Quantification of palladium-labelled nanoplastics algal uptake by single cell and single particle inductively coupled plasma mass spectrometry
Elizabeth C. Bair

A
B
C
Abstract
Plastic pollution is widespread and continues to be a major concern, both for the environment and human health. Identifying nanoplastics is challenging but is important to understand how they behave once in the environment. It is shown that a combination of single particle (SP) and single cell (SC) inductively coupled plasma mass spectrometry (ICP-MS) can be used to quantify nanoplastics on a per cell basis after exposure to algal cells.
The effects of plastic pollution on human health and the environment are not well known but there are significant concerns. Although research has increased in recent years, there remain many obstacles to the quantification of nanoplastics. This rapid communication demonstrates that combined single particle (SP)– and single cell (SC)–inductively coupled plasma–mass spectrometry (ICP-MS) provide a novel means to quantify pre-formed core–shell metal–plastic composite nanoparticles when exposed to two freshwater algal cells, Cryptomonas ovata (C. ovata) and Cryptomonas ozolini (C. ozolini). It is shown that individual palladium plastic nanoparticles (Pd NPPs) exposed to algal cells form agglomerates in the cell suspension respectively consisting of 165 and 157 (±3.8) individual Pd NPPs for C. ozolini and C. ovata cells, and that the agglomerates are also cell-associated with 1.75–1.85 agglomerates per cell.
Keywords: algae, algal exposure to nanoplastics, core shell nanoparticles, environmental nanoscience, freshwater algae, nanoparticles, nanoplastics, plastic nanoparticles, single cell, single particle, SC-ICP-MS, SP-ICP-MS.
The introduction of synthetic polymers has profoundly affected human society. Their chemical and physical properties make them ideally suited for many different applications including, but not limited to, medical supplies, food packaging, clothing and construction materials (Worm et al. 2017; Huang et al. 2021). Plastics are made in different sizes and eventually will breakdown and enter the microscale (1 μm–5 mm, in principle, but studies tend to examine the larger fractions) and nanoscale 1–100 or 1–1000 nm (Gigault et al. 2018; Gonçalves and Bebianno 2021). These small plastic materials enter the environment; it has been reported that wastewater treatment plants are one of the main pathways of microplastic pollution, and treated wastewater is an important source of nanoplastic discharge to freshwater sources (Carr et al. 2016; Murray and Örmeci 2020). Once in freshwater, microplastics are persistent and can have negative effects on the ecosystem (Wang et al. 2022). It has been shown that microplastics disrupt photosynthetic activity of freshwater algae due to the accumulation of intracellular reactive oxygen species (ROS) and the interruption of photosynthetic electron transport (Wu et al. 2019; Wang et al. 2022).
Once in the environment, plastics continue to break down into smaller nano-sizes (Gigault et al. 2018; Gonçalves and Bebianno 2021). Nanoplastics pose a risk to human health and the environment because they are persistent, easily ingested by microorganisms, can move to higher trophic levels and act as carriers for harmful chemicals (Hartmann et al. 2017; Cassano et al. 2023). It has been reported that nanoplastics can cross biological barriers (Mattsson et al. 2016), penetrate tissues (Kashiwada 2006), accumulate in organs (von Moos et al. 2012), disrupt metabolism (Mattsson et al. 2017; Ferreira et al. 2019) and adversely affect human health (Chang et al. 2020; Yee et al. 2021; Danopoulos et al. 2022). Nanoplastics might either have direct biological impacts or be a vector for the delivery of other materials such as plasticisers or metals (Thiagarajan et al. 2021; Cao et al. 2022).
Detection and quantification of smaller plastic particles have been challenging due to their chemical composition and size (Hendriks et al. 2023). Current techniques employed include hyperspectral imaging (Mattsson et al. 2017) and scanning electron microscopy (SEM) combined with Raman microscopy (Schmidt et al. 2021). Current methods have advantages but also significant limitations. For instance, Raman microscopy can identify different polymer types and is capable of resolutions down to 0.5 μm (Schmidt et al. 2021). Surface-enhanced Raman spectroscopy (SERS) has been used to measure 5 mg L−1 of 50-nm polystyrene nanoplastics in river water. These are much higher concentrations than likely in most environments and the method showed limitations due to poor reproducibility and matrix effects (Zhou et al. 2021). More recently, Hendriks et al. 2023 developed a method using single cell inductively coupled plasma time-of-flight mass spectrometry to quantify 200-nm nanoplastic–cell associations with human cells (Hendriks et al. 2023).
In this work, we report the use of single cell (SC)– and single particle (SP)–inductively coupled plasma–mass spectrometry (ICP-MS) to quantify synthetic nanoparticles consisting of a palladium and polyacrylonitrile (PAN) core with an outer polystyrene shell (Mitrano et al. 2019). This powerful combination of methods allows quantification of single particles in solution and particle–cell interactions on a single cell basis.
Palladium (Pd) plastic nanoparticles (Pd NPPs) were synthesised using a previously published method (Mitrano et al. 2019). The particles consist of a PAN core material that contains Pd as a metal tracer with a polystyrene outer shell. The core consisted of palladium surrounded by PAN which complexes Pd in the water phase (Mitrano et al. 2019). Details on the synthesis and characterisation of the particles can be found in Supplementary Table S1.
Two freshwater algal cell cultures were used in the exposure experiments, Cryptomonas ovata (C. ovata) and Cryptomonas ozolini (C. ozolini). The cells originated from The Culture Collection of Algae at the University of Texas at Austin (UTEX, strain numbers: C. ovata UTEX 2783, C. ozolini UTEX 2194). Cryptomonas ovata and C. ozolini cell cultures were grown in a ThermoFisher incubator at 20°C and with a 12-h light:12-h dark cycle until cell concentrations reached ~400,000 cells mL−1 (Merrifield et al. 2018). Cell suspension preparation and introduction into the plasma were optimised prior to exposing cells to particles. Once cell suspensions were ready, they were divided into 10-mL aliquots and exposed to 0.5 μg L−1 Pd NPPs for 72 h. A description of the cell suspension exposure and preparation can be found in the Supplementary material.
Supplementary Fig. S1 shows a simplified schematic of the cell preparation steps prior to SC-ICP-MS analysis. After preparation of the cell suspensions and prior to analysis by SC-ICP-MS, 2.2-mL aliquots of the cell suspensions were collected and preserved with Lugol’s iodine solution (VWR) to a final concentration of 2% v/v to count the cells. The cell aliquots were stored at 4°C until ready to count. Immediately following SC-ICP-MS analysis of the cells, the supernatant samples collected during the wash steps were diluted 1/10 (ultrapure water, 18.2 MΩ cm, Purelab Option-Q) and analysed by SP-ICP-MS. Cells were later counted using a Nikon Eclipse TS 100 inverted microscope at 40× power according to the Utermöhl method (Utermöhl 1931).
SP-ICP-MS (Pace et al. 2011; Reed et al. 2012; Bi et al. 2014; Tharaud et al. 2017; Lead et al. 2018) was used to quantify particle number concentration and other properties on a single particle basis in the suspension phase. Here, SP-ICP-MS was used to detect Pd within the PAN core in the collected supernatant by their size in nanometres (nm). It should be stated that the theoretical size detection limit for Pd nanoparticles by ICP-MS is 25–30 nm (Lee et al. 2014; Kińska et al. 2018). Here, we report a size detection limit for Pd NPPs of 31 (±4) and 32 (±1) nm for C. ozolini and C. ovata respectively. This equates to a mass detection limit of ~293 and 226 attograms (ag) for C. ozolini and C. ovata respectively, using the upper size limit within the standard deviation.
Supplementary Fig. S2–S4 respectively show the size distribution of the prepared Pd NPPs using dynamic light scattering (DLS), transmission electron microscopy (TEM) images of the NPPs and TEM–energy dispersive X-ray analysis (EDX) images of the NPPs. The size of the Pd NPPs based on TEM was 42 nm with a 10-nm Pd and PAN core as the tracer.
SP-ICP-MS parameters were modified from those we have used previously (Merrifield et al. 2018). The sample introduction system consisted of a PerkinElmer Asperon spray chamber, a high-efficiency nebuliser (HEN, Meinhard) and a 2-mm quartz injector (Elemental Scientific). Prior to analysis, the ICP-MS was tuned with a 1-ppb multi-element PerkinElmer SmartTune solution. The transport efficiency (TE) was determined using a 60-nm citrate-coated gold nanoparticle (nanoComposix, AUCN60) standard diluted to a concentration of 200,000 particles mL−1. The analytical run conditions including the nebuliser gas flow, additional gas flow, sample flow rate, transport efficiency and tune parameters can be found in Supplementary Table S5.
In our work, the TE for SC-ICP-MS (11.2–19.2%) is consistently higher than for SP-ICP-MS (6.4–8.3%). This may be due to the different geometries of the spray chambers, different gas flows and different sample flow rates. The SP pray chamber is a standard cyclonic spray chamber with a turbulent flow, whereas the SC spray chamber is an elongated Asperon style that is far less turbulent. In addition, sample flow rates are much lower using the Asperon spray chamber than the cyclonic spray chamber, ~0.07 and ~0.3 mL min−1 respectively (Supplementary Tables S2 and S5).
Table 1 and Supplementary Table S3 show the SP-ICP-MS data for the suspension phase samples collected from the wash steps. The SP data correspond to the Pd core tracer and not the outer polymer shell; TEM images show the NPP core Pd size to be ~10 nm and the whole NPP to be 42 nm. Using eqn 1 in the Supplementary material, the calculated mass of a 10-nm Pd particle, assuming the particle is spherical, is 6.28 ag (ρ = 12.0 g cm−3). SP-ICP-MS data respectively yielded a mean size for Pd of 55 and 54 nm for particles in the C. ozolini and C. ovata aqueous suspensions (not shown in Table 1). The mean mass for each agglomerate in the suspension phase was calculated from the mean size to be 1029 (±24) and 975 (±23) ag of Pd for the C. ozolini and C. ovata cell suspensions respectively. Based on this difference, the Pd NPPs formed agglomerates in the suspension phase, although we do not have data about the presence of dispersed NPPs, so can not rule out their presence. The mean number of NPPs in each agglomerate in suspension was equal to 165 and 157 (±3.8) individual Pd NPPs for the C. ozolini and C. ovata suspensions respectively.
SP-ICP-MS (suspension phase) | ||||
---|---|---|---|---|
Cell type media | Mean mass of Pd per agglomerate in suspension (ag) (±s.d.) | Number of Pd NPPs per agglomerate in suspension (mean mass/6.28 ag) (±s.d.) | Mean diameter per Pd NPP agglomerate (nm) (±s.d.) | |
Cryptomonas ozolini exposure media | 1029 (24) | 165 (3.8) | 55 (0.4) | |
Cryptomonas ovata exposure media | 975 (23) | 157 (3.8) | 54 (0.4) |
The SC-ICP-MS data in Table 2 yielded a mean mass of Pd per cell for C. ovata and C. ozolini of 1802 (±222) and 1785 (±176) ag respectively. Given the mean mass of individual NPPs and agglomerates, we calculate a mean number of NPPs of 286 (±28) and 284 (±36) per C. ovata and C. ozolini cell respectively. This equates to a mean of 1.75–1.85 agglomerates per cell, where they are present (see also Supplementary Table S4). The agglomerates of Pd NPPs were cell associated, either bound to or uptaken by the cell.
SC-ICP-MS (cell phase) | |||||
---|---|---|---|---|---|
Cell type | Mean mass of Pd per cell (ag) (±s.d.) | Number of Pd NPPs per cell (mean mass/6.28 ag) (±s.d.) | Number of peaks (cells) (±s.d.) | Pd NPP agglomerates per cell (mean mass of Pd per cell from SC data/mean mass of Pd per agglomerate from SP data) (±s.d.) | |
Cryptomonas ozolini | 1785 (176) | 284 (28) | 422 (102) | 1.7 (0.2) | |
Cryptomonas ovata | 1802 (222) | 286 (36) | 344 (99) | 1.8 (0.2) |
Fig. 1 shows a simplified schematic of the Pd NPP interaction based on SP- and SC-ICP-MS data. The combined data from both methods provide detailed information on the agglomeration of metal-labelled nanoplastic particles in suspension and their cell association when exposed to two different freshwater algal cells. As shown in Fig. 1, agglomeration of Pd NPPs increases in the presence of cells. This is consistent with others who found that changes in surface charge of micro- and nanoplastics enhances their ability to aggregate with algae (Wang et al. 2023; Zhao et al. 2023). It has also been reported that algae excrete polysaccharides, either at high cell concentrations or when they are stressed, increasing agglomeration (Long et al. 2015). Without further investigation, it is assumed that most of these agglomerates are external to the cell (as shown in Fig. 1), because of their size. However, it is possible the dispersed NPPs can be taken up, or that deagglomeration can occur at the cell wall.
Simplified schematic of the Pd@polymer nanoplastic nanoparticles and the difference between the solution phase and cell samples after the 72-h exposure. As shown in the schematic, both the suspension phase and cell samples showed agglomeration of Pd NPPs.
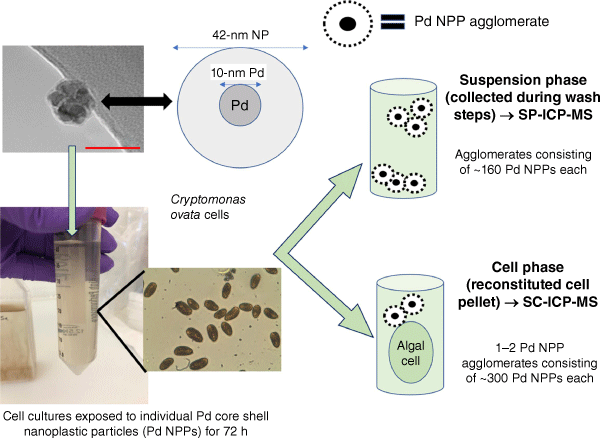
Taken together, the use of labelled nanoplastics and SC- and SP-ICP-MS provide a powerful means to examine solution phase transformations and cell association. Given the limitations of analytical sensitivity, there is no information about individual NPPs. This limitation strongly suggests the need for additional techniques within a multi-method approach (Domingos et al. 2009; Baalousha and Lead 2012; Baalousha et al. 2012a, 2012b), including TEM and confocal microscopy, in order to fully understand NPP behaviour.
Data availability
The data that support this study will be shared upon reasonable request to the corresponding author.
Conflicts of interest
Jamie Lead is the Editor-in-Chief of Environmental Chemistry, but did not at any stage have editor-level access to this manuscript while in peer review, as is the standard practice when handling manuscripts submitted by an editor to this journal. Environmental Chemistry encourages its editors to publish in the journal and they are kept totally separate from the decision-making processes for their manuscripts. The authors have no further conflicts of interest to declare.
Declaration of funding
This work was financially supported by the University of South Carolina SmartState Center for Environmental Nanoscience and Risk (CENR) and by the National Institute of Environmental Health Sciences of the National Institutes of Health under Award Number P01ES028942.
Acknowledgements
Elizabeth Bair thanks Kristiaän Merritt from the University of South Carolina Phytoplankton Ecology Research Laboratory for providing cultures of Cryptomonas ovata and Cryptomonas ozolini and cell growth media. The anonymous journal reviewers are thanked for their review of this manuscript.
References
Baalousha M, Lead JR (2012) Rationalizing nanomaterial sizes measured by atomic force microscopy, flow field-flow fractionation, and dynamic light scattering: sample preparation, polydispersity, and particle structure. Environmental Science & Technology 46, 6134-6142.
| Crossref | Google Scholar | PubMed |
Baalousha M, Ju-Nam Y, Cole PA, Gaiser B, Fernandes TF, Hriljac JA, Jepson MA, Stone V, Tyler CR, Lead JR (2012a) Characterization of cerium oxide nanoparticles – part 1: size measurements. Environmental Toxicology and Chemistry 31, 983-993.
| Crossref | Google Scholar |
Baalousha M, Ju-nam Y, Cole PA, Hriljac JA, Jones IP, Tyler CR, Stone V, Fernandes TF, Jepson MA, Lead JR (2012b) Characterization of cerium oxide nanoparticles – part 2: nonsize measurements. Environmental Toxicology and Chemistry 31, 994-1003.
| Crossref | Google Scholar |
Bi X, Lee S, Ranville JF, Sattigeri P, Spanias A, Herckes P, Westerhoff P (2014) Quantitative resolution of nanoparticle sizes using single particle inductively coupled plasma mass spectrometry with the K-means clustering algorithm. Journal of Analytical Atomic Spectrometry 29, 1630-1639.
| Crossref | Google Scholar |
Cao J, Liao Y, Yang W, Jiang X, Li M (2022) Enhanced microalgal toxicity due to polystyrene nanoplastics and cadmium co-exposure: from the perspective of physiological and metabolomic profiles. Journal of Hazardous Materials 427, 127937.
| Crossref | Google Scholar | PubMed |
Carr SA, Liu J, Tesoro AG (2016) Transport and fate of microplastic particles in wastewater treatment plants. Water Resources 91, 174-182.
| Crossref | Google Scholar | PubMed |
Cassano D, Bogni A, La Spina R, Gilliland D, Ponti J (2023) Investigating the cellular uptake of model nanoplastics by single-cell ICP-MS. Nanomaterials 13, 594.
| Crossref | Google Scholar | PubMed |
Chang X, Xue Y, Li J, Zou L, Tang M (2020) Potential health impact of environmental micro‐ and nanoplastics pollution. Journal of Applied Toxicology 40, 4-15.
| Crossref | Google Scholar | PubMed |
Danopoulos E, Twiddy M, West R, Rotchell JM (2022) A rapid review and meta-regression analyses of the toxicological impacts of microplastic exposure in human cells. Journal of Hazardous Materials 427, 127861.
| Crossref | Google Scholar | PubMed |
Domingos RF, Baalousha MA, Ju-Nam Y, Reid MM, Tufenkji N, Lead JR, Leppard GG, Wilkinson KJ (2009) Characterizing manufactured nanoparticles in the environment: multimethod determination of particle sizes. Environmental Science & Technology 43, 7277-7284.
| Crossref | Google Scholar | PubMed |
Ferreira I, Venâncio C, Lopes I, Oliveira M (2019) Nanoplastics and marine organisms: what has been studied? Environmental Toxicology and Pharmacology 67, 1-7.
| Crossref | Google Scholar | PubMed |
Gigault J, Halle AT, Baudrimont M, Pascal P-Y, Gauffre F, Phi T-L, El Hadri H, Grassl B, Reynaud S (2018) Current opinion: what is a nanoplastic? Environmental Pollution 235, 1030-1034.
| Crossref | Google Scholar | PubMed |
Gonçalves JM, Bebianno MJ (2021) Nanoplastics impact on marine biota: a review. Environmental Pollution 273, 116426.
| Crossref | Google Scholar | PubMed |
Hartmann NB, Rist S, Bodin J, Jensen LH, Schmidt SN, Mayer P, Meibom A, Baun A (2017) Microplastics as vectors for environmental contaminants: exploring sorption, desorption, and transfer to biota. Integrated Environmental Assessment and Management 13, 488-493.
| Crossref | Google Scholar | PubMed |
Hendriks L, Kissling VM, Buerki-Thurnherr T, Mitrano DM (2023) Development of single-cell ICP-TOFMS to measure nanoplastics association with human cells. Environmental Science: Nano 10, 3439-3449.
| Crossref | Google Scholar | PubMed |
Huang D, Tao J, Cheng M, Deng R, Chen S, Yin L, Li R (2021) Microplastics and nanoplastics in the environment: macroscopic transport and effects on creatures. Journal of Hazardous Materials 407, 124399.
| Crossref | Google Scholar | PubMed |
Kashiwada S (2006) Distribution of nanoparticles in the see-through medaka (Oryzias latipes). Environmental Health Perspectives 114, 1697-1702.
| Crossref | Google Scholar | PubMed |
Kińska K, Jiménez-Lamana J, kowalska J, Krasnodębska-Ostręga B, Szpunar J (2018) Study of the uptake and bioaccumulation of palladium nanoparticles by Sinapis alba using single particle ICP-MS. Science of the Total Environment 615, 1078-1085.
| Crossref | Google Scholar | PubMed |
Lead JR, Batley GE, Alvarez PJJ, Croteau MN, Handy RD, Mclaughlin MJ, Judy JD, Schirmer K (2018) Nanomaterials in the environment: behavior, fate, bioavailability, and effects – an updated review. Environmental Toxicology and Chemistry 37, 2029-2063.
| Crossref | Google Scholar | PubMed |
Lee S, Bi X, Reed RB, Ranville JF, Herckes P, Westerhoff P (2014) Nanoparticle size detection limits by single particle ICP-MS for 40 elements. Environmental Science & Technology 48, 10291-10300.
| Crossref | Google Scholar | PubMed |
Long M, Moriceau B, Gallinari M, Lambert C, Huvet A, Raffray J, Soudant P (2015) Interactions between microplastics and phytoplankton aggregates: impact on their respective fates. Marine Chemistry 175, 39-46.
| Crossref | Google Scholar |
Mattsson K, Adolfsson K, Ekvall MT, Borgström MT, Linse S, Hansson L-A, Cedervall T, Prinz CN (2016) Translocation of 40 nm diameter nanowires through the intestinal epithelium of Daphnia magna. Nanotoxicology 10, 1160-1167.
| Crossref | Google Scholar | PubMed |
Mattsson K, Johnson EV, Malmendal A, Linse S, Hansson L-A, Cedervall T (2017) Brain damage and behavioural disorders in fish induced by plastic nanoparticles delivered through the food chain. Scientific Reports 7, 11452.
| Crossref | Google Scholar | PubMed |
Merrifield RC, Stephan C, Lead JR (2018) Quantification of Au nanoparticle biouptake and distribution to freshwater algae using single cell-ICP-MS. Environmental Science & Technology 52, 2271-2277.
| Crossref | Google Scholar | PubMed |
Mitrano DM, Beltzung A, Frehland S, Schmiedgruber M, Cingolani A, Schmidt F (2019) Synthesis of metal-doped nanoplastics and their utility to investigate fate and behaviour in complex environmental systems. Nature Nanotechnology 14, 362-368.
| Crossref | Google Scholar | PubMed |
Murray A, Örmeci B (2020) Removal effectiveness of nanoplastics (<400 nm) with separation processes used for water and wastewater treatment. Water 12, 635.
| Crossref | Google Scholar |
Pace HE, Rogers NJ, Jarolimek C, Coleman VA, Higgins CP, Ranville JF (2011) Determining transport efficiency for the purpose of counting and sizing nanoparticles via single particle inductively coupled plasma mass spectrometry. Analytical Chemistry 83, 9361-9369.
| Crossref | Google Scholar | PubMed |
Reed RB, Higgins CP, Westerhoff P, Tadjiki S, Ranville JF (2012) Overcoming challenges in analysis of polydisperse metal-containing nanoparticles by single particle inductively coupled plasma mass spectrometry. Journal of Analytical Atomic Spectrometry 27, 1093-1100.
| Crossref | Google Scholar |
Schmidt R, Nachtnebel M, Dienstleder M, Mertschnigg S, Schroettner H, Zankel A, Poteser M, Hutter H-P, Eppel W, Fitzek H (2021) Correlative SEM-Raman microscopy to reveal nanoplastics in complex environments. Micron 144, 103034.
| Crossref | Google Scholar | PubMed |
Tharaud M, Gondikas AP, Benedetti MF, von der Kammer F, Hofmann T, Cornelis G (2017) TiO2 nanomaterial detection in calcium rich matrices by spICPMS. A matter of resolution and treatment. Journal of Analytical Atomic Spectrometry 32, 1400-1411.
| Crossref | Google Scholar |
Thiagarajan V, Alex SA, Seenivasan R, Chandrasekaran N, Mukherjee A (2021) Interactive effects of micro/nanoplastics and nanomaterials/pharmaceuticals: their ecotoxicological consequences in the aquatic systems. Aquatic Toxicology 232, 105747.
| Crossref | Google Scholar | PubMed |
Utermöhl H (1931) Neue Wege in der quantitativen Erfassung des Plankton. (Mit besonderer Berücksichtigung des Ultraplanktons.). SIL Proceedings, 1922-2010 5, 567-596 [In German].
| Crossref | Google Scholar |
von Moos N, Burkhardt-Holm P, Köhler A (2012) Uptake and effects of microplastics on cells and tissue of the blue mussel Mytilus edulis L. after an experimental exposure. Environmental Science & Technology 46, 11327-11335.
| Crossref | Google Scholar | PubMed |
Wang Q, Wang J, Chen H, Zhang Y (2022) Toxicity effects of microplastics and nanoplastics with cadmium on the alga Microcystis aeruginosa. Environmental Science and Pollution Research 30, 17360-17373.
| Crossref | Google Scholar | PubMed |
Wang Q, Liu WT, Zeb A, Lian YH, Shi RY, Li JT, Zheng ZQ (2023) Toxicity effects of polystyrene nanoplastics and arsenite on Microcystis aeruginosa. Science of the Total Environment 874, 162496.
| Crossref | Google Scholar |
Worm B, Lotze HK, Jubinville I, Wilcox C, Jambeck J (2017) Plastic as a persistent marine pollutant. Annual Review of Environment and Resources 42, 1-26.
| Crossref | Google Scholar |
Wu Y, Guo P, Zhang X, Zhang Y, Xie S, Deng J (2019) Effect of microplastics exposure on the photosynthesis system of freshwater algae. Journal of Hazardous Materials 374, 219-227.
| Crossref | Google Scholar | PubMed |
Yee MS-L, Hii L-W, Looi CK, Lim W-M, Wong S-F, Kok Y-Y, Tan B-K, Wong C-Y, Leong C-O (2021) Impact of microplastics and nanoplastics on human health. Nanomaterials 11, 496.
| Crossref | Google Scholar | PubMed |
Zhao Y, Tao S, Liu S, Hu T, Zheng K, Shen M, Meng G (2023) Research advances on impacts micro/nanoplastics and their carried pollutants on algae in aquatic ecosystems: a review. Aquatic Toxicology 264, 106725.
| Crossref | Google Scholar | PubMed |
Zhou XX, Liu R, Hao LT, Liu JF (2021) Identification of polystyrene nanoplastics using surface enhanced Raman spectroscopy. Talanta 221, 121552.
| Crossref | Google Scholar | PubMed |