
The mean Hg concentrations in leaf litter, bark and twigs from this study are in good agreement with the few data previously published for Eucalyptus in Victoria (Table 5). The mean Hg concentration in leaves in this study is 64 ± 9 ng g−1; this value exceeds both the global mean (54 ± 22 ng g−1) and median (46 ng g−1) reported by Wang X et al. (2016b) across 168 forest sites worldwide.
Study | Localisation | Leaves | Bark | Twigs | Litter | Analytical method | |
---|---|---|---|---|---|---|---|
Howard et al. (2019) | Pumphouse, Central Victoria | 72.9 ± 10.9 | 25.0 ± 11.1 | 10.1 ± 5.4 | AAS (DMA-80) | ||
Hellings et al. (2013) | Holey Plains State Park (south-eastern Victoria) | 78.5 ± 2.1 | 50.1 ± 2.5 | CV-ICP-OES | |||
Packham et al. (2009) | South-eastern Victoria | 83 | CV-ICP-OES | ||||
This study | Boola Boola State Forest | 71 ± 11 | 21 ± 13 | 8 ± 4 | AAS (DMA-80) |
Mercury concentrations in both foliage and litter are often strongly correlated with atmospheric Hg levels. In vascular plants, ~90% of the Hg found in leaves and needles is reported to be derived from the atmospheric uptake of gaseous elemental Hg (Zhou et al. 2021). Besides atmospheric Hg concentration, various environmental factors such as solar irradiation, air temperature, altitude and biological factors, including plant species, leaf age and leaf position, significantly influence Hg uptake by foliage (Ericksen et al. 2003; Blackwell and Driscoll 2015; Zhou et al. 2016).
Given that atmospheric Hg concentrations in Australia are relatively low compared to global averages (United Nations Environment Programme and Arctic Monitoring and Assessment Programme 2019; Fisher and Nelson 2020), the elevated Hg concentrations observed in Eucalyptus species may be attributed to unique biological factors, linked to the unique climate and soils in Australia. This influence of biological factors on Hg uptake is exemplified by studies reporting elevated Hg concentrations in evergreen broadleaf forest biomes (Wang X et al. 2016a; Zhou and Obrist 2021). Notably, the highest Hg concentrations in leaves and litterfall have been recorded in the Amazonian tropical forest, with mean and median concentrations of ~70 ng g−1 (Fostier et al. 2015; Wang X et al. 2016a), and in the subtropical evergreen Atlantic Forest, where annual concentrations range from 167 to 334 ng g−1, with a mean of 238 ± 52 ng g−1 (Teixeira et al. 2012). Eucalypt forests are also evergreen and broadleaf. There is a critical need for further research on Hg dynamics in Eucalyptus species in Australia, as well as a deeper understanding of atmospheric Hg patterns across the country.
Emission factor
For the burned litter, the overall Hg EF (19.8 ng Hg g−1 burned litter) was low when compared to EF for wildfires, which range from 315 ng Hg g−1 for Boreal and Equatorial Asia to 41 ng Hg g−1 for grasslands (Kumar et al. 2018). However, it is important to note that the intensity of wildfires is usually much higher than that of prescribed fires, and thus results in soil Hg emissions which were not observed in the present study. Conversely, our estimate is in the same range as the EF previously reported by Howard et al. (2019) (28.7 ± 8.1 ng g−1) as the upper estimate of Hg emission from Australian dry sclerophyll surface fuels for wildfires. During forest fire, Hg is mainly emitted as gaseous elemental mercury (GEM) and particulate bound mercury (PBM), however, many factors can influence their proportion such as moisture and fuel nature and fire intensity (Obrist et al. 2018). Furthermore, part of GEM can be absorbed on ash and PBM is easily deposited at short distance (De Simone et al. 2017; Richter et al. 2023), therefore limiting long distance atmospheric transport. However, fate and transport of Hg emitted during biomass burning are understudied and more work needs to be done to improve this understanding.
Future directions
To improve our understanding of Hg emissions from forest burning in sclerophyll ecosystems, future research should address several key areas:
The collection and analysis of ash samples are essential to accurately quantify Hg emissions. Without this data, assumptions about total Hg release may lead to over- or underestimation. To provide robust data that can inform policy decisions, future studies should incorporate ash sampling as a standard procedure in post-burn analyses. This will ensure a more accurate quantification of Hg emissions and reduce the uncertainties associated with current estimates.
More precise measurements of biomass loss and heat effects on remaining materials are necessary. Current estimates based solely on litter mass loss may miss Hg that is released from non-combusted material exposed to high temperatures. For example, this could include litter under fallen logs or litter adjacent to a burnt area. Including a more detailed assessment of partially combusted, and non-combusted, yet heated, material could improve estimates of Hg emissions.
Future studies should focus on collecting biomass data before and after fire from the exact study sites to reduce uncertainties in estimates of biomass loss and proportions of components. Although data from similar forests in the same geographic region are useful, site-specific measurements would reduce the margin of error and provide more accurate Hg emission factors. Expanding this research to include multiple forest types and burn intensities across Australia will further strengthen our understanding of Hg cycling in these ecosystems.
Fate and transport of Hg emitted during (and after) forest fires needs to be further studied to better assess the real contribution of biomass burning into global Hg emissions.
Future directions for this research should involve Indigenous fire management practices, which emphasise low-intensity burns aimed at ecological conservation. These practices may result in lower Hg emissions compared to a higher intensity burn, due to reduced combustion of organic material. By investigating the relationship between low-intensity burns and Hg mobility, we can gain valuable insights into sustainable land management and enhance understanding of these practices within the Hg cycle.
By addressing these gaps, future research can enhance the precision of Hg emission estimates and contribute to a more comprehensive understanding of Hg dynamics in Australian forests. The relatively high Hg concentrations found in litter leaves, highlights the need for further research on Hg uptake mechanisms in Australian sclerophyll forests.
Data availability
The data that support this study are available in the article and accompanying online supplementary material.
Declaration of funding
The fieldwork was funded under ‘Lost Mines: The Troubled Legacies of Former Mining Landscapes’ (ARC DP220101967), which was awarded to P. Davies and S. Lawrence. The laboratory work was funded under ‘A long term history of mercury in Australasia’ (ARC DE180100573), which was awarded to L. Schneider.
Acknowledgements
The authors acknowledge the Gunaikurnai people, the Traditional Custodians of the land sampled for this study.
References
Amirbahman A, Ruck PL, Fernandez IJ, Haines TA, Kahl JS (2004) The effect of fire on mercury cycling in the soils of forested watersheds: Acadia National Park, Maine, USA. Water, Air, and Soil Pollution 152, 315-331.
| Crossref | Google Scholar |
Andreae M, Merlet P (2001) Emission of trace gases and aerosols from biomass burning. Global Biogeochemical Cycles 15(4), 955-966.
| Crossref | Google Scholar |
Artaxo P, Calixto de Campos R, Fernandes ET, Martins JV, Xiao Z, Lindqvist O, Fernández-Jiménez MT, Maenhaut W (2000) Large scale mercury and trace element measurements in the Amazon Basin. Atmospheric Environment 34, 4085-4096.
| Crossref | Google Scholar |
Ashton D (1975) Studies in litter in Eucalyptus regnans forests. Australian Journal of Botany 23, 413-433.
| Crossref | Google Scholar |
Barry G (1997) Total heavy metal status of horticultural soils in Queensland. (Horticultural Research and Development Corporation: Sydney, NSW, Australia) Available at https://ausveg.com.au/app/data/technical-insights/docs/VG404.pdf
Basu N, Bastiansz A, Dórea JG, Fujimura M, Horvat M, Shroff E, Weihe P, Zastenskaya I (2023) Our evolved understanding of the human health risks of mercury. Ambio 52, 877-896.
| Crossref | Google Scholar |
Bates D, Mächler M, Bolker B, Walker S (2015) Fitting linear mixed-effects models using lme4. Journal of Statistical Software 67(1), 1-48.
| Crossref | Google Scholar |
Biswas A, Blum JD, Klaue B, Keeler GJ (2007) Release of mercury from Rocky Mountain forest fires. Global Biogeochemical Cycles 21, GB1002.
| Crossref | Google Scholar |
Blackwell BD, Driscoll CT (2015) Using foliar and forest floor mercury concentrations to assess spatial patterns of mercury deposition. Environmental Pollution 202, 126-134.
| Crossref | Google Scholar |
Boszke L, Kowalski A, Siepak J (2004) Grain size partitioning of mercury in sediments of the middle Odra river (Germany/Poland). Water, Air, and Soil Pollution 159, 125-138.
| Crossref | Google Scholar |
Chen L, Zhou J, Guo L, Bian X, Xu Z, Chen Q, Wen S-H, Wang K, Liu Y-R (2024) Global distribution of mercury in foliage predicted by machine learning. Environmental Science & Technology 58, 15629-15637.
| Crossref | Google Scholar |
Cobbett FD, Van Heyst BJ (2007) Measurements of GEM fluxes and atmospheric mercury concentrations (GEM, RGM and Hgp) from an agricultural field amended with biosolids in southern Ont., Canada (October 2004–November 2004). Atmospheric Environment 41, 2270-2282.
| Crossref | Google Scholar |
De Simone F, Artaxo P, Bencardino M, Cinnirella S, Carbone F, D’Amore F, Dommergue A, Feng XB, Gencarelli CN, Hedgecock IM, Landis MS, Sprovieri F, Suzuki N, Wängberg I, Pirrone N (2017) Particulate-phase mercury emissions from biomass burning and impact on resulting deposition: a modelling assessment. Atmospheric Chemistry and Physics 17, 1881-1899.
| Crossref | Google Scholar |
Dittman JA, Shanley JB, Driscoll CT, Aiken GR, Chalmers AT, Towse JE, Selvendiran P (2010) Mercury dynamics in relation to dissolved organic carbon concentration and quality during high flow events in three northeastern US streams. Water Resources Research 46, W07522.
| Crossref | Google Scholar |
Ericksen JA, Gustin MS (2004) Foliar exchange of mercury as a function of soil and air mercury concentrations. Science of The Total Environment 324, 271-279.
| Crossref | Google Scholar |
Ericksen JA, Gustin MS, Schorran DE, Johnson DW, Lindberg SE, Coleman JS (2003) Accumulation of atmospheric mercury in forest foliage. Atmospheric Environment 37, 1613-1622.
| Crossref | Google Scholar |
Feinberg A, Dlamini T, Jiskra M, Shah V, Selin NE (2022) Evaluating atmospheric mercury (Hg) uptake by vegetation in a chemistry-transport model. Environmental Science: Processes & Impacts 24, 1303-1318.
| Crossref | Google Scholar |
Filimonenko E, Vatutin G, Zherebyatyeva N, Uporova M, Milyaev I, Chausоva E, Gershelis E, Alharbi SA, Samokhina N, Matus F, Soromotin A, Kuzyakov Y (2024) Wildfire effects on mercury fate in soils of north-western Siberia. Science of The Total Environment 951, 175572.
| Crossref | Google Scholar |
Fisher JA, Nelson PF (2020) Atmospheric mercury in Australia: recent findings and future research needs. Elementa: Science of the Anthropocene 8, 070.
| Crossref | Google Scholar |
Fisher JA, Schneider L, Fostier A-H, Guerrero S, Guimarães JRD, Labuschagne C, Leaner JJ, Martin LG, Mason RP, Somerset V, Walters C (2023) A synthesis of mercury research in the Southern Hemisphere, part 2: anthropogenic perturbations. Ambio 52, 918-937.
| Crossref | Google Scholar |
Fitzgerald WF (1995) Is mercury increasing in the atmosphere? The need for an atmospheric mercury network (AMNET). Water, Air, and Soil Pollution 80, 245-254.
| Crossref | Google Scholar |
Fostier AH, Melendez-Perez JJ, Richter L (2015) Litter mercury deposition in the Amazonian rainforest. Environmental Pollution 206, 605-610.
| Crossref | Google Scholar |
Francisco López A, Heckenauer Barrón EG, Bello Bugallo PM (2022) Contribution to understanding the influence of fires on the mercury cycle: systematic review, dynamic modelling and application to sustainable hypothetical scenarios. Environmental Monitoring and Assessment 194, 707.
| Crossref | Google Scholar |
Friedli HR, Arellano AF, Cinnirella S, Pirrone N (2009) Initial estimates of mercury emissions to the atmosphere from global biomass burning. Environmental Science & Technology 43, 3507-3513.
| Crossref | Google Scholar |
Gabriel MC, Williamson DG (2004) Principal biogeochemical factors affecting the speciation and transport of mercury through the terrestrial environment. Environmental Geochemistry and Health 26, 421-434.
| Crossref | Google Scholar |
Gómez-Armesto A, Méndez-López M, Pérez-Rodríguez P, Fernández-Calviño D, Arias-Estévez M, Nóvoa-Muñoz JC (2020) Litterfall Hg deposition to an oak forest soil from southwestern Europe. Journal of Environmental Management 269, 110858.
| Crossref | Google Scholar |
Grigal DF (2003) Mercury sequestration in forests and peatlands. Journal of Environmental Quality 32, 393-405.
| Crossref | Google Scholar |
Gullan P (2017) Victorian ecosystems: wet sclerophyll forest. (Viridans Biological Databases) Available at https://www.viridans.com/ECOVEG/wet%20sclerophyll.htm
Gustin MS, Engle M, Ericksen J, Xin M, Krabbenhoft D, Lindberg S, Olund S, Rytuba J (2005) New insights into mercury exchange between air and substrate. In ‘Goldschmidt Conference Abstracts 2005: the Geochemistry of Mercury’, 21–25 May 2005, Moscow, ID, USA. p. A700. (Geochemical Society and of the European Association of Geochemistry) Available at https://goldschmidtabstracts.info/2005/700.pdf
Gustin MS, Lindberg SE, Weisberg PJ (2008) An update on the natural sources and sinks of atmospheric mercury. Applied Geochemistry 23, 482-493.
| Crossref | Google Scholar |
Hall BD, St Louis VL (2004) Methylmercury and total mercury in plant litter decomposing in upland forests and flooded landscapes. Environmental Science & Technology 38, 5010-5021.
| Crossref | Google Scholar |
Heise-Pavlov S, Procter-Gray E (2021) Chapter 7 – How an understanding of Lumholtz’s tree kangaroo behavioral ecology can assist conservation. In ‘Tree Kangaroos: Science and Conservation. Biodiversity of World: Conservation from Genes to Landscapes’. (Eds L Dabek, P Valentine, J Blessington, KR Schwartz). pp. 85–107. (Academic Press) 10.1016/B978-0-12-814675-0.00028-2
Hellings J, Adeloju SB, Verheyen TV (2013) Rapid determination of ultra-trace concentrations of mercury in plants and soils by cold vapour inductively coupled plasma–optical emission spectrometry. Microchemical Journal 111, 62-66.
| Crossref | Google Scholar |
Howard D, Macsween K, Edwards GC, Desservettaz M, Guérette E-A, Paton-Walsh C, Surawski NC, Sullivan AL, Weston C, Volkova L, Powell J, Keywood MD, Reisen F, Mick Meyer CP (2019) Investigation of mercury emissions from burning of Australian eucalypt forest surface fuels using a combustion wind tunnel and field observations. Atmospheric Environment 202, 17-27.
| Crossref | Google Scholar |
Jiskra M, Sonke JE, Obrist D, Bieser J, Ebinghaus R, Myhre CL, Pfaffhuber KA, Wängberg I, Kyllönen K, Worthy D, Martin LG, Labuschagne C, Mkololo T, Ramonet M, Magand O, Dommergue A (2018) A vegetation control on seasonal variations in global atmospheric mercury concentrations. Nature Geoscience 11, 244-250.
| Crossref | Google Scholar |
Johnson DW, Lindberg SE (1995) The biogeochemical cycling of Hg in forests: alternative methods for quantifying total deposition and soil emission. Water, Air, and Soil Pollution 80, 1069-1077.
| Crossref | Google Scholar |
Kronberg R-M, Jiskra M, Wiederhold JG, Björn E, Skyllberg U (2016) Methyl mercury formation in hillslope soils of boreal forests: the role of forest harvest and anaerobic microbes. Environmental Science & Technology 50, 9177-9186.
| Crossref | Google Scholar |
Kumar A, Wu S, Huang Y, Liao H, Kaplan JO (2018) Mercury from wildfires: global emission inventories and sensitivity to 2000–2050 global change. Atmospheric Environment 173, 6-15.
| Crossref | Google Scholar |
Kuznetsova A, Brockhoff PB, Christensen RHB (2017) lmerTest package: tests in linear mixed effects models. Journal of Statistical Software 82(13), 1-26.
| Crossref | Google Scholar |
Landis JD, Obrist D, Zhou J, Renshaw CE, McDowell WH, Nytch CJ, Palucis MC, Del Vecchio J, Montano Lopez F, Taylor VF (2024) Quantifying soil accumulation of atmospheric mercury using fallout radionuclide chronometry. Nature Communications 15, 5430.
| Crossref | Google Scholar |
Lin C-J, Pehkonen SO (1999) The chemistry of atmospheric mercury: a review. Atmospheric Environment 33, 2067-2079.
| Crossref | Google Scholar |
Lindqvist O, Johansson K, Bringmark L, Timm B, Aastrup M, Andersson A, Hovsenius G, Håkanson L, Iverfeldt Å, Meili M (1991) Mercury in the Swedish environment – recent research on causes, consequences and corrective methods. Water, Air, and Soil Pollution 55, xi-261.
| Crossref | Google Scholar |
Liu Y-R, Zheng Y-M, Shen J-P, Zhang L-M, He J-Z (2010) Effects of mercury on the activity and community composition of soil ammonia oxidizers. Environmental Science and Pollution Research 17, 1237-1244.
| Crossref | Google Scholar |
Miretzky P, Bisinoti MC, Jardim WF, Rocha JC (2005) Factors affecting Hg(II) adsorption in soils from the Rio Negro basin (Amazon). Química Nova 28, 438-443.
| Crossref | Google Scholar |
Morgan GW, Tolhurst KG, Poynter MW, Cooper N, McGuffog T, Ryan R, Wouters MA, Stephens N, Black P, Sheehan D, Leeson P, Whight S, Davey SM (2020) Prescribed burning in south-eastern Australia: history and future directions. Australian Forestry 83, 4-28.
| Crossref | Google Scholar |
Neumann M, Turner J, Lewis T, McCaw L, Cook G, Adams MA (2021) Dynamics of necromass in woody Australian ecosystems. Ecosphere 12, e03693.
| Crossref | Google Scholar |
NSW Rural Fire Service (2005) Standards for low intensity bush fire hazard reduction burning (for private landholders). (NSW Rural Fire Service: Sydney, NSW, Australia) Available at https://www.rfs.nsw.gov.au/__data/assets/pdf_file/0011/13322/Standards-for-Low-Intensity-Bush-Fire-Hazard-Reduction-Burning.pdf
Obrist D (2007) Atmospheric mercury pollution due to losses of terrestrial carbon pools? Biogeochemistry 85, 119-123.
| Crossref | Google Scholar |
Obrist D, Johnson DW, Lindberg SE, Luo Y, Hararuk O, Bracho R, Battles JJ, Dail DB, Edmonds RL, Monson RK, Ollinger SV, Pallardy SG, Pregitzer KS, Todd DE (2011) Mercury distribution across 14 US forests. Part I: spatial patterns of concentrations in biomass, litter, and soils. Environmental Science & Technology 45, 3974-3981.
| Crossref | Google Scholar |
Obrist D, Pearson C, Webster J, Kane T, Lin C-J, Aiken GR, Alpers CN (2016) A synthesis of terrestrial mercury in the western United States: spatial distribution defined by land cover and plant productivity. Science of The Total Environment 568, 522-535.
| Crossref | Google Scholar |
Obrist D, Kirk JL, Zhang L, Sunderland EM, Jiskra M, Selin NE (2018) A review of global environmental mercury processes in response to human and natural perturbations: changes of emissions, climate, and land use. Ambio 47, 116-140.
| Crossref | Google Scholar |
Obrist D, Roy EM, Harrison JL, Kwong CF, Munger JW, Moosmüller H, Romero CD, Sun S, Zhou J, Commane R (2021) Previously unaccounted atmospheric mercury deposition in a midlatitude deciduous forest. Proceedings of the National Academy of Sciences 118, e2105477118.
| Crossref | Google Scholar |
Outridge PM, Mason RP, Wang F, Guerrero S, Heimbürger-Boavida LE (2018) Updated global and oceanic mercury budgets for the United Nations global mercury assessment 2018. Environmental Science & Technology 52, 11466-11477.
| Crossref | Google Scholar |
Packham D, Tapper N, Griepsma D, Friedli H, Hellings J, Harris S (2009) Release of mercury from biomatter after burning: Release of mercury in the Australian environment by burning: a preliminary investigation of biomatter and soils. Air Quality and Climate Change 43, 24-27.
| Google Scholar |
Pokharel AK, Obrist D (2011) Fate of mercury in tree litter during decomposition. Biogeosciences 8, 2507-2521.
| Crossref | Google Scholar |
Ravichandran M (2004) Interactions between mercury and dissolved organic matter––a review. Chemosphere 55, 319-331.
| Crossref | Google Scholar |
Ravichandran M, Baskaran M, Santschi PH, Bianchi TS (1995) History of trace metal pollution in Sabine–Neches Estuary, Beaumont, Texas. Environmental Science & Technology 29, 1495-1503.
| Crossref | Google Scholar |
Rayment GE, Jeffrey AJ, Barry GA (1998) Heavy metals in New South Wales canelands. Proceedings of the Australian Society of Sugar Cane Technologists 20, 63-68.
| Google Scholar |
Read DJ, Perez-Moreno J (2003) Mycorrhizas and nutrient cycling in ecosystems – a journey towards relevance? New Phytologist 157, 475-492.
| Crossref | Google Scholar |
Reimann C, de Caritat P (2017) Establishing geochemical background variation and threshold values for 59 elements in Australian surface soil. Science of The Total Environment 578, 633-648.
| Crossref | Google Scholar |
Richter L, Amouroux D, Tessier E, Fostier AH (2023) Impact of forest fire on the mercury stable isotope composition in litter and soil in the Amazon. Chemosphere 339, 139779.
| Crossref | Google Scholar |
Ryan PJ, McGarity JW (1983) The nature and spatial variability of soil properties adjacent to large forest eucalypts. Soil Science Society of America 47, 286-293.
| Crossref | Google Scholar |
Saiz-Lopez A, Travnikov O, Sonke JE, Thackray CP, Jacob DJ, Carmona-García J, Francés-Monerris A, Roca-Sanjuán D, Acuña AU, Dávalos JZ, Cuevas CA, Jiskra M, Wang F, Bieser J, Plane JMC, Francisco JS (2020) Photochemistry of oxidized Hg(I) and Hg(II) species suggests missing mercury oxidation in the troposphere. Proceedings of the National Academy of Sciences 117, 30949-30956.
| Crossref | Google Scholar |
Schneider L, Rose NL, Myllyvirta L, Haberle S, Lintern A, Yuan J, Sinclair D, Holley C, Zawadzki A, Sun R (2021) Mercury atmospheric emission, deposition and isotopic fingerprinting from major coal-fired power plants in Australia: insights from palaeo-environmental analysis from sediment cores. Environmental Pollution 287, 117596.
| Crossref | Google Scholar |
Schwesig D, Matzner E (2000) Pools and fluxes of mercury and methylmercury in two forested catchments in Germany. Science of The Total Environment 260, 213-223.
| Crossref | Google Scholar |
Shah V, Jacob DJ, Thackray CP, Wang X, Sunderland EM, Dibble TS, Saiz-Lopez A, Černušák I, Kellö V, Castro PJ, Wu R, Wang C (2021) Improved mechanistic model of the atmospheric redox chemistry of mercury. Environmental Science & Technology 55, 14445-14456.
| Crossref | Google Scholar |
Shi Y, Zhao A, Matsunaga T, Yamaguchi Y, Zang S, Li Z, Yu T, Gu X (2019) High-resolution inventory of mercury emissions from biomass burning in tropical continents during 2001–2017. Science of The Total Environment 653, 638-648.
| Crossref | Google Scholar |
Skyllberg U (2010) Chapter 13 - Mercury Biogeochemistry in Soils and Sediments. In ‘Developments in Soil Science. Vol. 34: Synchrotron-Based Techniques in Soils and Sediments’. (Eds B Singh, M Gräfe) pp. 379–410. (Elsevier) 10.1016/S0166-2481(10)34013-X
Skyllberg U, Qian J, Frech W, Xia K, Bleam WF (2003) Distribution of mercury, methyl mercury and organic sulphur species in soil, soil solution and stream of a boreal forest catchment. Biogeochemistry 64, 53-76.
| Crossref | Google Scholar |
Sun X, Wang Q, Ma H, Wang Z, Yang S, Zhao C, Xu L (2011) Effects of plant rhizosphere on mercury methylation in sediments. Journal of Soils and Sediments 11, 1062-1069.
| Crossref | Google Scholar |
Teixeira DC, Montezuma RC, Oliveira RR, Silva-Filho EV (2012) Litterfall mercury deposition in Atlantic forest ecosystem from SE – Brazil. Environmental Pollution 164, 11-15.
| Crossref | Google Scholar |
Turull M, Komarova T, Noller B, Fontàs C, Díez S (2018) Evaluation of mercury in a freshwater environment impacted by an organomercury fungicide using diffusive gradient in thin films. Science of The Total Environment 621, 1475-1484.
| Crossref | Google Scholar |
United Nations Environment Programme and Arctic Monitoring and Assessment Programme (2019) Technical background report to the global mercury assessment 2018. (Narayana Press: Gylling, Denmark) Available at https://www.unep.org/globalmercurypartnership/resources/report/technical-background-report-global-mercury-assessment-2018
United States Environmental Protection Agency (1998) Method 7473 (SW-846): mercury in solids and solutions by thermal decomposition, amalgamation, and atomic absorption spectrophotometry, revision 0. (US EPA: Washington, DC, USA) Available at https://www.epa.gov/sites/default/files/2015-07/documents/epa-7473.pdf
Volkova L, Weston C (2015) Carbon loss from planned fires in southeastern Australian dry Eucalyptus forests. Forest Ecology and Management 336, 91-98.
| Crossref | Google Scholar |
Volkova L, Roxburgh SH, Surawski NC, Meyer CP (Mick), Weston CJ (2019) Improving reporting of national greenhouse gas emissions from forest fires for emission reduction benefits: An example from Australia. Environmental Science & Policy 94, 49-62.
| Crossref | Google Scholar |
Wang D, Shi X, Wei S (2003) Accumulation and transformation of atmospheric mercury in soil. Science of The Total Environment 304, 209-214.
| Crossref | Google Scholar |
Wang J, Xiang Y, Tian X, Zhang C, Gong G, Xue J, Jiang T, Wang D, Wang Y (2022) Role of the rhizosphere of a flooding-tolerant herb in promoting mercury methylation in water-level fluctuation zones. Journal of Environmental Sciences 119, 139-151.
| Crossref | Google Scholar |
Wang Q, Li Y, Wang Y (2011) Optimizing the weight loss-on-ignition methodology to quantify organic and carbonate carbon of sediments from diverse sources. Environmental Monitoring and Assessment 174, 241-257.
| Crossref | Google Scholar |
Wang X, Lin C-J, Yuan W, Sommar J, Zhu W, Feng X (2016a) Emission-dominated gas exchange of elemental mercury vapor over natural surfaces in China. Atmospheric Chemistry and Physics 16, 11125-11143.
| Crossref | Google Scholar |
Wang X, Bao Z, Lin C-J, Yuan W, Feng X (2016b) Assessment of global mercury deposition through litterfall. Environmental Science & Technology 50, 8548-8557.
| Crossref | Google Scholar |
Wang X, Yuan W, Lin C-J, Zhang L, Zhang H, Feng X (2019) Climate and vegetation as primary drivers for global mercury storage in surface soil. Environmental Science & Technology 53, 10665-10675.
| Crossref | Google Scholar |
Zhang P, Zhang Y (2022) Earth system modeling of mercury using CESM2 – part 1: atmospheric model CAM6-Chem/Hg v1.0. Geoscientific Model Development 15, 3587-3601.
| Crossref | Google Scholar |
Zhou J, Obrist D (2021) Global mercury assimilation by vegetation. Environmental Science & Technology 55, 14245-14257.
| Crossref | Google Scholar |
Zhou J, Wang Z, Sun T, Zhang H, Zhang X (2016) Mercury in terrestrial forested systems with highly elevated mercury deposition in southwestern China: the risk to insects and potential release from wildfires. Environmental Pollution 212, 188-196.
| Crossref | Google Scholar |
Zhou J, Obrist D, Dastoor A, Jiskra M, Ryjkov A (2021) Vegetation uptake of mercury and impacts on global cycling. Nature Reviews Earth & Environment 2, 269-284.
| Crossref | Google Scholar |