Seventy-five years of vegetation change after fire in Tasmanian alpine heathland
Ellen-Rose Sorensen A and Jamie B. Kirkpatrick
A
Abstract
Alpine ecosystems are threatened by warming and an associated increase in fire frequency. There is a gap in our knowledge of succession in Tasmanian alpine heath more than 50 years after fire. The literature suggests that the alpine successional progression usually involves decreasing rates of change, decreasing differences among fire ages, ongoing transitions among shrub species, ongoing transitions from some lifeforms/species to others, and that warming results in increases in species richness.
We test for these tendencies up to 75 years from fire in alpine vegetation on kunanyi/Mount Wellington, Tasmania, Australia.
We documented the changes in vegetation structure and composition between 1998 and 2022 in plots on either side of an alpine fire boundary in the alpine heathland and used earlier data and observations to extend the record of change after fire to 75 years. We put these changes in the context of the only area of alpine vegetation that was not burnt in 1947 or later.
The area last burnt in 1947 exhibited declines in all lifeform covers between 1998 and 2022. All lifeforms except tall shrubs and mat shrubs declined in cover in the area last burnt in 1962. By 2022, shrub cover in the 1962-burnt area had not attained equivalence with the area last burnt in 1947. Herbs had the most dramatic decline in both fire-age classes. There were few shrub seedlings in 2022. All but six taxa, three being exotic, were observed in both the plots and previous broader surveys. Increases in species richness caused by the upward migration of lower-elevation species were not observed. The long-unburnt patch lacked the major dominant of the 1947-burnt plots, namely Orites acicularis, and was dominated by a gymnosperm absent from most of the mountain.
Succession follows the initial floristic composition model. The differences in trajectories from the 1947 and 1962 fires could possibly be due to desiccation or abrasion damage from increasing wind speeds and temperatures. There are strong indications of further potential change in the absence of fire.
The slow rate of recovery and its on-going nature emphasise the importance of keeping fire out of this vegetation type.
Keywords: alpine heathland, climate change, fire succession, floristics, long-term dynamics, slope effects, vegetation structure.
Introduction
Understanding successional processes that lead to the attainment of relative stability in vegetation composition and structure has been a major focus in ecology (Connell and Slatyer 1977; White 1979; Niering 1987). Successional theory, as originally conceived, suggests that, following exogenous disturbance such as fire, plant communities tend to return to a stable composition, in which establishment is triggered by endogenous processes, such as the senescence of mature individuals (Connell and Slatyer 1977). Vegetation dynamics following fire are now understood to not necessarily lead to the pre-disturbance state or even a stable state (Pickett et al. 2009). Alternative stable states can result from different exogenous disturbance regimes (Jackson 1968; Laycock 1991; Lasslop et al. 2016) and from different conditions in the early re-establishment phase (Egler 1954). The notion of stability within a ‘stable’ state may be illusory, with negative feedback systems setting up fluctuations in structure and species composition, as in many cyclic processes, such as the ridge–swale temporal replacement patterns in some Tasmanian subalpine bogs (Morgan et al. 2010). Nevertheless, an understanding of the directionality and speed of vegetation change after exogenous disturbance is important in understanding the implications for nature conservation of changes in disturbance regimes.
Even in fire-sensitive areas, such as alpine ecosystems, fire has an important role in the maintenance of biodiversity (Williams et al. 2008, 2012). The frequency and intensity of bushfires in Australian alpine ecosystems are projected to increase with climate change (Camac et al. 2017). Vegetation recovery from fire in alpine ecosystems can take years to centuries (Kirkpatrick and Dickinson 1984; Misiak 2007; Kirkpatrick and Bridle 2013). Whereas alpine grasslands and herbfields can recover their pre-fire vegetation structure and composition within a decade or two after fire (Verrall and Pickering 2019), other vegetation types such as coniferous heath and alpine bogs can take centuries (Cheal 2010; Kirkpatrick et al. 2010; Tolsma 2020). After the British invasion, fire was used as a tool in alpine vegetation in the Central Plateau of Tasmania to eliminate unpalatable shrubs and encourage the growth of palatable herbs and grasses (Kirkpatrick and Bridle 2013). The results of an extensive series of human-ignited fires in the late 1960s in alpine Tasmania (Kirkpatrick and Dickinson 1984) resulted in a policy of fire exclusion (Kirkpatrick and Bridle 2013), although lightning fires have occurred more frequently since the 1990s (Kirkpatrick et al. 2018).
Ecological monitoring over decades can contextualise what could otherwise be perceived as short-term dynamics, particularly in relation to climate change (Verrall et al. 2021), and provide information about successional processes after disturbance in slow-growing ecosystems, such as the alpine, that may otherwise not be gained (Bakker et al. 1996; Scherrer and Pickering 2005).
Alpine heathlands are dynamic communities even many decades after fire when structural and compositional change can still occur (Churchill and Hanson 1958; Carr 1962; Williams and Ashton 1988; Williams 1992). The early successional stages following fire in Australian alpine heathlands are characterised by a high proportion of bare ground, with the dominant lifeforms being grasses, sedges, rushes and herbs (Carr 1962; Wahren et al. 2001; Cheal 2010). This structure was seen in the kunanyi 1962 burn area in 1978 (Kirkpatrick et al. 2002a). The mid-stage of succession is characterised by an expansion of shrubs and a decline in bare ground, herb cover and grass cover (Cheal 2010), which exemplifies the structure of the 1962-burnt area in 1998 (Kirkpatrick et al. 2002a). The late stage is characterised by the dominance of tall shrubs, with there being minimal available bare ground for any other lifeforms to establish. Shrub regeneration may be largely dependent on the creation of gaps caused by the senescence of older shrubs, sometimes within the canopy gap of the shrub (Williams and Ashton 1988; Williams 1992).
Only a few Australian studies have documented the long-term recovery from fire by itself, with most of the work covering recovery after the cessation of both fire and grazing. In Tasmanian alpine vegetation, Kirkpatrick and Dickinson (1984) found that between 11 and 40 years after fire in areas never grazed by stock, there was limited regeneration of shrubs. Following on from the 1984 study, Kirkpatrick et al. (2010) found that the long-term effects of fire on vegetation were severe and persistent, with a delayed reinvasion of dominant shrub species (both gymnosperms and angiosperms) and much bare ground still apparent 43–69 years after fire. These two studies largely took place in coniferous heaths, which are dominated by slow-growing shrub species from the Gymnospermae that are commonly dominant in the western and central mountains; however, most of the Tasmanian and mainland Australian alpine heath is dominated by shrub species in the Angiospermae (Kirkpatrick 1997). Harrison-Day et al. (2016) documented Orites acicularis and Eucalyptus coccifera expansion in angiosperm-dominated alpine vegetation between three and five decades after fire on Mount Rufus in central Tasmania. Kirkpatrick et al. (2002a) examined the long-term impacts of fire in angiosperm alpine heath on kunanyi in the south-east of Tasmania, re-sampling plots that were first placed 31 and 16 years after fires burnt parts of the study area in 1947 and 1962. Fifty-one years was insufficient time since fire for the alpine heath community to reach a stable state in either vegetation structure or species composition. In this and other longitudinal studies of succession in alpine vegetation after fire, there is a slowing of the rate of change, a convergence in the characteristics of burnt and unburnt and temporal transitions in dominance and presence of taxa associated with the colonisation of bare ground and increasing dominance of taller taxa.
Climate change is predicted to have a severe impact on alpine ecosystems (Verrall and Pickering 2020). Increasing temperatures and decreases in snow persistence and snow depth have been recorded on the Australian mainland (Nicholls 2005; Green and Pickering 2009; McGowan et al. 2018; Ji et al. 2022). There has been a decrease in snow incidence below 1350 m a.s.l. in Tasmania since the mid- to late-20th century (Kirkpatrick et al. 2017). A decline in snow persistence threatens plant species confined to snow patches (Green and Pickering 2009). The migration of thermophilic species from lower elevations into the alpine zone has been recorded in the mainland Australian alpine zone (Verrall et al. 2021; Auld et al. 2022) and in alpine areas worldwide (Walther et al. 2005; Jurasinski and Kreyling 2007; Lenoir et al. 2008; He et al. 2019). However, Cannone and Pignatti (2014) noted that ‘range-filling’, the dispersal of species from neighbouring sites at similar elevation, is also occurring in the alpine zone, which is not necessarily related to warming temperatures.
An expansion of shrubs in the alpine zone through the densification of individuals and the establishment of shrub seedlings in areas previously not dominated by shrubs has occurred as temperatures have increased in Australia (McDougall 2003; Scherrer and Pickering 2005; Venn et al. 2012, 2014; Verrall et al. 2021) as well as in many other alpine areas around the world (Myers-Smith et al. 2011). Warming has been found to be associated with a statistically significant increase in shrub growth in Australian alpine heath (Wahren et al. 2013). Given the above findings, we expected that the 20-year odd difference in fire age on kunanyi could have resulted in different trajectories of change, and that species richness might increase.
We present data on 75 years of alpine vegetation change after fire on an Australian mountain that has experienced over 1°C increase in mean annual temperature over the same period. We test the hypotheses that there have been decreasing rates of change; decreasing differences between fire ages; ongoing transitions among shrub species; ongoing transitions from some lifeforms/species to others, associated with the successional process, as bare ground is colonised and taller plants establish. We put these changes in the context of the only small area of alpine vegetation on kunanyi that was not been burnt in 1947 or later. We also attempt to deduce whether any of the results of our analyses could relate to climate change rather than successional processes. We tested for the increases in species richness found elsewhere.
Materials and methods
Study area
The study area is located on the alpine plateau of kunanyi (Mount Wellington), Tasmania, Australia, which has a maximum elevation of 1270 m above sea level (Fig. 1). The geology of the alpine zone of kunanyi is predominantly Jurassic dolerite (Kirkpatrick 1996). The landscape of the plateau is dominated by dolerite boulders and alpine heathland, with some areas lower on the slope covered by alpine bogs and Poa tussock grasslands (Martin 1940; Kirkpatrick 1996). O. acicularis (authorities are given in the Supplementary Appendix) is the most dominant of the shrubs in the alpine heath of the plateau (Kirkpatrick et al. 2002a). The mountain was subject to periglacial activity during the Last Glacial (Kirkpatrick 1996). The soils on the plateau are shallow, consisting of organosols and rudosols. Charcoal deposits in an alpine bog on kunanyi indicate that fire occurred occasionally prior to the British invasion of Tasmania in the early 19th century, becoming more frequent post-settlement (Whinam and Kirkpatrick 1994). Fires have burnt on parts of the alpine plateau in 1914, 1947, 1962 and 1967 (Wellington Park Management Trust 2006).
Location of plots in the study area by date of last fire. Twenty metre contours are shown, as is the elevation of the peak. The geolocation of the peak is 42°52′S, 147°14′E. Figure has been modified from fig. 1 in Kirkpatrick et al. (2002a).
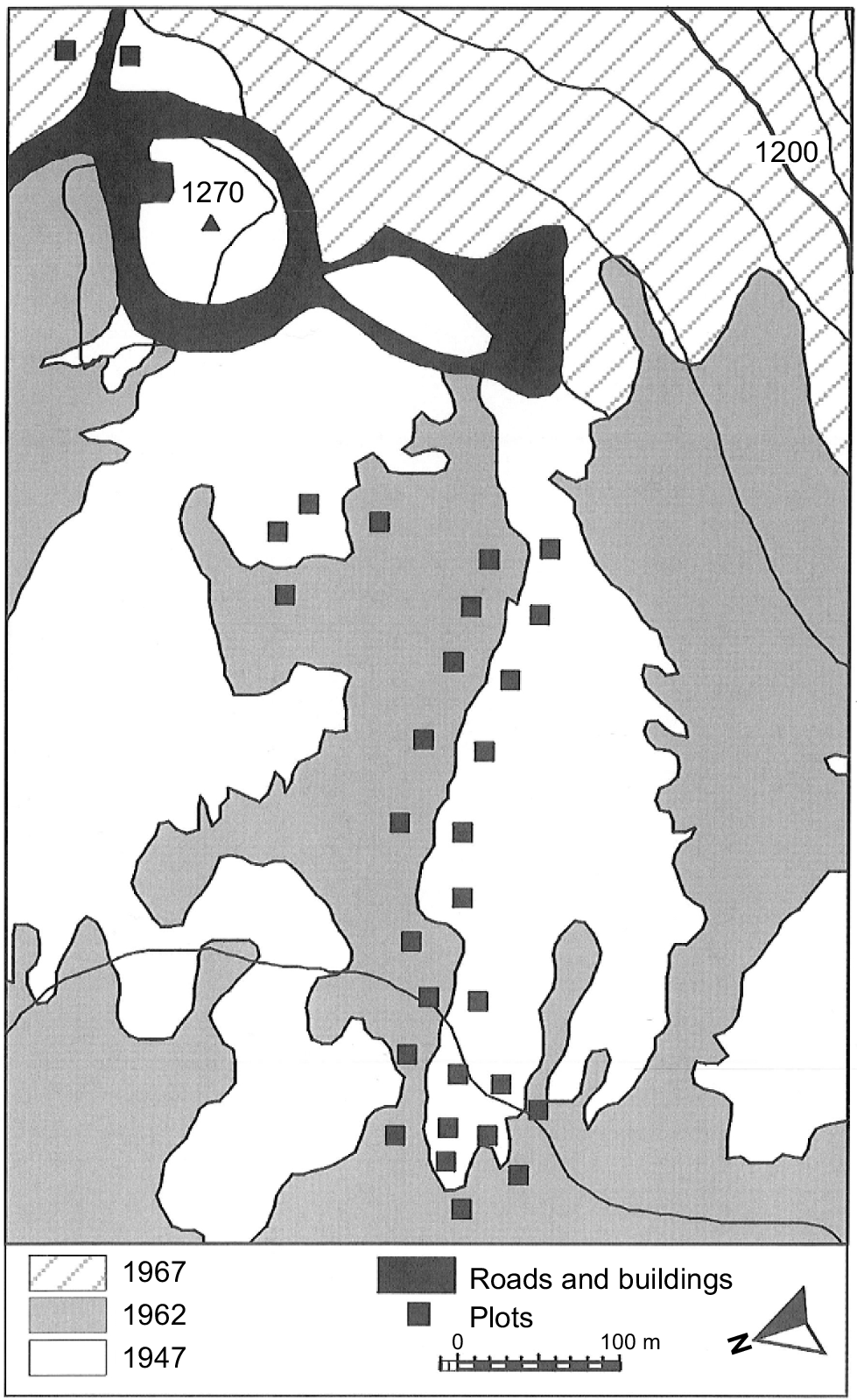
Mean rainfall in the driest month (September) at the kunanyi Pinnacle Station averages 72.4 mm (113.4 mm at the Springs weather station, located at 720 m a.s.l.), and in the wettest month (December) it averages 87.9 mm (118.1 mm at the Springs). Rainfall on the Pinnacle is approximately 27% lower annually than that at the Springs, 36% lower in the driest month and 25% lower in the wettest (Bureau of Meteorology 2022). Unlike some other alpine habitats in Tasmania and most of the alpine zone on mainland Australia, snow does not persist long during the winter months except for one small snow patch (Parry et al. 2016). Mean daily maximum temperature in the coldest month (July) is 2.5°C and in the warmest month (January) it is 13.6°C.
There has been an increase in the mean annual daily maximum temperature (from 7.2°C to 8.4°C) and mean annual daily minimum temperature (from 0.9°C to 1.7°C) on kunanyi between 1961 and 2020, as derived from a line of best fit to the data from the BOM station on the top of the mountain. The rainfall data from the kunanyi summit are unreliable because of the interaction of strong winds and snow and hail. Annual rainfall at the BOM station at the Springs, on the eastern slopes below the peak, showed no clear tendency to increase or decrease. The three lowest-rainfall years were 1963 (810.6 mm), 1968 (791.5 mm) and 1979 (813.6 mm), and the highest-rainfall years were in 1975 (1607.8 mm), 1996 (1653 mm), 2009 (1862.1 mm) and 2020 (1347 mm). There has been an increase in the mean annual wind speed at both the kunanyi station and the Hobart Airport West station between 1961 and 2022, with mean speed increasing from 25 km/h to 32 km/h at the kunanyi Pinnacle and from 14 km/h to 17 km/h at Hobart Airport West in lines of best fit.
Data collection
Fieldwork occurred in March 2022. Site location and methods for data collection followed Kirkpatrick et al. (2002a). It was not possible to exactly replicate the site locations from the 1998 survey; however, they were approximated using photographs taken for the earlier study. Thirty 5 m × 5 m plots were placed on either side of the boundary line separating vegetation that last burnt in 1962, and vegetation last burnt in 1947, with 15 plots in each area (Fig. 1). However, the eastern-most site was last burnt in 1967; following Kirkpatrick et al. (2002a), this site is treated with the other 1960s fire sites. Eastings and northings were recorded from the north-eastern corner of each plot. The maximum height, the widest diameter and the diameter orthogonal to the widest diameter were recorded for all individuals of O. acicularis, Orites revolutus, Coprosma nitida, Tasmannia lanceolata, Richea scoparia, Ozothamnus rodwayi, O. hookeri and O. ledifolius. Nine 1 m × 1 m quadrats were arranged in a cross formation within the 5 m × 5 m plot and the covers of all vascular plant species, bare ground and rock, moss and lichen, and litter were recorded in each. Cover estimates were aided by 100 grid squares within the quadrat. The cover of individual species with less than 0.5% cover was recorded as 0.1%.
The papers of Martin (1940) and Ratkowsky and Ratkowsky (1976) were used to gain an understanding of the species composition and abundance of species in the study area prior to 1998. This information was used to understand the temporal changes in lifeform species richness and cover. The two studies recorded abundance of taxa by zone, rather than by quadrat or plot. Cover estimates for species were made for the one small patch of alpine vegetation that has not been burnt in 1947 or later. This patch was in a block stream adjacent to a large parking area on the Pinnacle Road (GDA94 MGA55 518074E 5251461N) and was 24 m × 4 m, running upslope.
Data analysis
Species richness was calculated at the plot level (the large 5 m × 5 m plot) and the quadrat level (the nine 1 m × 1 m quadrats) as well as for all plots. Covers for the dominant shrub species (O. acicularis, O. revolutus, C. nitida, T. lanceolata, R. scoparia, O. rodwayi, O. hookeri and O. ledifolius) were established by calculating the total area of the shrub species per quadrat. The two diameter measurements were averaged and divided in half to obtain a radius for the calculation of the area of a circle. Height-class histograms were also created.
For all other taxa and ground covers (bare ground and rock, moss and lichen, and litter), percentage cover was calculated using the mean percentage cover values from the nine 1 m × 1 m quadrats. The mean percentage covers for taxa, lifeforms and bare ground were calculated for both the 1962- and 1947-burnt areas. The significance of variation in frequency of each taxon with sufficient data between each of year and burn year was determined using Fisher’s exact test. The significance of variation in slope rank between the presence and absence of each taxon was determined using one-way ANOVA.
A divergence index was calculated for each variable following Kirkpatrick et al. (2002a). The first step in the calculation of the index was to divide the highest of the two fire-year values in each data-collection year by the lowest of the two values. The 2022 ratio was then divided by the 1998 ratio to give the index. A species, lifeform, or ground cover was said to converge (i.e. become more similar over time) between the 1947 and 1962 burnt areas if the values were <1, and was said to diverge (i.e. become less similar over time) if the result was >1.
The influence of fire-history, year and slope on lifeform cover was tested using a general linear model following the default options in Minitab16 (no transformation, adjusted sums of squares, two-sided 95% confidence intervals) at both the plot and the quadrat level. The normalities of the residuals were examined to ensure the veracity of the models. The factors in the models were the year of sampling and the year of last fire. The models also included the interaction between the two. The rank of position on slope was used as a covariate in the models. There was less than 10 m difference in elevation across all the sites and a coarse contour interval, so the sites were ranked (1 was designated the lowest (furthest down slope) and 30 the highest (furthest up slope)). Fisher’s exact test was used to test the influences of each of fire history and year on the presence or absence of individual taxa. The influence of slope rank on presence or absence was tested using ANOVA. The Mann–Whitney U-test was used to test the effect of slope on species presence among survey years. Floristic data were ordinated using two-dimensional non-metric multidimensional scaling (NMDS) so as to establish the relationships among floristic composition, fire history, year and slope. The input to the ordination was the percentage frequency of each taxon in the 5 m × 5 m plots. The ordination was randomised until the smallest acceptable stress was reached. Dissimilarity was calculated using the Bray–Curtis distance measure. The relationship between composition and the environmental variables was established by fitting directional vectors of maximum correlation through the ordination space.
To test whether the boundary between the 1947 and 1962 burns represented an environmental break rather than being a product of the winds on the 1967 fire day, we determined the number of species the frequencies of which were significantly different by each fire age. Within each of these two groups of species, we recorded those that were significantly related to slope rank at the high and low ends of the ranking. This process created a two by two table defined by fire year and low or high slope ranks. Fisher’s exact test was used to determine whether the values in the four cells deviated from random; if they did, the boundary would have had an environmental cause, because slope rank reflects variation in soil drainage and rockiness, two strong influences on alpine vegetation (Kirkpatrick 1997).
The temporal change in the species richness of lifeforms for the study area was determined by calculating the percentage of lifeforms within the species lists given for the study area in Martin (1940) and Ratkowsky and Ratkowsky (1976), as well as for the lists for the 1998 and 2022 plot surveys.
NMDS ordination was performed using R Studio (ver. 22.07.2, Posit, PBC, https://posit.co/download/rstudio-desktop/). General linear models were performed using Minitab (ver. 16 and 21, Minitab LLC, https://www.minitab.com/). Vectors were fitted to the ordination in DECODA (Minchin 1990).
Results
Underlying environmental effects
Six taxa were significantly associated with the 1962 fire and were also significantly associated with the poorly drained extreme of the slope gradient, three were associated with the 1947 fire and the poorly drained extreme of the slope gradient, two were related to the 1962 fire and the sharply drained extreme, and three were associated with the 1947 fire and the sharply drained extreme. These numbers were very close to expected values (Fisher’s exact test, P = 0.5804).
Change in rates of change
Total cover and total overlapping cover exhibited a steady decline in mean annual increase, both rates being positive but negligible by 75 years after fire (Table 1). Bare ground and rock also decreased in rate of change, with the opposite absolute tendency in percentage cover (Table 1). Upright shrubs had an increase in their rate of increase until 50 years, then the rate decreased into negative by 75 years (Table 1). Mat shrubs had an increasing rate of increase until 31 years, then largely declined in their cover (Table 1). Monocots declined from a peak rate of increase from 0 to 16 years, to increasingly negative values at a decreasing rate (Table 1). Herbs declined in their rate of change then increased again, as their cover decreased after 50 years.
Item | Value | ||||||
---|---|---|---|---|---|---|---|
Measurement period | 1962–1978 | 1947–1978 | 1978–1998 | 1978–1998 | 1998–2022 | 1998–2022 | |
Years since burn | 16 | 31 | 36 | 51 | 60 | 75 | |
Percentage cover | |||||||
Bare ground + rock | −3.28 | −1.09 | −1.48 | −1.05 | −0.28 | −0.13 | |
Upright shrub | 1.58 | 1.56 | 1.96 | 2.48 | 0.26 | −0.11 | |
Mat shrub | 0.45 | 0.69 | −0.03 | −0.62 | 0.13 | −0.08 | |
Herb and fern | 0.68 | 0.31 | −0.10 | −0.10 | −0.32 | −0.30 | |
Grass and gram | 2.85 | 1.38 | −1.11 | −1.12 | −0.40 | −0.47 | |
Total cover | 2.98 | 2.14 | 1.48 | 1.05 | 0.28 | 0.13 | |
Total overlapping cover | 5.58 | 3.95 | 0.69 | 0.65 | −0.33 | −0.96 | |
Bellendena montana | 0.01 | 0.09 | 0.15 | −0.03 | −0.02 | 0.01 | |
Epacris serpyllifolia | 0.37 | 0.35 | 1.17 | 1.08 | 0.17 | 0.05 | |
Orites acicularis | 0.04 | 0.46 | 0.31 | 0.66 | 0.30 | −0.18 | |
Orites revolutus | 0.17 | 0.10 | 0.06 | 0.11 | 0.13 | 0.18 | |
Ozothamnus hookeri | 0.05 | 0.05 | 0.11 | −0.03 | −0.12 | −0.03 | |
Ozothamnus ledifolius | 0.40 | 0.02 | −0.17 | 0.00 | −0.05 | 0.10 | |
Ozothamnus rodwayi | 0.08 | 0.12 | 0.14 | 0.48 | −0.07 | −0.44 | |
Richea scoparia | 0.01 | 0.05 | 0.02 | 0.04 | 0.10 | 0.20 |
Temporal change in differences between fire ages
Total cover, total overlapping cover, bare ground, tall shrubs, mat shrubs and rock became more similar in their covers between fire ages over the last two measurements, whereas cryptogams and non-woody vascular plants became less similar (Table 2). Ten cover variables became more similar between the two burns in both time periods, whereas 13 diverged in at least one time period (Table 2).
Item | Value | ||||||||
---|---|---|---|---|---|---|---|---|---|
Divergence index | 1978–1998 | 1998–2022 | |||||||
Year of data collection | 1978 | 1978 | 1998 | 1998 | 2022 | 2022 | |||
Years since fire (burn year) | 16 (1962) | 31 (1947) | 36 (1962) | 51 (1947) | 60 (1962) | 75 (1947) | |||
Cover (%) | |||||||||
Exocarpos humifusus | 0 | 0 | 0.01 | 0.09 | 0.33 | 0.53 | n.a. | 0.14 | |
Ozothamnus rodwayi | 1.3 | 3.73 | 4.05 | 13.39 | 2.48 | 2.94 | 1.16 | 0.36 | |
Ozothamnus ledifolius | 6.4 | 0.73 | 3.05 | 0.73 | 1.83 | 3.07 | 0.48 | 0.40 | |
Orites acicularis | 0.63 | 14.33 | 6.79 | 27.43 | 13.92 | 23.01 | 0.18 | 0.41 | |
Coprosma nitida | 0 | 0 | 0.01 | 0.12 | 0.01 | 0.08 | n.a. | 0.55 | |
Ozothamnus hookeri | 0.87 | 1.67 | 3.15 | 1.12 | 0.29 | 0.46 | 1.46 | 0.58 | |
Monotoca empetrifolia | 1.03 | 0.43 | 1.17 | 0.67 | 1.13 | 1.07 | 0.73 | 0.61 | |
Richea scoparia | 0.13 | 1.4 | 0.61 | 2.15 | 2.89 | 7 | 0.33 | 0.69 | |
Rubus gunnianus | 0 | 0 | 0.06 | 0.03 | 0.06 | 0.04 | n.a. | 0.75 | |
Bellendena montana | 0.17 | 2.87 | 3.16 | 2.24 | 2.79 | 2.39 | 0.08 | 0.76 | |
Pentachondra pumila | 0.8 | 8.67 | 2.21 | 4.35 | 3.41 | 2.09 | 0.18 | 0.83 | |
Acaena montana | 0.47 | 0.33 | 0.55 | 0.3 | 0.31 | 0.19 | 1.29 | 0.89 | |
Epacris serpyllifolia | 5.97 | 10.93 | 29.29 | 32.49 | 33.38 | 33.65 | 0.61 | 0.91 | |
Leptospermum rupestre | 0 | 0 | 0.68 | 1.37 | 3.36 | 1.78 | na | 0.94 | |
Poa spp. | 38.9 | 35 | 20.56 | 19.54 | 8.82 | 8.45 | 0.95 | 0.99 | |
Orites revolutus | 2.7 | 3.0 | 3.85 | 5.13 | 7.05 | 9.43 | 1.16 | 1.01 | |
Celmisia asteliifolia | 4.1 | 5.57 | 6.74 | 6.84 | 0.24 | 0.19 | 0.74 | 1.25 | |
Montitega dealbata | 5.67 | 11.73 | 2.46 | 2.69 | 1.29 | 1.79 | 0.53 | 1.27 | |
Richea sprengelioides | 5.13 | 6.2 | 6.26 | 9.18 | 5.06 | 10.68 | 1.21 | 1.44 | |
Planocarpa petiolaris | 1.07 | 1.27 | 1.31 | 2.72 | 0.32 | 2.74 | 1.75 | 4.14 | |
Carpha alpina | 0.33 | 0.2 | 0.68 | 0.25 | 2.75 | 0.04 | 1.59 | 25.28 | |
Bare ground | 19 | 12.5 | 2.41 | 1.03 | 1.2 | 0.85 | 1.54 | 0.60 | |
Tall shrub | 25.34 | 48.47 | 64.45 | 98.15 | 70.76 | 95.49 | 0.79 | 0.90 | |
Mat shrub | 7.5 | 21.5 | 6.52 | 9.16 | 9.52 | 7.25 | 0.48 | 0.93 | |
Rock | 33.4 | 21.2 | 20.42 | 11.75 | 14.96 | 8.88 | 1.1 | 0.97 | |
Cryptogams | n.a. | n.a. | 10.93 | 6.18 | 10.13 | 4.82 | n.a. | 1.19 | |
Grass, sedge, and rush | 45.56 | 42.83 | 23.29 | 20.51 | 13.68 | 9.22 | 1.06 | 1.30 | |
Herb and fern | 1.94 | 9.74 | 8.89 | 7.79 | 1.22 | 0.66 | 1.02 | 1.71 | |
Total cover | 47.6 | 66.3 | 77.17 | 87.22 | 83.84 | 90.27 | 0.81 | 0.96 | |
Total overlapping cover | 89.34 | 122.5 | 103.15 | 135.61 | 95.18 | 112.62 | 0.96 | 0.90 |
n.a., not available because of zero values in a fire age in 1978 or 1998 or lack of measurement.
There was no relationship between lifeform class and the divergence index between 1998 and 2022 (ANOVA, F = 0.193,17, P = 0.93). For example, although tall-shrub cover converged overall between 1998 and 2022, O. rodwayi, O. ledifolius, O. acicularis, C. nitida, O. hookeri, R. scoparia, Bellendena montana and Epacris serpyllifolia all converged, whereas O. revolutus, Richea sprengelioides and Planocarpa petiolaris diverged (Table 2). Of the mat shrubs, Exocarpos humifusus, Monotoca empetrifolia, Pentachondra pumila and Leptospermum rupestre converged, whereas Montitega dealbata diverged (Table 2). Of the herbs, Rubus gunnianus and Acaena montana converged, and Celmisia asteliifolia diverged. Of the monocotyledons, Poa spp. converged and Carpha alpina diverged (Table 2).
Dynamics of tall shrubs
There was a strong decline in the number of tall shrub individuals between 1998 and 2022 in both burn ages (Table 3), contrasting with constancy in tall-shrub cover in both burn ages (Table 2). Between 1998 and 2022, tall shrubs became taller (Fig. 2). Shrub recruitment almost ceased between the two dates (Fig. 2). There was strong evidence of continuing species turnover. Fire age was the only significant component in the model for tall shrubs (Table 4).
Species | 1998 | 2022 | |||
---|---|---|---|---|---|
1962 burn | 1947 burn | 1962 burn | 1947 burn | ||
Orites acicularis | 100 | 151 | 50 | 64 | |
Orites revolutus | 44 | 37 | 29 | 69 | |
Bellendena montana | 134 | 117 | 99 | 55 | |
Richea scoparia | 29 | 14 | 40 | 24 | |
Ozothamnus rodwayi | 313 | 356 | 98 | 120 | |
Ozothamnus hookeri | 67 | 46 | 5 | 8 | |
Ozothamnus ledifolius | 59 | 7 | 20 | 16 | |
Coprosma nitida | 1 | 5 | 0 | 1 | |
Tasmannia lanceolata | 1 | 1 | 2 | 0 | |
Olearia algida | 62 | 49 | 0 | 0 | |
Total | 810 | 783 | 343 | 357 |
Cover type | Fire history | Year | Slope | Year × fire history | R2 (%) | |
---|---|---|---|---|---|---|
Cryptogams | ** | n.s. | *** | n.s. | 35.21 | |
Rock and bare ground | *** | n.s. | n.s. | n.s. | 26.53 | |
Tall shrub | *** | n.s. | n.s. | n.s. | 36.78 | |
Mat shrub | n.s. | n.s. | * | n.s. | 10.93 | |
Grass | n.s. | *** | n.s. | n.s. | 48.62 | |
Sedge and rush | n.s. | n.s. | * | n.s. | 12.96 | |
Herb | n.s. | *** | n.s. | n.s. | 44.98 | |
Richness | n.s. | *** | n.s. | n.s. | 47.53 |
The models have fire history (fixed), year (fixed), slope (fixed) and year by fire history as the explanatory variables. ***P ≤ 0.001; **P > 0.001–0.01; *P = 0.01–0.05; n.s., not significant.
The Asteraceae shrubs, O. ledifolius, O. hookeri, O. rodwayi and Olearia algida, all reduced in numbers between 1998 and 2022 (Table 3). The last of the listed species, Olearia algida, completely disappeared from the plots, despite there being 111 individuals in 1998 (Table 3). O. hookeri and O. rodwayi declined in cover in both burn ages between 1998 and 2022, whereas O. ledifolius decreased in the 1962 burn and increased in the 1947 burn (Table 2).
O. acicularis, the dominant shrub in most plots in 2022, steadily increased its cover in the 1962-burnt plots, but slightly decreased in cover between 1998 and 2022 in the 1947-burnt plots. Its numbers and cover were higher in the 1947-burnt plots than the 1962-burnt plots in all years (Tables 2, 3). Recruitment of new individuals had ceased by 2022 in both burn ages (Fig. 3).
The most numerous tall shrub overall was B. montana, which decreased in density between 1998 and 2022 (Table 3), while having new recruits in the 1962 burn (Fig. 4). Between 1998 and 2022, there was little variation in cover of the species between years or the two burns (Table 2). R. sprengelioides increased marginally in the 1947-burnt plots and decreased in the 1962-burnt plots between 1998 and 2022 (Table 2). P. petiolaris had a marginal increase in the 1947-burnt area and decreased in the 1962-burnt area (Table 2).
The covers of R. scoparia, E. serpyllifolia and O. revolutus increased overall between 1998 and 2022 (Table 2). The numbers of R. scoparia and O. revolutus increased between 1998 and 2022 (Table 3).
Dynamics of other lifeforms
The overall pattern of change between 1998 and 2022 in the lower strata was for a strong decrease in grass cover, herb cover and species richness and constancy in sedge, rush, mat shrub and cryptogram cover (Table 2).
Mat-shrub cover was stable through time in the 1947-burnt plots, but declined in the 1962-burnt plots (Table 2). The model for the 1998–2022 data for mat shrubs had only the covariate of slope position as a significant component. Mat shrubs had greater cover on the upper slopes. The covers of all mat shrubs increased from 1998 to 2022, except for P. pumila and M. dealbata, which declined but only a little (Table 2). The covers of E. humifusus and L. rupestre increased in both fire-age classes between 1998 and 2022, whereas the covers of M. empetrifolia, P. pumila and M. dealbata decreased in both fire-age classes (Table 2).
Sedge and rush cover also had only slope position as a component in its model (Table 4), decreasing in cover upslope. C. alpina declined from 0.25% to 0.04% in the 1947-burnt area and increased in the 1962-burnt area from 0.68% to 2.75% (Table 2).
Grass cover responded only to year in the model (Table 4), with a marked decrease between 1998 and 2022 (Table 2). Poa spp. declined in both fire-age classes, from 19.54% to 9.45% in the 1947-burnt area and from 20.56% to 8.82% in the 1962-burnt area (Table 2).
Herb cover had the same pattern as grass cover (Table 4), with a dramatic decrease between 1998 and 2022 (Table 2). R. gunnianus increased very marginally from 0.03% to 0.04% in the 1947-burnt area and remained at 0.06% cover in the 1962-burnt area (Table 2). A. montana declined in both fire-age classes, as did C. asteliifolia (Table 2).
The model for cryptogam cover had fire age and slope position as significant components (Table 4). Cryptogams had most cover on the lower slopes and in the 1962-burnt area.
Changes in species composition
Fifteen taxa differed by fire age (Table 5), 23 by year and 27 by slope rank. The numbers of taxa that preferentially occurred on high- and low-slope ranks and in different burn-year areas were closely similar. There were many more species that were less frequent in the 2022 samples than in the 1998 samples than vice versa (Table 5).
Species | Fire history | Sampling year | Slope (average score) | |||||||
---|---|---|---|---|---|---|---|---|---|---|
1947 | 1962 | P | 1998 | 2022 | P | Presence | Absence | P | ||
Abrotanella forsteroides | 0.37 | 5.93 | <0.001 | 1.11 | 5.19 | 0.01 | 5.17 | 15.62 | <0.001 | |
Acaena montana | 7.78 | 12.96 | n.s. | 13.33 | 7.41 | 0.03 | 13.91 | 15.46 | n.s. | |
Acrothamnus montanus | 1.48 | 5.56 | 0.01 | 6.3 | 0.74 | <0.001 | 19.63 | 15.14 | 0.02 | |
Agrostis spp. | 2.59 | 5.19 | n.s. | 6.3 | 1.48 | 0.006 | 18.43 | 15.73 | 0.08 | |
Bellendena montana | 30.37 | 31.11 | n.s. | 30 | 31.48 | n.s. | 9.29 | 17.96 | <0.001 | |
Carpha alpina | 8.89 | 30 | <0.001 | 21.48 | 17.41 | n.s. | 10.2 | 16.53 | <0.001 | |
Celmisia asteliifolia | 72.22 | 74.81 | n.s. | 95.56 | 51.48 | <0.001 | 15.48 | 14.77 | n.s. | |
Craspedia spp. | 7.78 | 14.07 | 0.02 | 18.15 | 3.7 | <0.001 | 8.61 | 16.12 | <0.001 | |
Deyeuxia spp. | 5.93 | 4.07 | n.s. | 7.04 | 2.96 | 0.04 | 21.93 | 14.95 | <0.001 | |
Empodisma minus | 0.37 | 8.15 | <0.001 | 4.81 | 3.7 | n.s. | 9.39 | 15.56 | 0.001 | |
Epacris serpyllifolia. | 93.33 | 91.85 | n.s. | 92.59 | 92.59 | n.s. | 14.99 | 19.1 | 0.003 | |
Euchiton spp. | 3.7 | 2.59 | n.s. | 6.3 | 0 | <0.001 | 22.53 | 15.06 | <0.001 | |
Euphrasia spp. | 27.78 | 5.56 | <0.001 | 16.3 | 17.04 | n.s. | 11.12 | 16.13 | <0.001 | |
Exocarpos humifusus | 2.59 | 8.15 | 0.006 | 2.59 | 8.15 | 0.006 | 6.54 | 14.97 | <0.001 | |
Gentianella diemensis | 0.37 | 5.19 | n.s. | 5.56 | 0 | <0.001 | 9.93 | 15.45 | 0.01 | |
Hierochloe fraseri | 5.56 | 0 | <0.001 | 5.56 | 0 | <0.001 | 12.07 | 15.39 | n.s. | |
Leptospermum rupestre | 6.67 | 4.81 | n.s. | 4.07 | 7.41 | n.s. | 25.55 | 14.67 | <0.001 | |
Luzula spp. | 12.22 | 21.85 | 0.004 | 32.96 | 1.11 | <0.001 | 18.57 | 14.62 | <0.001 | |
Monotoca empetrifolia | 16.3 | 22.96 | n.s. | 22.22 | 17.04 | n.s. | 21.65 | 13.74 | <0.001 | |
Montitega dealbata | 16.3 | 14.44 | n.s. | 20.74 | 10 | <0.001 | 12.94 | 15.72 | 0.006 | |
Olearia algida | 7.41 | 11.85 | n.s. | 19.26 | 0 | <0.001 | 8.44 | 16.03 | <0.001 | |
Olearia ledifolia | 4.07 | 3.7 | n.s. | 6.67 | 1.11 | 0.001 | 24.95 | 14.9 | <0.001 | |
Orites acicularis | 61.11 | 36.67 | <0.001 | 51.85 | 45.93 | n.s. | 13.25 | 17.26 | <0.001 | |
Orites revolutus | 26.67 | 23.33 | n.s. | 22.59 | 27.41 | n.s. | 22.08 | 13.04 | <0.001 | |
Ozothamnus hookeri | 5.19 | 13.33 | 0.005 | 14.44 | 4.07 | <0.001 | 11.82 | 15.65 | 0.002 | |
Ozothamnus rodwayi | 48.52 | 35.56 | 0.002 | 54.44 | 29.63 | <0.001 | 10.96 | 18.44 | <0.001 | |
Pentachondra pumila | 40.37 | 40.37 | n.s. | 47.04 | 33.7 | 0.002 | 15.29 | 15.3 | n.s. | |
Planocarpa petiolaris | 18.15 | 10 | 0.009 | 17.04 | 11.11 | n.s. | 19.93 | 14.54 | <0.001 | |
Plantago tasmanica | 5.56 | 5.19 | n.s. | 10.37 | 0.37 | <0.001 | 23.48 | 14.83 | <0.001 | |
Poa spp. | 94.07 | 87.04 | 0.007 | 98.15 | 82.96 | <0.001 | 15.68 | 11.57 | 0.001 | |
Richea scoparia | 16.3 | 13.33 | n.s. | 8.89 | 20.74 | <0.001 | 16.21 | 15.14 | n.s. | |
Richea sprengelioides | 40 | 29.63 | 0.01 | 37.78 | 31.85 | n.s. | 19.64 | 12.98 | <0.001 | |
Rubus gunnianus | 11.48 | 15.19 | n.s. | 17.78 | 8.89 | 0.003 | 15.16 | 16.18 | n.s. | |
Schizacme montana | 19.63 | 19.26 | n.s. | 38.89 | 0 | <0.001 | 16.61 | 14.98 | n.s. |
The influence of fire history and year were tested using Fisher’s exact test. The columns for fire history and year show the percentage frequency of taxa in all quadrats as well as the P-value. The influence of slope rank was tested using ANOVA. Presence values indicate the location on the slope (1 being the lowest on slope, 30 being the highest) at which the individual taxa is most common, with the values in the table corresponding to the mean slope class. P-values < 0.05 are shown in bold; n.s., not significant.
The ordination attained a minimum stress of 0.19 in two dimensions. The vectors indicated that variation in floristic composition was best explained by year (R = 0.56, P ≤ 0.001) (Fig. 5), followed by slope rank (R = 0.54, P ≤ 0.001) (Fig. 6). There was no relationship between fire history and floristic composition (R = 0.02, P = 0.43) (Fig. 5).
The long-unburnt vegetation patch
The dominant species (~80% cover) in the long-unburnt patch was the gymnosperm, Podocarpus lawrencei, a species not found in any of our plots. Other abundant shrubs were C. nitida, Orites revoluta and T. lanceolata. The less abundant shrubs were Trochocarpa thymifolia, E. humifusus, Cyathodes straminea, Leptecophylla parvifola, E. serpyllifolia and M. empetrifolia. The shrub layer was less than 1 m tall. There were very small covers of the non-woody plants, including Astelia alpina, Poa spp., Uncinia compacta, Lycopodium laterale and Polystichum proliferum.
Changes in diversity
Of the 88 species recorded in the plots in both 1998 and 2022, only 11 were not observed in the study area in either 1939 or 1976 (Supplementary Appendix 1). There was a decline and increase in shrubs, and a corresponding increase and decline in herbs, as a percentage of the overall species mix between 1939 and 2022 (Fig. 7).
Fifty-four taxa were recorded in the 2022 plots, a decline from the 76 taxa recorded in 1998 (Supplementary Appendix 1). Of the 29 species only found in 1998, 18 were herbs, 6 were grasses, sedges, or rushes, 4 were shrubs, and one was a cushion. Of the six species found only in 2022, four were herbs and two were shrubs. Species richness declined between 1998 and 2022 at the plot level (ANOVA, F = 47.641,29, P ≤ 0.001, Table 6). There was no relationship between plot species richness and fire history in either 1998 or 2022.
Year | Quadrat (1 m2) | Plot (25 m2) | All plots (750 m2) | |
---|---|---|---|---|
1998 | 9.4 | 19.9 | 76 | |
2022 | 6.1 | 13.1 | 54 |
There was a decline in species richness at the quadrat level (ANOVA, F = 393.981,539, P ≤ 0.001, Table 6). There was a relationship between fire history and richness in the 1998 quadrats (ANOVA, F = 5.361,539, P = 0.02), with the 1962-burnt quadrats having the greater species richness. There was no relationship between fire history and richness in 2022.
Discussion
Temporal patterns in rates of change
The decline in the mean annual rate of change of total cover, total overlapping cover and bare ground and rock between 1998 and 2022 was consistent with our hypotheses and other research in Tasmania (Kirkpatrick and Dickinson 1984; Kirkpatrick et al. 2010). Shrubs appeared to be the drivers of these changes, cover change slowing as they occupied a larger portion of the available space and overlapping cover change slowing as their litter and shade inhibited grass and herb growth beneath their canopies.
Upright shrubs peaked between 30 and 50 years after fire on kunanyi, contrasting with coniferous heath in which tall-shrub cover did not cease to increase over 75 years (Kirkpatrick et al. 2010), suggesting that angiosperm heath behaves differently from gymnosperm heath in Tasmania. Only one gymnosperm species is found on alpine vegetation on kunanyi. P. lawrencei is a fire-sensitive species that survives on fire-prone mountains, such as kunanyi and the Snowy Mountains, in block streams. It is dispersed by birds, which have carried it up to 300 m in 44 years from one of the two known populations that survived fire on kunanyi (Kirkpatrick and Bridle 2013). On kunanyi, it has colonised all but poorly drained sites within its dispersal range, so its potential spread is likely to continue to cause slow change in our plots if the alpine areas avoid fire for many centuries.
Differences between fire ages
The compositional difference at the quadrat level between the two burnt ages in 1998 but not 2022 indicated the predicted declining difference between burn years, as did convergence in cover for bare ground, rock, tall-shrub cover, mat-shrub cover, total cover and total overlapping cover. However, divergence between the two fire ages occurred in other lifeforms and many species between 1998 and 2022, possibly explicable as a response to the patterns of change in cover of tall shrubs, which strongly influence habitat availability for ground-stratum species and lifeforms.
Dynamics of tall shrubs
The study site differs from other sites in alpine Australia that have observed an expansion of shrub cover in that it has historically been shrub-dominated when fire has been excluded (Martin 1940). The persistent difference in shrub cover between fire-age classes, and the slowing rate of shrub expansion in the 1962-burnt area, is a novel finding of the present study, given the expansion of shrubs on alpine summits in Australia (Venn et al. 2012, 2014; Verrall et al. 2021) and elsewhere (Myers-Smith et al. 2011). It may be that the dynamics of shrubs are particularly sensitive to climatic conditions in the early decades following fire.
O. acicularis, the tallest shrub in most plots, regenerates after fire from seed (Kirkpatrick 1997; Misiak 2007). The decline in its cover in the 1947-burnt area and the lack of individuals in the smallest size class in 2022, indicate an aging population (Enright et al. 2015), as opposed to the continuous regeneration indicated by a reverse J curve in 1998 (Kirkpatrick et al. 2002a). Kirkpatrick et al. (2010) found that O. acicularis did not regenerate 31 and 47 years after fire on Mount Field, which is in line with the 30–50 cycle of angiosperm shrub establishment, growth and senescence found by Williams and Ashton (1988). As an obligate seed reproducer, the regeneration ability of O. acicularis is particularly dependent on the establishment of bare-ground gaps, and the decline in bare ground between 1998 and 2022 could explain the observed cessation of regeneration.
R. scoparia was one of only two species to increase in absolute number between 1998 and 2022, from 43 to 64 individuals. R. scoparia is a widespread and abundant Tasmanian alpine and subalpine heath species, and dominant in many areas of long-unburnt alpine and subalpine vegetation (Menadue and Crowden 1983; Kirkpatrick 1997). The population on kunanyi is at the south-eastern edge of its range and is correspondingly on warmer and drier sites than are other populations in Tasmania (Menadue and Crowden 1983). Kirkpatrick et al. (2002a) theorised that R. scoparia requires greater areas of open ground to establish in the warmer and drier environment of kunanyi on the basis of its greater abundance in the 1962-burnt area relative to the 1947-burnt area in the 1998 survey. However, new individuals established in both burn ages between 1998 and 2022, suggesting that it is capable of establishing in existing vegetation cover. Kirkpatrick et al. (2010) noted that there appeared to be competition for regeneration niche space between O. acicularis and R. scoparia on Mount Field. Although O. acicularis is unquestionably dominant in the study area in terms of both number of individuals and cover, it is no longer regenerating. Given that R. scoparia is dominant in sites that have had fire intervals of over a 100 years (Kirkpatrick et al. 2010), it could possibly take over as dominant if O. acicularis does not regenerate and if fire continues to be excluded.
O. revolutus was the other species that increased in number between the two survey years. This increase occurred in the 1947-burnt area, whereas numbers declined in the 1962-burnt area from 43 to 28. O. revolutus both resprouts vegetatively and regenerates from seed after fire (Kirkpatrick et al. 2002a). Seedlings were observed in areas that had previously been burnt in 1962, and adults persisted in areas of long-unburnt vegetation on the Central Plateau (Foulkes et al. 2021), indicating that this species is capable of persisting long after the 30–50 year timeframe of shrub succession as postulated by Williams and Ashton (1988), as do our observations of the long-unburnt patch on kunanyi.
R. sprengelioides, another increaser, prefers some shade and is common in the understorey in the subalpine zone (Menadue and Crowden 2000). It was more abundant with greater cover and was frequently observed under the canopy gaps of senescing shrubs during the survey.
Kirkpatrick et al. (2002a) observed that O. hookeri and O. ledifolius do not establish in long-unburnt vegetation, and that O. ledifolius was in the midst of a population crash, whereas O. hookeri continued to persist. O. hookeri and O. ledifolius have declined overall in the study area between 1998 and 2022; however, O. ledifolius has almost doubled in numbers in the 1947-burnt area, rising from 7 to 16 individuals, contrary to expectations. Its persistence in the long-unburnt sites indicates that the species is perhaps more resilient to infrequent fire than previously thought. Species with small, wind-dispersed seeds are more likely to colonise small gaps than those with larger seeds (Misiak 2007), which could explain the ongoing regeneration of O. ledifolius in the 1947-burnt area; however, this is not an explanation for its greater persistence than that of O. hookeri, given the similarities in reproductive traits between the two species (Kirkpatrick et al. 2002a). O. rodwayi had one individual in the lowest height class in the 1962-burnt area, showing that regeneration is still taking place; however, it has ceased to regenerate in the 1947-burnt area.
B. montana is capable of regenerating, with a handful of individuals in the lowest height class in the 1962-burnt area. The increase, however modest, in the populations of R. scoparia, O. revolutus and O. ledifolius demonstrates that the study area as a whole has not yet reached a stable state. It also demonstrates that some shrub species are capable of regenerating in closed heaths where bare ground is extremely limited (Table 2), consistent with the finding of Williams (1992) that seedlings in closed heaths are not necessarily more likely to be from the dominant species.
The long-unburnt patch of alpine vegetation in the blockstream is notable not only for the dominance of P. lawrencei, but also for the absences of all of the shrubs mentioned in this discussion except for O. revolutus. These absences may be due to the block stream environment, which was not sampled in our 30 fire-affected plots.
Dynamics of subsidiary lifeforms and species
Few long-term studies have focused on the herb community within alpine heath. In open heathland, approximately 50 years post-fire, herb cover continued to increase along with shrubs and graminoids (Bridle et al. 2001). In Tasmanian alpine coniferous heath, Kirkpatrick et al. (2010) found that grass and herb cover combined had a mean cover percentage of 0.9% 79 years after fire and 6.6% 49 years after fire, which indicates that sharp declines in herb cover are not necessarily uncommon with longer post-fire intervals.
The decline of all non-woody lifeform covers in the 1962-burnt area was expected as part of the successional process, as shrub cover increases and shades out or invades areas of grass and herb (Marcante et al. 2009; Cheal 2010). However, the severity of the decline is striking, particularly given that herb cover was much lower in the 1962-burnt area in 2022 than it was in the 1947-burnt area in 1998, which were at similar intervals after fire. The acceleration of herb decline over time is marked, particularly as there has been no corresponding acceleration in increase of shrub cover (Table 3). Grass, sedge and rush cover has slowed at a slower rate than has herb cover, which could indicate that they are better competitors for the limited amount of bare ground. In closed heath, shrub seedlings are more common in gaps than are grasses or herb seedlings (Williams and Ashton 1988; Williams 1992).
In the long-unburnt alpine vegetation patch in the blockstream there were even fewer non-woody plant species than in our 1947-burnt plots and their cover was much lower than in any of our plots. Again, this could be largely due to the extreme environment of the blockstream.
Changes in diversity
Many alpine ecosystems, including the Australian Alps, have recorded an increase in species richness resulting from the upward migration of lower-altitude taxa into the mountain summits with a warming climate (Gottfried et al. 2012; Verrall et al. 2021; Auld et al. 2022). This was not replicated in the present study. There were significant declines in species richness, with a reduction of diversity at the quadrat, plot and area scales, invalidating our research hypothesis.
Increases in aridity are associated with a decline in species richness in alpine areas in the warmer and drier montane ecosystems of the Mediterranean (Pauli et al. 2012). The kunanyi decline in species richness is unlikely to be linked to range contraction because of an increase in temperature, because the most alpine of the plant species, Celmisia saxifraga and M. dealbata, have persisted, whereas the altitudinally more widespread shrubs, O. algida and Baeckea gunniana, have disappeared from the plots, despite being recorded at other times.
The decline in species richness was particularly manifest in herbs and grasses, sedges and rushes. Almost all herb species still present in the 2022 survey declined in their mean cover values and no herb species increased between survey years (Supplementary Appendix 1). One of the most significant declines in herb cover was that of C. asteliifolia, which was the sixth-most abundant species in 1998 (Supplementary Appendix 1). It was commonly observed during the survey under the canopy gaps of senescing shrubs, a habitat that is commonly exploited by herb and grass species in closed heath when the availability of bare ground between plants is low (Williams and Ashton 1988). It is possible that the severe decline of C. asteliifolia is related to the decline in open ground, and we might expect to see an increase in the population of C. asteliifolia as shrub senescence increases and gaps form.
Marcante et al. (2009) theorised that high-stress environments such as alpine areas may select for longer-lived herbs with high adult survival rates from the outset of the successional process. Given the decline of so many herb species, this theory does not necessarily hold true for the study site; however, the herb species that have persisted into 2022 and which were also recorded in the study area before the 1947 fire, are all widespread and common alpine and montane species in Tasmania, many of which are colonisers of bare ground. A. montana, R. gunnianus and C. asteliifolia were also present 50 years post-fire in other areas of alpine Tasmania (Kirkpatrick et al. 2010). Tall, vigorous herb species from the Asteraceae, such as Craspedia and Celmisia, were found to be competitive in the face of environmental change in alpine areas of the mainland (Venn et al. 2011, 2012).
Species diversity can decline over successional time as late-successional species proliferate and exclude pioneering and mid-stage species (Marcante et al. 2009; Hédl et al. 2010). Species decline on kunanyi has been severe; however, it has followed the expected successional pathway, with the cover and richness of herbs, grass, sedge and rush declining as shrub cover has increased. This has not been uniform among all herb and shrub species, and those that have persisted into the late successional environment are likely to have traits that allow them to compete in an environment almost completely dominated by shrubs, by colonising bare-ground gaps, or growing in the canopy gaps of shrubs, as seen with C. asteliifolia (Connell and Slatyer 1977; Williams and Ashton 1988; Marcante et al. 2009).
The main determinant of the post-fire community composition is often the pre-fire community composition (Anderson and Romme 1991). Our data suggest that succession on kunanyi follows this initial floristics model of Egler (1954) rather than a relay floristics model, with species dropping out rather than invading in. There were species recorded in the Martin (1940) and Ratkowsky and Ratkowsky (1976) survey that were not found in the 1998 or 2022 surveys; however, given that those authors surveyed a much larger area than the more recent surveys, this does not necessarily indicate that they were truly absent from the study area in 1998 and/or 2022.
Given that Martin (1940) and Ratkowsky and Ratkowsky (1976) used non-quantitative measures of abundance in their surveys, it is difficult to deduce the changes in abundance of individual species pre- and post-fire; however, it is possible to draw some conclusions about the presence or absence of individual species, in particular to establish to what degree successional processes can explain the presence or absence of species in the later surveys.
Dichelachne spp., Centrolepis spp., Leptecophylla juniperina and Monotoca linifolia were recorded at lower elevations on kunanyi by both Martin (1940) and Ratkowsky and Ratkowsky (1976), and their presence in the study area from 1998 could therefore be due to upward migration. Brachyscome tenuiscapa and Cotula alpina were not recorded elsewhere on the mountain by either of the two authors; however, both species are known to be present on the mountain and are capable of dispersing into the study area (Kirkpatrick 1997; Jarman and Kantvilas 2017; Walker et al. 2020). Cannone and Pignatti (2014) determined that, for a number of locations in the European Alps, the increase in lower-elevation species in the alpine zone was not related to range expansion as a result of warming temperatures, but instead as a result of ‘range filling’ from species that were always capable of inhabiting the alpine zone. All the lower-elevation species are found in alpine or treeless subalpine vegetation elsewhere in Tasmania in areas with lower temperatures and higher elevations than in kunanyi; therefore, their presence in the alpine zone is more likely to be due to range filling than upward migration enabled by climatic warming.
Effects of climate change?
It is possible that the increase in wind speeds, exacerbated by an increase in temperature, is having an adverse effect on the cover of alpine shrubs, which could explain the persistent difference in shrub cover between the 1947- and 1962-burnt areas. Wind is one of the dominant factors that shape the composition and morphology of alpine ecosystems, with both the prevailing winds and localised wind patterns caused by topography and plant morphology able to affect plant growth (Wilson 1959; Fitzgerald and Kirkpatrick 2017). Wind effects the evaporation and transpiration rate of plants, with an observed increase in evaporation rates with an increasing height above the ground surface, which can lead to greater evaporation rates in tall shrubs than in groundcover vegetation (Wilson 1959; Fitzgerald and Kirkpatrick 2017). An increase in both temperature and speed of the hot, dry north-westerly winds that occur on kunanyi in the summer growing season, coupled with water stress in shallow soils, could be causing desiccation, with higher evaporation rates above the ground level having a more severe effect on shrubs than on lower-lying vegetation (Sharples et al. 2010; Fitzgerald and Kirkpatrick 2017). Wilson (1959) noted that shoots are particularly adversely affected by the effects of wind. Desiccation could cause the direct mortality of shoots, which could be an explanation for the lack of regeneration of most shrub species in the 1962-burnt area, despite sufficient areas of bare ground. Increased abrasion by wind-transported particles could also adversely affect both adult shrubs and seedlings, either by suppressing growth or causing direct damage to the individual (Wilson 1959; Lynch and Kirkpatrick 1995; Kirkpatrick et al. 2002b).
Conclusions
The alpine heath community has progressed from having a high cover of bare ground, grass, sedge and rush, and herbs in the first decades after fire, to being dominated by shrubs in the medium term, to approaching stability with high shrub cover and very low herb cover more than a half century after fire. Change has slowed and the two burn ages have converged in their vegetation characteristics, and diversity has declined at all scales. Shrub cover in the 1962-burnt area has not yet reached the levels of cover attained in the 1947-burnt area after a similar interval post-fire, possibly because of effects of higher wind speeds and temperatures. Mat shrubs, being more tolerant to high winds, may not have been as negatively affected by this increase, which could explain their expansion in the 1962-burnt area. There is a previously unsuspected possibility that R. scoparia could replace O. acicularis as the dominant shrub in the future. All species that are currently present in the study area were present in the pre-fire community in 1939, suggesting that succession follows the initial floristics model. The species that have disappeared from our plots are still common in subalpine vegetation on kunanyi, indicating that a long-term lack of fire may reduce the diversity of alpine vegetation, but not the diversity of the mountain as a whole, whereas fire can set back a number of species for many decades. It therefore seems better to exclude fire where possible, rather than engaging in planned burning. Fire exclusion would also allow the gymnosperm, P. lawrencei, to expand from its present refugium.
Many alpine areas in Australia and elsewhere have recorded an increase in species richness with warming temperatures, not observed in the present study. Some species were not found in the study area pre-fire. They may have dispersed into the study area from the lower-elevation subalpine zone; however, their ingress is more likely to be the result of range filling rather than range expansion, given that these species are known to inhabit the higher reaches of the alpine zone of Tasmania elsewhere than kunanyi.
Declaration of funding
The authors thank the Premier of Tasmania and the Department of Premier and Cabinet for awarding E-R. S. the Premier of Tasmania’s Honours Scholarship to help undertake this work.
Author contributions
Conceptualisation, J. B. K. and E-R. S.; methodology, E-R. S. and J. B. K.; formal analysis, E-R. S and J. B. K.; investigation, E-R. S. and J. B. K.; data curation, E-R. S. and J. B. K.; writing – original draft preparation, E-R. S.; writing – review and editing, J. B. K. and E-R. S.; supervision, J. B. K.; project administration, J. B. K.; funding acquisition, E-R. S. All authors have read and agreed to the published version of the paper.
References
Anderson JE, Romme WH (1991) Initial floristics in lodgepole pine (Pinus contorta) forests following the 1988 Yellowstone fires. International Journal of Wildland Fire 1, 119-124.
| Crossref | Google Scholar |
Auld J, Everingham SE, Hemmings FA, Moles AT (2022) Alpine plants are on the move: quantifying distribution shifts of Australian alpine plants through time. Diversity and Distributions 28, 943-955.
| Crossref | Google Scholar |
Bakker JP, Olff H, Willems JH, Zobel M (1996) Why do we need permanent plots in the study of long-term vegetation dynamics? Journal of Vegetation Science 7, 147-156.
| Crossref | Google Scholar |
Bridle KL, Kirkpatrick JB, Cullen P, Shepherd RR (2001) Recovery in alpine heath and grassland following burning and grazing, eastern Central Plateau, Tasmania, Australia. Arctic, Antarctic, and Alpine Research 33, 348-356.
| Crossref | Google Scholar |
Bureau of Meteorology (2022) Monthly rainfall, kunanyi (Mount Wellington Pinnacle). Available at http://www.bom.gov.au/jsp/ncc/cdio/weatherData/av?p_nccObsCode=139&p_display_type=dataFile&p_stn_num=094087 [accessed 17 October 2022]
Camac JS, Williams RJ, Wahren C-H, Hoffmann AA, Vesk PA (2017) Climatic warming strengthens a positive feedback between alpine shrubs and fire. Global Change Biology 23, 3249-3258.
| Crossref | Google Scholar | PubMed |
Cannone N, Pignatti S (2014) Ecological responses of plant species and communities to climate warming: upward shift or range filling processes? Climatic Change 123, 201-214.
| Crossref | Google Scholar |
Carr SGM (1962) The role of shrubs in some plant communities of the Bogong High Plains. Proceedings of the Royal Society of Victoria 75, 301-310.
| Google Scholar |
Churchill ED, Hanson HC (1958) The concept of climax in arctic and alpine vegetation. The Botanical Review 24, 127-191.
| Crossref | Google Scholar |
Connell JH, Slatyer RO (1977) Mechanisms of succession in natural communities and their role in community stability and organization. The American Naturalist 111, 1119-1144.
| Crossref | Google Scholar |
Egler FE (1954) Vegetation science concepts I. Initial floristic composition, a factor in old-field vegetation development. Vegetatio 4, 412-417.
| Crossref | Google Scholar |
Enright NJ, Fontaine JB, Bowman DMJS, Bradstock RA, Williams RJ (2015) Interval squeeze: altered fire regimes and demographic responses interact to threaten woody species persistence as climate changes. Frontiers in Ecology and the Environment 13, 265-272.
| Crossref | Google Scholar |
Fitzgerald NB, Kirkpatrick JB (2017) Wind distortion in alpine and subantarctic plants is constant among life forms but does not necessarily reflect prevailing wind direction. Arctic, Antarctic, and Alpine Research 49, 521-535.
| Crossref | Google Scholar |
Foulkes JA, Prior LD, Leonard SWJ, Bowman DMJS (2021) Demographic effects of severe fire in montane shrubland on Tasmania’s Central Plateau. Fire 4, 32.
| Crossref | Google Scholar |
Gottfried M, Pauli H, Futschik A, Akhalkatsi M, Barancok P, Benito Alonso JL, Coldea G, Dick J, Erschbamer B, Fernandez Calzado MR, Kazakis G, Krajči J, Larsson P, Mallaun M, Michelsen O, Moiseev D, Moiseev P, Molau U, Merzouki A, Nagy L, Nakhutsrishvili G, Pedersen B, Pelino G, Puscas M, Rossi G, Stanisci A, Theurillat J-P, Tomaselli M, Villar L, Vittoz P, Vogiatzakis I, Grabherr G (2012) Continent-wide response of mountain vegetation to climate change. Nature Climate Change 2, 111-115.
| Crossref | Google Scholar |
Green K, Pickering CM (2009) The decline of snowpatches in the Snowy Mountains of Australia: importance of climate warming, variable snow, and wind. Arctic, Antarctic, and Alpine Research 41, 212-218.
| Crossref | Google Scholar |
Harrison-Day V, Annandale B, Balmer J, Kirkpatrick JB (2016) Decadal-scale vegetation dynamics above the alpine treeline, Mount Rufus, Tasmania. Papers and Proceedings of the Royal Society of Tasmania 150, 9-18.
| Crossref | Google Scholar |
He X, Burgess KS, Yang X-F, Ahrends A, Gao L-M, Li D-Z (2019) Upward elevation and northwest range shifts for alpine Meconopsis species in the Himalaya–Hengduan Mountains region. Ecology and Evolution 9, 4055-4064.
| Crossref | Google Scholar | PubMed |
Hédl R, Kopecký M, Komárek J (2010) Half a century of succession in a temperate oakwood: from species-rich community to mesic forest. Diversity and Distributions 16, 267-276.
| Crossref | Google Scholar |
Jackson WD (1968) Fire, air, water and earth – an elemental ecology of Tasmania. Proceedings of the Ecological Society of Australia 3, 9-16.
| Google Scholar |
Jarman SJ, Kantvilas G (2017) Leptecophylla in Tasmania: a reassessment of four species. Swainsonia 31, 1-16.
| Google Scholar |
Ji F, Nishant N, Evans JP, Di Luca A, Di Virgilio G, Cheung KKW, Tam E, Beyer K, Riley ML (2022) Rapid warming in the Australian Alps from observation and NARCliM simulations. Atmosphere 13, 1686.
| Crossref | Google Scholar |
Jurasinski G, Kreyling J (2007) Upward shift of alpine plants increases floristic similarity of mountain summits. Journal of Vegetation Science 18, 711-718.
| Crossref | Google Scholar |
Kirkpatrick JB, Bridle KL (2013) Natural and cultural histories of fire differ between Tasmanian and mainland Australian alpine vegetation. Australian Journal of Botany 61, 465-474.
| Crossref | Google Scholar |
Kirkpatrick JB, Dickinson KJM (1984) The impact of fire on Tasmanian alpine vegetation and soils. Australian Journal of Botany 32, 613-629.
| Crossref | Google Scholar |
Kirkpatrick JB, Bridle KL, Wild AS (2002a) Succession after fire in alpine vegetation on Mount Wellington, Tasmania. Australian Journal of Botany 50, 145-154.
| Crossref | Google Scholar |
Kirkpatrick JB, Bridle KL, Lynch AJJ (2002b) Changes in alpine vegetation related to geomorphological processes and climatic change on Hill One, Southern Range, Tasmania, 1989–2000. Australian Journal of Botany 50, 753-759.
| Crossref | Google Scholar |
Kirkpatrick JB, Bridle KL, Dickinson KJM (2010) Decades-scale vegetation change in burned and unburned alpine coniferous heath. Australian Journal of Botany 58, 453-462.
| Crossref | Google Scholar |
Kirkpatrick JB, Nunez M, Bridle KL, Parry J, Gibson N (2017) Causes and consequences of variation in snow incidence on the high mountains of Tasmania, 1983–2013. Australian Journal of Botany 65, 214-224.
| Crossref | Google Scholar |
Kirkpatrick JB, Styger JK, Marsden-Smedley JB (2018) Impact of changes in lightning fire incidence on the values of the Tasmanian wilderness world heritage area. Papers and Proceedings of the Royal Society of Tasmania 152, 27-32.
| Crossref | Google Scholar |
Lasslop G, Brovkin V, Reick CH, Bathiany S, Kloster S (2016) Multiple stable states of tree cover in a global land surface model due to a fire–vegetation feedback. Geophysical Research Letters 43, 6324-6331.
| Crossref | Google Scholar |
Laycock WA (1991) Stable states and thresholds of range condition on North American rangelands: a viewpoint. Journal of Range Management 44, 427-433.
| Crossref | Google Scholar |
Lenoir J, Gégout JC, Marquet PA, de Ruffray P, Brisse H (2008) A significant upward shift in plant species optimum elevation during the 20th century. Science 320, 1768-1771.
| Crossref | Google Scholar | PubMed |
Lynch AJJ, Kirkpatrick JB (1995) Pattern and process in alpine vegetation and landforms at Hill One, Southern Range, Tasmania. Australian Journal of Botany 43, 537-554.
| Crossref | Google Scholar |
Marcante S, Winkler E, Erschbamer B (2009) Population dynamics along a primary succession gradient: do alpine species fit into demographic succession theory? Annals of Botany 103, 1129-1143.
| Crossref | Google Scholar | PubMed |
Martin D (1940) The vegetation of Mt. Wellington, Tasmania: the plant communities and a census of the plants. Papers and Proceedings of the Royal Society of Tasmania 74, 97-124.
| Google Scholar |
McDougall KL (2003) Aerial photographic interpretation of vegetation changes on the Bogong High Plains, Victoria, between 1936 and 1980. Australian Journal of Botany 51, 251-256.
| Crossref | Google Scholar |
McGowan H, Callow JN, Soderholm J, McGrath G, Campbell M, Zhao J-X (2018) Global warming in the context of 2000 years of Australian alpine temperature and snow cover’. Scientific Reports 8, 4394.
| Crossref | Google Scholar |
Menadue Y, Crowden RK (1983) Morphological and chemical variation in populations of Richea scoparia and R. angustifolia (Epacridaceae). Australian Journal of Botany 31, 73-84.
| Crossref | Google Scholar |
Menadue Y, Crowden RK (2000) Taxonomic revision of Richea R.Br. (Epacridaceae). Australian Systematic Botany 13, 773-802.
| Crossref | Google Scholar |
Morgan SW, Kirkpatrick JB, Di Folco M-B (2010) Wind-controlled linear patterning and cyclic succession in Tasmanian Sphagnum mires. Journal of Ecology 98, 583-591.
| Crossref | Google Scholar |
Myers-Smith IH, Forbes BC, Wilmking M, Hallinger M, Lantz T, Blok D, Tape KD, Macias-Fauria M, Sass-Klaassen U, Lévesque E, Boudreau S, Ropars P, Hermanutz L, Trant A, Collier LS, Weijers S, Rozema J, Rayback SA, Schmidt NM, Schaepman-Strub G, Wipf S, Rixen C, Ménard CB, Venn S, Goetz S, Andreu-Hayles L, Elmendorf S, Ravolainen V, Welker J, Grogan P, Epstein HE, Hik DS (2011) Shrub expansion in tundra ecosystems: dynamics, impacts and research priorities. Environmental Research Letters 6, 045509.
| Crossref | Google Scholar |
Nicholls N (2005) Climate variability, climate change and the Australian snow season. Australian Meteorology Magazine 54, 177-185.
| Google Scholar |
Niering WA (1987) Vegetation dynamics (succession and climax) in relation to plant community management. Conservation Biology 1, 287-295.
| Crossref | Google Scholar |
Parry J, Kirkpatrick JB, Marsden-Smedley J (2016) Explaining the distribution, structure and species composition of snow-patch vegetation in Tasmania, Australia. Australian Journal of Botany 64, 484-491.
| Crossref | Google Scholar |
Pauli H, Gottfried M, Dullinger S, Abdaladze O, Akhalkatsi M, Alonso JLB, Coldea G, Dick J, Erschbamer B, Calzado RF, Ghosn D, Holten JI, Kanka R, Kazakis G, Kollar J, Larsson P, Moiseev P, Moiseev D, Molau U, Mesa JM, Nagy L, Pelino G, Puscas M, Rossi G, Stanisci A, Syverhuset AO, Theurillat J-P, Tomaselli M, Unterluggauer P, Villar L, Vittoz P, Grabherr G (2012) Recent plant diversity changes on Europe’s mountain summits. Science 336, 353-355.
| Crossref | Google Scholar | PubMed |
Pickett STA, Cadenasso ML, Meiners SJ (2009) Ever since Clements: from succession to vegetation dynamics and understanding to intervention. Applied Vegetation Science 12, 9-21.
| Crossref | Google Scholar |
Ratkowsky DA, Ratkowsky AV (1976) Changes in the abundance of the vascular plants of the Mount Wellington Range, Tasmania, following a severe fire. Papers and Proceedings of The Royal Society of Tasmania 110, 63-90.
| Crossref | Google Scholar |
Scherrer P, Pickering CM (2005) Recovery of alpine vegetation from grazing and drought: data from long-term photoquadrats in Kosciuszko National Park, Australia. Arctic, Antarctic, and Alpine Research 37, 574-584.
| Crossref | Google Scholar |
Sharples JJ, Mills GA, McRae RHD, Weber RO (2010) Foehn-like winds and elevated fire danger conditions in southeastern Australia. Journal of Applied Meteorology and Climatology 49, 1067-1095.
| Crossref | Google Scholar |
Venn SE, Green K, Pickering CM, Morgan JW (2011) Using plant functional traits to explain community composition across a strong environmental filter in Australian alpine snowpatches. Plant Ecology 212, 1491-1499.
| Crossref | Google Scholar |
Venn S, Pickering C, Green K (2012) Short-term variation in species richness across an altitudinal gradient of alpine summits. Biodiversity and Conservation 21, 3157-3186.
| Crossref | Google Scholar |
Venn S, Pickering C, Green K (2014) Spatial and temporal functional changes in alpine summit vegetation are driven by increases in shrubs and graminoids. AoB Plants 6, plu008.
| Crossref | Google Scholar |
Verrall B, Pickering CM (2019) Recovery of subalpine grasslands 15 years after landscape level fires. Australian Journal of Botany 67, 425-436.
| Crossref | Google Scholar |
Verrall B, Pickering CM (2020) Alpine vegetation in the context of climate change: a global review of past research and future directions. Science of The Total Environment 748, 141344.
| Crossref | Google Scholar |
Verrall B, Green K, Pickering CM (2021) Dynamics in plant diversity and composition on Australian alpine summits over time. Biodiversity and Conservation 30, 1855-1880.
| Crossref | Google Scholar |
Wahren C-HA, Papst WA, Williams RJ (2001) Early post-fire regeneration in subalpine heathland and grassland in the Victorian Alpine National Park, south-eastern Australia. Austral Ecology 26, 670-679.
| Crossref | Google Scholar |
Wahren C-H, Camac JS, Jarrad FC, Williams RJ, Papst WA, Hoffmann AA (2013) Experimental warming and long-term vegetation dynamics in an alpine heathland. Australian Journal of Botany 61, 36-51.
| Crossref | Google Scholar |
Walker KJ, Robinson L, Donald D (2020) Cotula alpina (Asteraceae) naturalised in the British Isles. British & Irish Botany 2, 43-55.
| Crossref | Google Scholar |
Walther G-R, Beiẞner S, Burga CA (2005) Trends in the upward shift of alpine plants. Journal of Vegetation Science 16, 541-548.
| Crossref | Google Scholar |
Whinam J, Kirkpatrick JB (1994) The Mount Wellington string bog, Tasmania. Papers and Proceedings of the Royal Society of Tasmania 128, 63-68.
| Crossref | Google Scholar |
White PS (1979) Pattern, process, and natural disturbance in vegetation. The Botanical Review 45, 229-299.
| Crossref | Google Scholar |
Williams RJ (1992) Gap dynamics in subalpine heathland and grassland vegetation in south-eastern Australia. Journal of Ecology 80, 343-352.
| Crossref | Google Scholar |
Williams RJ, Ashton DH (1988) Cyclical patterns of regeneration in subalpine heathland communities on the Bogong High-Plains, Victoria. Australian Journal of Botany 36, 605-619.
| Crossref | Google Scholar |
Williams RJ, Wahren C-H, Tolsma AD, Sanecki GM, Papst WA, Myers BA, McDougall KL, Heinze DA, Green K (2008) Large fires in Australian alpine landscapes: their part in the historical fire regime and their impacts on alpine biodiversity. International Journal of Wildland Fire 17, 793-808.
| Crossref | Google Scholar |
Williams RJ, Wahren C-H, Shannon JM, Papst WA, Heinze DA, Camac JS (2012) Fire regimes and biodiversity in Victoria’s alpine ecosystems. Proceedings of the Royal Society of Victoria 124, 101-109.
| Crossref | Google Scholar |
Wilson JW (1959) Notes on wind and its effects in arctic–alpine vegetation. Journal of Ecology 47, 415-427.
| Crossref | Google Scholar |