Are orthodox Australian rainforest seeds short-lived in storage?
Karen D. Sommerville
A The Australian PlantBank, Botanic Gardens of Sydney, Mount Annan, NSW 2567, Australia.
Australian Journal of Botany - https://doi.org/10.1071/BT22104
Submitted: 2 September 2022 Accepted: 24 April 2023 Published online: 19 May 2023
© 2023 The Author(s) (or their employer(s)). Published by CSIRO Publishing. This is an open access article distributed under the Creative Commons Attribution-NonCommercial-NoDerivatives 4.0 International License (CC BY-NC-ND)
Abstract
Context: Fifty per cent of Australian rainforest species produce orthodox seeds, but little is known about their longevity in storage.
Aims: To (1) estimate the longevity of seeds of 33 rainforest species using artificial aging; (2) assess the influence of habit, habitat range (restricted to rainforest or more broadly distributed), seed characteristics (endospermy, dry weight), and collection environment (e.g. precipitation, elevation and average temperature) on longevity; (3) compare longevity of rainforest seeds to previously assessed species from non-rainforest habitats; (4) compare longevity in artificial aging to real-time longevity in storage.
Methods: Seeds were aged at 60% relative humidity and 45°C, and tested at pre-determined intervals until germination was reduced to zero. The time taken for germination to decline to 50% (p50AA) was calculated by probit analysis. Ordinary least-squares regression was used to model p50AA for rainforest and non-rainforest species against predictor variables. Values for p50AA were then compared with the actual longevity in storage at −20°C (p50RT) using Pearson’s correlation.
Key results: Species restricted to rainforest exhibited a significantly lower p50AA than species with a wider distribution or those restricted to non-rainforest habitats. Collection elevation had a significant negative influence on p50AA. In all, 14 of 33 species showed a significant decline in viability after ≤12 years in storage. Values of p50AA were not correlated with p50RT.
Conclusions: Rainforests contain a high proportion of potentially, and actually, short-lived species; however, p50AA is not a good predictor of their real-time longevity.
Implications: Rainforest species should be managed as short-lived, with viability checked at least every 5 years, until real-time longevity data indicate otherwise.
Keywords: accelerated aging, artificial aging, comparative longevity, plant conservation, rainforest seeds, real-time aging, seed banking, seed lifespan, seed storage, viability.
Introduction
Concern regarding the rapid loss of diversity from rainforest habitats around the world has led to increased interest in the conservation of rainforest plants ex situ (Brooks et al. 2009; Sommerville et al. 2017). Seed banking is one effective method of plant conservation that has been used to conserve the seed of food crops for thousands of years (Klein 2003) and, in recent decades, has been employed to conserve the diversity of wild plants from many regions around the world (Liu et al. 2018; Walters and Pence 2020). Seed banking may be used to conserve any species producing seeds that tolerate drying and storage at low temperatures, criteria that apply to an estimated 92% of seed-producing species (Wyse and Dickie 2017). Although rainforest environments are known to have a higher proportion of species producing seeds intolerant of drying than other habitats (Tweddle et al. 2003), recent research on 162 Australian rainforest species demonstrated that 74% were fully, or at least partially, tolerant of the drying required for conventional seed banking and 50% were also tolerant of storage at −20°C (Sommerville et al. 2021). These results suggested that seed banking may be more feasible for conserving rainforest species than previously expected.
Little is known about the longevity of rainforest seeds in conservation storage; however, many Australian species from other habitats have been found to be well suited to seed banking in the short to medium term (Offord et al. 2004; Crawford et al. 2007). Due to the difficulty of obtaining real-time longevity data for many species, particularly non-crop species, artificial aging studies (also known as accelerated aging) are now commonly used for estimating the longevity of wild-sourced seeds (Hay and Probert 2013; Davies et al. 2016). Some Australian species have been predicted to be incredibly long-lived using this method (Probert et al. 2009; Merritt et al. 2014a); for example, of the 172 species classified as long-lived by Colville and Pritchard (2019), 112 originated from Australia. However, there are other Australian species with significantly shorter lifespans, including terrestrial orchids (Hay et al. 2010), understorey species such as Wahlenbergia, Plantago and Minuria (Kochanek et al. 2009), some temporary wetland aquatic species such as Glossostigma and Myriophyllum (Tuckett et al. 2010) and alpine species (Satyanti et al. 2018), summarised in Merritt et al. (2021). Whereas some seeds have the capacity to last hundreds, and potentially thousands, of years under suitable conditions (Shen-Miller et al. 1995; Sallon et al. 2008), others may lose viability in less than a decade (Merritt et al. 2014a, 2014b).
In two large scale studies, Probert et al. (2009) and Merritt et al. (2014a) determined that the comparative longevity of seeds in ex situ storage was related to their structure and to the environment in which they were produced. Both studies found that seeds with endosperm were shorter-lived than non-endospermic seeds, although this has not been supported by more recent studies (Mondoni et al. 2011; Satyanti et al. 2018). Seeds from hot, dry environments (such as those found in arid parts of Australia) have been found to be longer-lived than those from cool, wet environments (Walters et al. 2005; Probert et al. 2009), and seeds from warm, wet environments have been found to be longer-lived than those from cool, dry environments (Merritt et al. 2014a); however, few species from warm, wet environments were included in these earlier studies (Merritt et al. 2014a).
In a recent study of 57 Australian alpine species, Satyanti et al. (2018) found that species in that environment with larger seed mass, or collected from higher elevations, had the shortest-lived seeds under artificial aging. Physical seed characteristics, such as seed size, embryo size and type, and plant family/phylogeny have also been considered as predictors of seed longevity (Davies et al. 2016, and references therein). Merritt et al. (2014a), for example, found that species in the Fabaceae tended to be long-lived, whereas those in the Asteraceae tended to be short-lived. However, environmental conditions prior to seed collection, level of seed maturity, and postharvest handling can all affect potential seed longevity (Probert et al. 2007; Kochanek et al. 2009), leading to intraspecific variations in the longevity of seed lots held in a genebank (Hay and Whitehouse 2017).
In this study, we used artificial aging to estimate the comparative longevity in storage of 33 rainforest species with likely orthodox seed storage behaviour (Sommerville et al. 2021). We compared longevity to habit, habitat range, seed characteristics (endospermy and dry weight), and environmental variables in the collection habitat (such as precipitation, average temperature and elevation) to determine which factors had the greatest influence on seed longevity. We then compared longevity under artificial aging to actual longevity in storage at −20°C for up to 15 years, to determine whether artificial aging provides a useful indication of real-time longevity for rainforest seeds.
Materials and methods
Seed collections
Seeds had previously been collected from 33 rainforest species growing in temperate and subtropical climate zones in eastern New South Wales (NSW), Australia (Fig. 1, Table 1). Collection elevation ranged from 5 to 1165 m a.s.l., latitude from 28°S to 34°S and longitude from 151°E to 154°E. In most cases, seeds were collected from rainforest habitats; for six species with a broader distribution, seeds were collected from open forest, swamp or gully habitats (Supplementary Table S1). Seeds for Corchorus cunninghamii were collected from plants of wild provenance cultivated at the Australian Botanic Garden, Mount Annan.
Collection locations for seed used in artificial aging experiments to estimate the comparative longevity in storage of 89 Australian plant species. Solid circles indicate rainforest species assessed in the present study; open circles indicate species assessed in a previous study (Merritt et al. 2014a) used for comparison. Shaded regions represent protected areas within the New South Wales National Parks Estate.
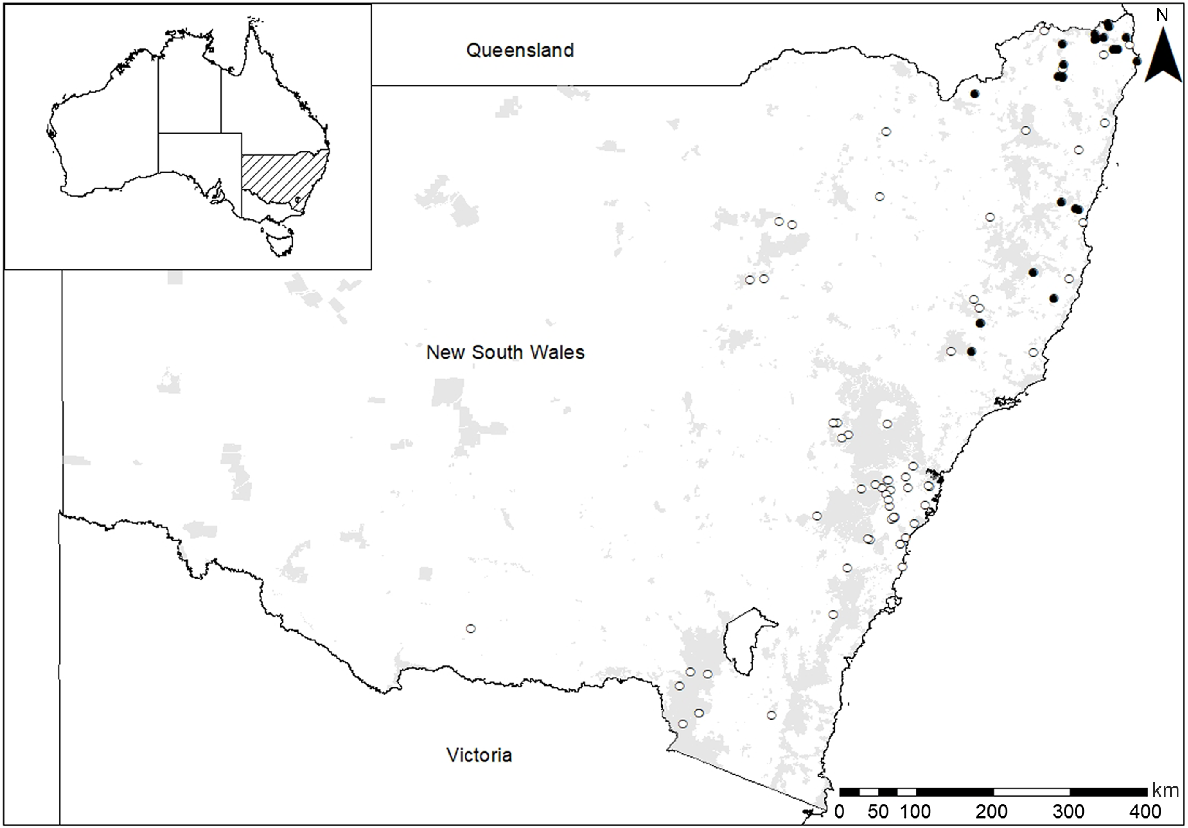
Family | Species | Habit | Habitat range | End. | Dry weight (mg) | Germination (age) | Comparative longevity | ||
Re-test 1 % (years) | Re-test 2 % (years) | p50AA (days) | p50RTA (years) | ||||||
Apocynaceae | Alstonia constricta | Shrub/tree | Littoral and dry rf, vine thickets; other | E | 1.0 | 88 (0.7) | 89 (3.7) | 43.3 | > |
Apocynaceae | Parsonsia fulva | Vine | Subtrop, warm and cool temp rf | E | 10.0 | 81 (2.6) | 89 (4.7) | 2.8 | > |
Apocynaceae | Parsonsia induplicata | Vine | Subtrop, warm and cool temp rf | E | 3.8 | 98 (0.5) | 100 (3.5) | 1.5 | > |
Araliaceae | Hydrocotyle pedicellosa | Herb | Trop rf, vine forest and rf margins | E | 0.2 | 80 (0.5) | 69 (3.3) | 0.71 | 7.8 |
Argophyllaceae | Argophyllum nullumense | Shrub/tree | Trop, subtrop and warm temp rf and rf margins | E | 0.05 | 100 (1.0) | 100 (5.3) | 10.9 | – |
Bignoniaceae | Pandorea pandorana | Vine | Trop, warm and cool temp, littoral and dry rf; other | N | 3.8 | 94 (12.1) | 93 (14.9) | 7.4 | 67.2 |
Cunoniaceae | Ackama paniculosa | Tree | Trop, subtrop, warm and cool temp rf | E | 0.1 | 84 (1.9) | 69 (6.6) | 3.7 | 11.2 |
Cunoniaceae | Ceratopetalum gummiferum | Tree | Warm temp rfB; other | E | 10.5 | 88 (8.0) | 62 (11.7) | 9.7 | 11.3 |
Cunoniaceae | Karrabina benthamiana | Tree | Subtrop and warm temp rf | N | 0.6 | 83 (0.7) | 8.8 (4.0) | 17.9 | 2.1 |
Cunoniaceae | Vesselowskya venusta | Shrub/tree | Cool temp rf | N | 0.1 | 86 (0.4) | 62 (4.4) | 24.9 | 6.0 |
Gesneriaceae | Fieldia australis | Vine | Warm and cool temp rf | N | 0.04 | 100 (0.5) | 96 (3.5) | 11.9 | 4.5 |
Lamiaceae | Plectranthus nitidus | Herb | Subtrop rf | N | 0.2 | 94 (0.5) | 80 (3.5) | 66.5 | 7.0 |
Linderniaceae | Artanema fimbriatum | Herb | Trop rf, subtrop rfB, other | E | 0.1 | 95 (2.2) | 100 (5.4) | 55.4 | > |
Malvaceae | Abutilon oxycarpum | Shrub | Subtrop and dry rf margins, vine thickets | E | 1.2 | 86 (3.6) | 92 (6.8) | 41.5 | > |
Malvaceae | Corchorus cunninghamii | Vine | Dry rf marginsC, other | E | 1.4 | 84 (0.5) | 72 (3.5) | 55.4 | 7.7 |
Menispermaceae | Stephania japonica var. discolor | Vine | Trop, subtrop, warm temp, littoral and dry rf | E | 12.9 | 78 (2.0) | 58 (5.7) | 0.8 | 6.1 |
Moraceae | Ficus fraseri | Tree | Trop, subtrop, littoral and dry rf | E | 0.5 | 100 (0.3) | 98 (2.6) | 27.8 | 3.6 |
Moraceae | Ficus henneana | Tree | Trop, subtrop, littoral and dry rf | E | 0.6 | 94 (0.4) | 98 (3.4) | 45.0 | > |
Myrtaceae | Lenwebbia prominens | Shrub/tree | Subtrop and warm temp rf | N | 17.2 | 88 (1.0) | 2 (3.5) | −1.2 | 1.9 |
Myrtaceae | Melaleuca styphelioides | Shrub/tree | Subtrop and dry rf and margins | N | 0.03 | 94 (5.3) | 53 (8.1) | 159.7 | 8.2 |
Onagraceae | Ludwigia octovalvis | Herb | Trop rf, vine forest; other | N | 0.1 | 89 (3.2) | 70 (6.4) | 125.6 | 8.8 |
Paracryphiaceae | Quintinia verdonii | Tree | Subtrop and warm temp rf | N | 0.03 | 91 (2.1) | 88 (6.8) | 2.9 | 36.4 |
Philydraceae | Helmholtzia glaberrima | Herb | Subtrop rf | E | 0.1 | 96 (2.7) | 100 (6.6) | 19.8 | > |
Pittosporaceae | Pittosporum lancifolium | Shrub/tree | Subtrop and dry rf | E | 8.4 | 88 (0.5) | 98 (3.5) | 14.0 | > |
Poaceae | Entolasia marginata | Grass | Trop and subtrop rf; otherD | E | 1.1 | 96 (4.6) | 63 (6.8) | 37.1 | 7.3 |
Poaceae | Panicum bisulcatum | Grass | Subtrop rfB, otherD | E | 0.6 | 53 (1.7)E | 69 (6.4) | 52.3 | 7.8 |
Proteaceae | Lomatia arborescens | Shrub/tree | Subtrop, warm and cool temp. rf | N | 12.8 | 100 (1.6) | 74 (5.3) | 6.4 | 5.7 |
Rousseaceae | Abrophyllum ornans | Shrub/tree | Trop, subtrop and warm temp rf | N | 0.1 | 94 (1.0) | 0 (5.3) | 3.7 | 3.1 |
Rousseaceae | Cuttsia viburnea | Shrub/tree | Subtrop and warm temp rf and margins | E | 0.04 | 86 (1.7) | 44 (6.4) | 4.8 | 5.8 |
Rubiaceae | Psychotria loniceroides | Shrub/tree | Subtrop, warm temp, littoral and dry rf | E | 6.4 | 98 (2.7) | 72 (6.4) | 15.2 | 7.9 |
Solanaceae | Nicotiana forsteri | Herb | Subtrop rfB, vine thickets, other | N | 0.1 | 94 (1.3) | 72 (5.7) | 22.0 | 8.3 |
Solanaceae | Solanum vicinum | Shrub | Subtrop, warm and cool temp rf margins | E | 0.7 | 95 (1.4) | 92 (3.5) | 19.0 | 15.8 |
Urticaceae | Boehmeria macrophylla | Shrub | Subtrop rf | N | 0.1 | 96 (1.3) | 57 (5.5) | 12.1 | 6.0 |
Data on plant habit and habitat range were derived from Harden et al. (2016), RBGDT (2020) and Zich et al. (2018) unless otherwise indicated. Habitat range refers to the full range of rainforest and other habitats occupied by the species: trop, tropical; subtrop, subtropical; temp, temperate; rf, rainforest; dry rf, seasonally dry rainforests and monsoon forests; other, any non-rainforest habitat. The column End. indicates whether seeds are endospermic (E) or non-endospermic (N). Dry weight is the mean weight of individual seeds following drying for 17 h at 103°C. Germination refers to mean percentage germination (adjusted for empty seeds) of a single seed collection held at The Australian PlantBank just prior to artificial aging (Re-test 1), and when re-tested after several years of additional storage at −20°C (Re-test 2); age refers to the number of years since the seeds were collected. Comparative longevity is the estimated time required for germination to be reduced to 50% under artificial aging (p50AA) or real-time storage (p50RT) in days or years, respectively.
AReal-time p50 values could not be calculated for species showing no change (–) or an increase (>) in germination compared to previous tests.
BBased on range of habitats (and co-occurring species) from which seed has been collected and stored at the Australian PlantBank.
CHabitat data derived from https://www.environment.nsw.gov.au/threatenedspeciesapp/profile.aspx?id=10177.
DHabitat data derived from https://ausgrass2.myspecies.info/.
ESeeds of the same age, from the same collection, germinated to 84% following trial storage for 1 month at −192°C.
Seeds were collected at the point of natural dispersal then dried at 15°C and 15% relative humidity, cleaned of debris and checked for viability by cut test (dissection of seeds to check for the presence of a healthy embryo) or germination of a subsample. The collections were then vacuum-sealed in foil packets and stored at −20°C at the Australian PlantBank. A supporting herbarium specimen for each species was lodged with the National Herbarium of New South Wales.
Data for an additional 56 species collected from non-rainforest habitats, also in temperate and subtropical climate zones in eastern NSW, were available for comparison (Fig. 1). Collection elevation for these ranged from 10 to 1950 m a.s.l., latitude from 28°S to 36°S, and longitude from 146°E to 153°E. Seeds for these species had been dried, stored and subjected to artificial aging at the Australian Plant Bank, using the same protocols and equipment described herein, as part of an earlier Australian-wide study on comparative longevity (Merritt et al. 2014a). The distributional range of four species extended to rainforest; the remainder were restricted to non-rainforest habitats (Table S1).
Seed characteristics and environmental data
The presence or absence of endosperm was determined by dissecting rehydrated seeds under a microscope. Oven dry weight for each species was determined by drying seeds for 17.5 ± 0.5 h at 103°C and weighing after cooling over silica gel. Mean dry weight was calculated from 10 replicates of individual seeds or, for small-seeded species, from three replicates of 5–100 seeds, depending on seed size.
Data on elevation, latitude and longitude were obtained using a hand-held GPS unit at the time of seed collection. Within the collection region of eastern NSW, latitude, interacting with elevation, has a strong influence on maximum and minimum temperatures, whereas longitude is strongly associated with rainfall, vapour pressure and evaporation (Bureau of Meteorology 2023).
Climatic data for each location were derived from ANUClimate 1.0, a national climatic dataset based on 30 years of recorded data interpolated to a geographic grid scale of 0.01 degrees (approximately 1 km; Hutchinson et al. 2015; Table S1). The data represented annual means for temperature (diurnal range and average, maximum and minimum temperatures) and water availability (precipitation, evaporation, vapour pressure, and the proportion of days per month in which precipitation was greater than 0.2 mm (proportion wet days)). Long-term climate data (rather than data from the year of collection) were chosen for this study because (1) brief events such as drought, flood or strong winds during flowering or seed development can affect seed quality within a given year but may not be reflected in the climate averages for that year, (2) rainforest species tend to exhibit masting, i.e. the irregular production of large fruit crops, that is likely to be related to long-term rather than annual climatic variables.
Preliminary germination testing
Preliminary germination tests were conducted to determine whether the stored seeds (ranging from 0.3 to 12.1 years in age) remained sufficiently viable for testing longevity by artificial aging. Seed packets were withdrawn from storage and held for 24 h at 15°C before the packets were opened and seed samples were removed. Subsamples of each collection were rehydrated at ambient humidity, then sown on 0.7% agar (with 0.25 g L−1 gibberellic acid, if necessary, to relieve suspected dormancy) and incubated at 20°C or 25°C with a day–night cycle of 12 h–12 h. These germination conditions matched pre-storage germination conditions for those species that had been tested prior to storage. All tests were conducted with five replicates of 10–20 seeds each. Replicates for a given species were arranged randomly within a single stack in a single incubator and re-randomised following each germination check. Seeds were considered to have germinated when the emerging radicle was at least 2 mm in length. Experiments were terminated when no further germination had occurred for at least 14 days. On termination of the experiment, seeds that failed to germinate were dissected under a microscope to determine whether they remained viable.
Comparative longevity under artificial aging
Collections with a post-storage germination percentage ≥80% (78% for Stephania japonica var. discolor) were subsampled for further testing of comparative longevity, following the procedures described in Newton et al. (2009), or Davies et al. (2016) for small collections. The collection of Panicum bisulcatum exhibited low post-storage germination, but a subsample of this collection subsequently stored at −192°C germinated to 84% (data not shown) and so the collection was considered to meet the viability criterion for artificial aging.
In preparation for the aging experiments, 10 samples of 50 seeds per species, or two samples of 25 seeds and 2 of 50 seeds (following Davies et al. 2016), were placed in open glass vials and rehydrated at 20°C and 47% relative humidity for 2 weeks. The vials of rehydrated seeds were then transferred to an artificial aging environment of 45°C and 60% relative humidity. For both rehydration and aging, seeds were held over a solution of lithium chloride, with a concentration suitable to generate the desired humidity, in an airtight electrical box. To ensure experimental conditions were comparable among seed lots and with earlier experiments, the relative humidity of rehydration and aging environments was checked using a hygrometer, and adjusted as needed, prior to commencing the experiment and periodically throughout it. Likewise, the temperature of the incubators used for rehydration and aging were checked before commencing and monitored to ensure temperatures were consistent throughout the aging process.
A single sample for each species was withdrawn from the aging environment at intervals of 1, 2, 5, 9, 20, 30, 50, 76, 100 and 126 days (following Newton et al. 2009) or 1, 10, 15 and 30 days for smaller collections (following Davies et al. 2016), and germinated under the same conditions as used for preliminary germination testing (see above). Seeds of Melaleuca styphelioides were found to be very long-lived and therefore the experiment was repeated for this species, with nine samples withdrawn at intervals of 1, 37, 79, 126, 170, 219, 272, 320 and 370 days. Only data from the latter experiment are reported for this species.
Germination was considered to have been reduced to zero for a given sample if no germination had been observed for at least 30 days after the usual commencement of germination for that species. The time taken (in days) for the germination percentage to be reduced to 50% (p50AA) was then calculated in R v4.1.1 (R Core Team 2021). To calculate p50AA, we first generated a matrix of binomial response data (number alive/dead) against time in the aging environment for each species. We then applied a general linear model to each matrix using binomial regression with a probit-link function (referred to hereafter as probit analysis), as follows:
where y1 is the response matrix.
p50AA was then estimated using the function dose.p in the MASS package (https://cran.r-project.org/web/packages/MASS/index.html), as follows:
The coding used for calculating p50AA in R was tested against a dataset provided by the Millennium Seed Bank, Kew (produced using Genstat software, https://vsni.co.uk/software/genstat), to ensure that the output was comparable with previously published datasets (e.g. Hay et al. 2010; Merritt et al. 2014a).
If germination initially increased in response to aging, data were analysed from the point where a decline in viability commenced. For Hydrocotyle pedicellosa, a steady decline in germination from Day 1 to Day 5 was followed by a sudden increase on Day 9, before declining to zero; this outlier was excluded from the calculation of p50AA.
Real-time longevity
To determine whether artificial aging provides a useful indication of real-time longevity in storage for rainforest seeds, the germinability of all 33 species was re-tested after an additional 2–4.5 years in storage (amounting to a total storage time of 3.1–14.9 years; Table 1). Experiments were conducted using the same germination conditions employed for preliminary testing and artificial aging; these were terminated when no further germination had been observed for 30 days. Cut tests were completed on all non-germinating seeds and final germination percentages were adjusted for empty seeds. For species exhibiting a significant decline in germination following storage (P < 0.05), a value for real-time p50 (p50RT) was calculated using probit analysis based on two or three data points, namely, germination after drying (Initial, if available), germination after short-term storage (Re-test 1), and germination after further storage (Re-test 2). Data on germination before storage could only be included in the calculation if there was no subsequent increase in germination following storage.
Analysis
The influence of plant habit (trees vs shrubs + shrub/trees, vines, and grasses + herbs), habitat range (occurring in rainforest only vs occurring in both rainforest and non-rainforest habitat) and endospermy (present or absent) on p50AA for the 33 previously unstudied rainforest species (Table 1) was first assessed using a Kruskal–Wallis test. Simple linear regression was then used to assess the influence of seed dry weight, and geographic and climatic variables, on log p50AA. Variables were checked for normality prior to analysis and log-transformed as needed. Normality plots and plots of residuals versus fits were used to ensure that the log-transformed data met the assumptions for linear regression. Variables displaying a significant influence on log p50AA were then selected for modelling using ordinary least squares (OLS) regression. A Pearson’s correlation matrix was first generated to identify any collinearity (r ≥ 0.7; Dormann et al. 2013); OLS was then undertaken using individually significant categorical and non-collinear variables combined with one variable from each collinear pair. All possible combinations of individually significant collinear predictors were tested to identify the most parsimonious model. Assumptions of normality and homoskedasticity were checked via residual plots and density plots, respectively.
To compare the longevity of rainforest-specialist and rainforest-generalist to non-rainforest species, data for the 33 previously unstudied rainforest species were combined with data for the 56 species assessed in an earlier study (see Seed Collections). Species were grouped into one of the following three habitat categories on the basis of their distributional range: rainforest, occurring in rainforest habitats only; rainforest and non-rainforest, occurring in both rainforest and non-rainforest habitats; and non-rainforest, occurring in non-rainforest habitats only (Table S1). Differences in p50AA among the three habitat-range categories were first explored using a Kruskal–Wallis test. Simple linear regression and OLS regression were then applied as described above to identify individually significant predictors and produce the most parsimonious model explaining variation in log p50AA. Assumptions of normality and homoskedasticity in the final model were checked via residual plots and density plots, respectively.
The non-rainforest dataset included three species with a p50AA that greatly exceeded the range of rainforest species (i.e. Allocasuarina distyla, 295.3; Dodonaea heteromorpha, 376.0; and Dodonaea viscosa subsp. spatula, 294.3; Table S1). In addition, species in the Fabaceae (n = 9) and Asteraceae (n = 13) in the non-rainforest dataset, and Cunoniaceae (n = 4) in the rainforest dataset, were over-represented compared with other families. The Kruskal–Wallis tests, and the most significant of the OLS models described above, were therefore repeated after excluding the three very long-lived species and limiting the representatives of the Fabaceae, Asteraceae and Cunoniaceae to three species from each family with the lowest, highest and mid-range p50AA values in this dataset.
Differences among post-drying and post-storage germination tests for the 33 rainforest species assessed in this study were analysed using a Kruskal–Wallis test, with treatment means separated using the Wilcox test. A real-time p50 (p50RT) was calculated for all species showing a significant reduction in germination following storage. Values of log p50RT were then compared with log p50AA using Pearson’s correlation, following Probert et al. (2009). As estimates of p50RT can be unreliable for species showing a decline in germination of less than 5–6% (Walters et al. 2005), the correlation was repeated using only those species showing a decline in germination of 10% or more.
Kruskal–Wallis and simple linear regression analyses were performed in Minitab ver. 16 (https://www.minitab.com/en-us/products/minitab/). OLS regression was performed in R v4.1.1 (R Core Team 2021).
Results
Seed characteristics, geographic and environmental data
Dry-seed weight for the 33 previously unstudied rainforest species ranged from 0.1 to 17.2 mg (Table 1). The proportions of endospermic and non-endospermic species were 61% and 39% respectively (Table 1).
Factors influencing comparative longevity of rainforest species
The p50AA of the 33 previously unstudied rainforest species ranged from −1.2 to 159.7 days (mean 27.9 ± 6.2 days; Table 1). A negative value for one species (Lenwebbia prominens) resulted from a drop in viability of more than 40% following just 1 day of aging. Because a loss of viability between preliminary testing and commencing artificial aging may have contributed to this result, the species was excluded from all analyses. For the 32 remaining species, neither habit [trees (n = 6) vs shrubs + shrub/trees (n = 12), vines (n = 6) and grasses + herbs (n = 8)] nor endospermy had a significant effect on p50AA (P = 0.099 and 0.683 respectively); however, species restricted to rainforest habitat had a significantly lower p50AA (P = 0.028) than species with a distribution extending to non-rainforest habitat.
C. cunninghamii was excluded from linear regression and subsequent modelling because of lack of precise provenance data; the following analyses were therefore conducted on 31 species. Simple linear regression of dry-seed weight and geographic and climatic variables against log(p50AA) identified only average temperature (R2 = 14.7%, P = 0.033) and log(elevation) (R2 = 25.5%, P = 0.004) as significant predictors. Correlation analysis identified a strong negative relationship between these two variables (r = −0.815, P = 0.000) therefore modelling was performed using each variable separately.
OLS regression of log p50AA against habitat range and average temperature produced an overall significant model (F2,28 = 4.808, P = 0.016) in which average temperature had a significant positive relationship with log p50AA (P = 0.034). OLS regression of log(p50AA) against habitat range and log(elevation) produced a slightly more significant model (F2,28 = 6.837, P = 0.004) in which log(elevation) had a significant negative relationship with log(p50AA) (P = 0.007). Both models showed no excessive departure from the assumptions of normality and homoskedasticity.
Comparative longevity of rainforest-specialist compared with rainforest-generalist and non-rainforest species
Comparison of the combined set of 87 species by habitat range showed that species restricted to rainforest habitats exhibited significantly lower values of p50AA than species restricted to non-rainforest habitats (P < 0.001), and species that occur in both rainforest and non-rainforest habitats (P = 0.038; Fig. 2). This difference remained when three very long-lived species were removed from the dataset (P < 0.001, n = 84) and when the data were corrected for over-representation of Asteraceae and Fabaceae in the non-rainforest species dataset and Cunoniaceae in the rainforest species dataset (P = 0.001, n = 67). There was no significant difference between non-rainforest species and species occurring in both rainforest and non-rainforest habitats (P = 0.392 and 0.974 for the full and adjusted datasets, respectively).
Influence of habitat range on the comparative longevity of seeds of native plant species from New South Wales, Australia. Habitat-range categories are as follows: rainforest, occurring in rainforest habitats only (n = 18); rainforest and non-rainforest, occurring in both rainforest and non-rainforest habitats (n = 18); and non-rainforest, occurring in non-rainforest habitats only (n = 52). Values of p50AA represent the number of days in the artificial aging environment (45°C, 60% relative humidity) needed for seeds to decline to 50% viability. Three species with very large p50AA have been excluded from the chart to simplify presentation. Different letters above the boxplots represent a significant (P < 0.05) difference in log p50AA among categories.
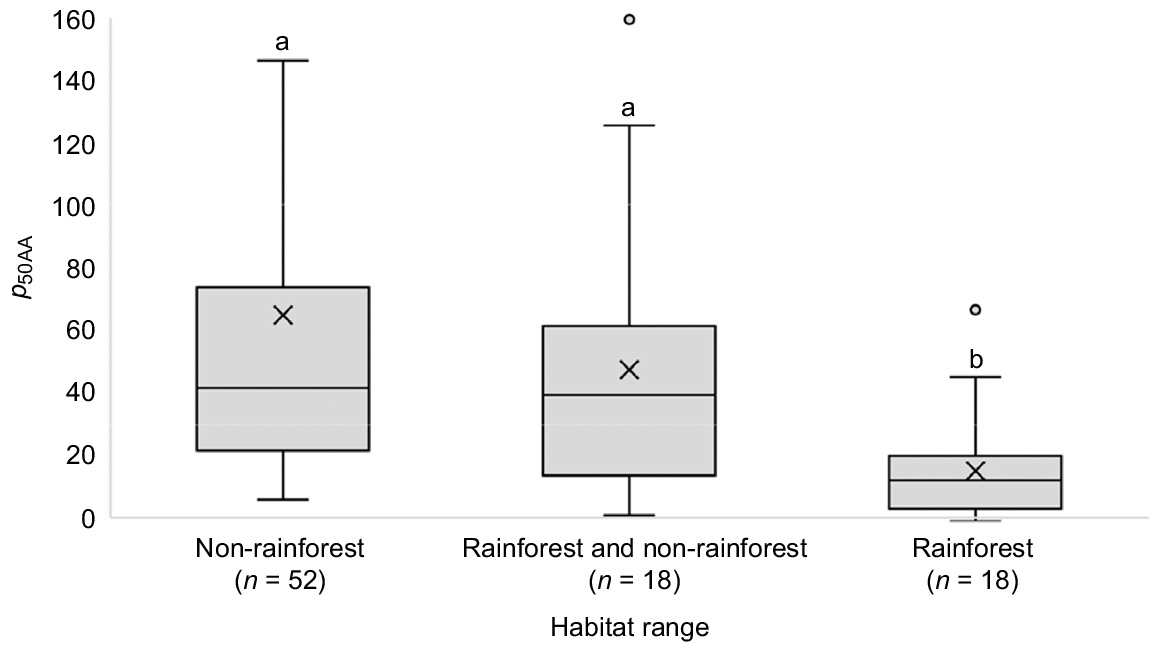
Simple linear regression of individual predictors against log p50AA identified weak but significant relationships with log(elevation) (R2 = 10.6, P = 0.002), log(latitude) (R2 = 9.7, P = 0.003), log(longitude) (R2 = 7.1, P = 0.013), log(precipitation) (R2 = 14.4, P = 0.000), diurnal temperature range (R2 = 5.8, P = 0.025) and log(proportion wet days) (R2 = 7.7, P = 0.009). Correlation analysis identified strong collinearity between log(latitude) and log(longitude) (r = −0.817, P < 0.001), between diurnal temperature range and both log(precipitation) and log(proportion wet days) (r = −0.750 and −0.826, P < 0.001 respectively), and between log(precipitation) and log(proportion wet days) (r = 0.838, P < 0.001). OLS regression was therefore performed in six variations combining habitat range and log(elevation) with log(latitude) or log(longitude) and one of diurnal range, log(precipitation) or log(proportion wet days). The best model (in terms of significance) incorporated habitat range, log(elevation), log(precipitation) and log(latitude). This model was significant overall (F5,81 = 8.928, P < 0.001) and identified significant negative relationships between the response variable and (a) restriction to rainforest habitats (P = 0.002), and (b) log(elevation) (P = 0.001). The model showed no departure from the assumptions of normality and homoskedasticity.
The above model was then applied to the revised dataset excluding the three very long-lived species and correcting for over-representation of Asteraceae, Fabaceae and Cunoniaceae. For this dataset, the overall model was once again significant (F5,61 = 7.137, P < 0.001) but log(elevation) was the only significant predictor (P = 0.002). This model showed slight departure from normality, with nine samples falling just outside the 95% confidence interval.
Artificial aging versus real-time longevity
Re-testing of the 33 previously unstudied rainforest species following additional time in storage showed that germination had declined significantly within 3–6 years for seven species, and within 6–12 years for another seven species (Fig. 3). For Entolasia marginata, H. pedicellosa and P. bisulcatum, non-germinating seeds appeared viable on termination of the experiment, indicating either induction of dormancy during storage or undetected changes in the embryo. For eight species in this group, a decrease in germination was accompanied by an increase in variation among replicates; this was most evident for species showing a significant decline within 6–12 years of storage (6 spp. of 7; Fig. 3).
Mean germination percentages for seeds of Australian rainforest plants tested immediately after drying (Initial), then re-tested after short-term storage at −20°C (Re-test 1) and longer-term storage at the same temperature (Re-test 2). The species presented exhibited a significant (P < 0.05) decline in viability in storage within (a) 3–6 years or (b) 6–12 years.
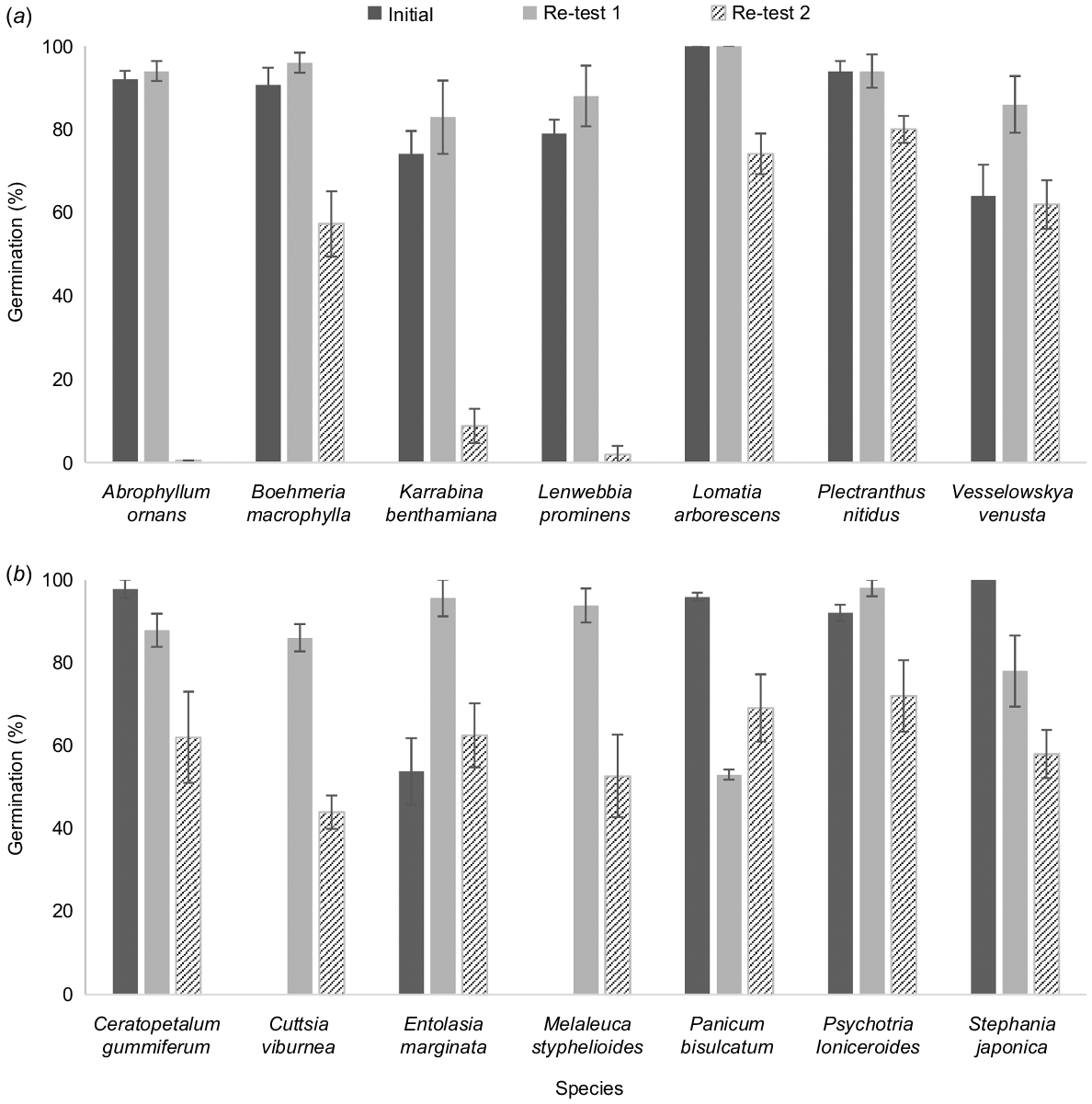
For the remaining 19 species, no significant declines in germination were detected but five species showed a reduction in germination of 10–22% (Table 1); comparatively wide variation among replicates for these species may have prevented detection of a significant decline.
Analysis of the relationship between log p50RT and log p50AA was completed using the 23 species for which it was possible to calculate p50RT (Table 1). There was no correlation between the two sets of log-transformed p50 values (Pearson’s correlation r = −0.106, P = 0.631, n = 23). Removal of five species from the real-time longevity dataset which had shown a decline of ≤6% (Ficus fraseri, Fieldia australis, Pandorea pandorana, Quintinia verdonii and Solanum vicinum) did not change the outcome (Pearson’s correlation r = 0.155, P = 0.540, n = 18). The order of lowest to highest p50 for species (i.e. the order of shortest- to longest-lived) varied between the test methods for all species except for Cuttsia viburnea, which had equal rankings via both methods.
Discussion
Understanding seed lifespan is essential to ensuring conservation collections are maintained with high viability for as long as possible (Hay and Whitehouse 2017). Those collections can then provide a valuable source of material for ongoing research and for returning species to the wild when threats have been controlled (Cochrane et al. 2007; Commander et al. 2018). Although the 33 rainforest species tested here ranged in longevity under artificial aging from very short-lived (e.g. S. japonica) to very long-lived (e.g. M. styphelioides), more than half (18 spp.) had a p50AA < 20 days and, of those, 11 had a p50AA < 10 days. These results suggest that rainforest seeds are likely to be very short-lived in storage compared with seeds from drier environments, a prediction supported by the significant reduction in viability of 11–14 species following just 12 years or less in storage.
The comparative longevity predicted by artificial aging for a few species was matched by real-time viability loss. L. prominens, for example, was predicted to be the shortest-lived species by artificial aging (p50AA = −1.2 days) and was second-shortest based on real-time aging (p50RT = 2.2 years). Although this species was not included in the correlation analysis due to the negative p50AA, the species was clearly very short-lived, with germination declining from 98% to 2% in just under 3.5 years in storage. Similarly, Abrophyllum ornans showed a rapid decline in germination from 94% after 1 year in storage to 0% after 5.2 years in storage. This species also had low values for both p50AA (3.7 days) and p50RT (1.8 years). However, comparative longevity predicted by artificial aging for the remaining species was not well supported by the real-time aging experiments. Four species estimated to be shorter lived than A. ornans by artificial aging, for example, proved to be substantially longer-lived in real-time. These included H. pedicellosa with a p50AA of 0.71 and p50RT of 7.8 years, S. japonica var. discolor with a p50AA of 0.8 and p50RT of 6.1 years, and Q. verdonii with a p50AA of 2.9 and p50RT of 36.4 years, the second-longest real-time estimate of all the species showing some decline in germination. The longest-lived species by artificial aging, M. styphelioides, had a p50AA of 159.7, yet this species has already shown a 41% decline in viability in just 8 years in storage. These examples highlighted the difficulty of predicting real-time seed longevity using artificial aging. Although a significant correlation has previously been found between artificial aging and real-time data (Probert et al. 2009; P < 0.001), this correlation was not supported by Colville and Pritchard (2019), nor by the present study.
A difficulty in using artificial aging to predict comparative real-time longevity lies in the contrast between the aging environment (high humidity, high temperature) and standard storage conditions (low humidity, low temperature; Walters et al. 2005; Mira et al. 2015; Zinsmeister et al. 2020). The relative ability to survive under these contrasting conditions is likely to be dependent on adaptions to the environment from which the species was collected. For example, the conditions commonly used in artificial aging (60% relative humidity and 45°C) approximate a summer heat-wave event in lowland coastal areas of NSW. Species native to those areas are more likely to have mechanisms to deal with the temperature of the aging environment than species from higher elevations that do not encounter such high temperatures in their natural environment. The relatively poor longevity of high-elevation species under artificial aging (e.g. Satyanti et al. 2018) may therefore be more a reflection of thermal tolerance limits than their likely longevity in storage.
A second difficulty encountered in this study was the relatively short period of time the study species had been in storage. While this was sufficient to detect declining viability in a surprisingly high number of species, it made it difficult to reliably calculate p50RT. In many cases, only two data points were available for estimating p50 from the real-time data, and germination had decreased to 50% for only five species. Walters et al. (2005) found that p50 values obtained by extrapolation beyond available datapoints were less certain than those calculated from data encompassing the target value. These authors also found that p50 (modelled using Avrami parameters) was likely to be overestimated if less than 30 years of data were available and the reduction in germination was less than 5–6%. Values for p50RT will therefore need to be recalculated in a few years’ time for those species showing only a minor reduction in germination (e.g. P. pandorana and S. vicinum).
The lack of change, or increase, in germination exhibited by nine species in this study (Table 1) indicated that viability decline has not yet commenced, and additional re-testing will be required to model their longevity in storage. For the remainder, it is evident from this data set that very short-lived rainforest species do indeed have a low p50 value when tested by artificial aging (as seen here with L. prominens and A. ornans), but a low p50AA does not necessarily mean that a species will be short-lived in storage. The implication of these results is that the viability of species identified as very short-lived by artificial aging (e.g. p50AA ≤ 10) should be monitored closely in real time to enable early detection of decline.
Predictors of seed longevity
In this study, rainforest-restricted species exhibited a significantly lower p50AA than species with a distribution extending to, or limited to, other habitats (such as open forest, woodland and grassland). This result suggested that species from warm, wet habitats are likely to be shorter-lived in storage than those from warm, dry habitats; a finding that contradicts Merritt et al. (2014a) but no doubt reflects the higher number of rainforest-restricted species included in this analysis. The comparatively poor longevity of such species may reflect adaptation to the more stable temperature and soil moisture conditions typical of many rainforest habitats. Under these conditions, survival may be assured by germination and the formation of seedling banks, rather than long-lived soil seed banks (Vázquez-Yanes and Orozco-Segovia 1993), and seeds may therefore lack the repair mechanisms required to remain viable in more extreme habitats.
Plant habit and the presence or absence of endosperm were not associated with longevity for the 33 rainforest species assessed here, although both traits have been found to be significantly associated with longevity previously. Merritt et al. (2014a), for example, found that woody plants produced seeds that were longer-lived than those of herbaceous plants, and both Merritt et al. (2014a) and Probert et al. (2009) found that non-endospermic seeds were longer-lived than endospermic seeds. The lack of relationship found here may be an indication that some correlates of longevity are less applicable in the rainforest environment or may simply be a function of the comparatively small dataset.
Simple linear regression of p50AA for rainforest species against seed weight, geographic and climatic variables showed a significant relationship with only two variables, namely, a positive relationship with average temperature and a negative relationship with elevation. As might be expected, these two variables were highly correlated, with average temperature decreasing as elevation increased. The result suggests that lowland rainforest species are likely to be longer-lived than their montane counterparts. The lack of any influence from precipitation on this dataset is likely to reflect the high annual rainfall in rainforest environments (>1000 mm per annum for all but three species; Table S1).
Regression of p50AA for both rainforest and non-rainforest species against the same set of individual predictors showed that increasing precipitation, proportion of wet days per month, elevation, southerly latitude and longitude had a significant negative influence, whereas increasing diurnal temperature range had a significant positive influence, on seed longevity. The negative influence of precipitation supported previous studies that found species from drier environments were longer-lived than those from wetter environments (Probert et al. 2009; Merritt et al. 2014a). As precipitation increases with longitude (i.e. from west to east) across the sampling region of this study (Bureau of Meteorology 2023), the negative influence of longitude is likely to be associated with rainfall. Similarly, the positive influence of diurnal temperature range, which increases from east to west across the sampling region (Bureau of Meteorology 2023), is likely to be associated with an increasingly dry environment. The negative influence of increasing elevation on longevity in both the rainforest dataset and the larger dataset supported previous studies that found that species from high elevations were shorter-lived than species from low elevations (Satyanti et al. 2018). However, the low R2 value of all individual predictors (≤14%) suggested that multiple factors may be at play.
Subsequent modelling identified both average temperature and elevation as individually significant predictors of longevity among rainforest species, whereas elevation and restriction to rainforest habitats were the only significant predictors for the full dataset of rainforest and non-rainforest species. Given that high-elevation environments (such as alpine and tropical montane habitats) are considered at great risk from climate change (Costion et al. 2015; Cotto et al. 2017), this result suggests the need for greater investment in viability monitoring and re-collecting or regenerating seed collections from high altitudes. Data comparing longevity under artificial aging with real-time longevity are lacking for species from high-altitude environments, so it is possible that their comparatively low p50AA values are more a reflection of intolerance of the aging conditions than poor performance in storage; this possibility will only be confirmed or refuted when sufficient data from real-time monitoring are available for comparison.
Although phylogeny can have an important influence on longevity (Merritt et al. 2014a), a full phylogenetic comparison was not attempted in this study because nearly all of the rainforest genera, and more than half of the families, were not represented in the non-rainforest dataset. Instead, we accounted for any over-representation of families that might skew results by conducting a final analysis limiting the number of species per family to three in each habitat category. For this final dataset, elevation was once again a significant predictor (in this case, the only predictor) of longevity.
Other factors limiting longevity
Longevity is highly variable among species (Walters et al. 2005; Probert et al. 2009; Merritt et al. 2014a), and can also vary among different collections of the same taxon (Crawford et al. 2007; Hay and Whitehouse 2017). This infraspecific variation can be related to the initial quality of the collection (Crawford et al. 2011), conditions in the maternal environment during seed development (Kochanek et al. 2009), seed maturity (Hay and Probert 2013) and post-harvest handling (Probert et al. 2007). Although the level of genetic diversity and the maternal environment are generally not possible to control in the wild, careful attention to seed maturity and post-harvest handling can maximise the longevity of a collection in storage. However, natural variation in the timing of flowering within and among individuals can mean that any collection of seed from wild sources is likely to contain a proportion of immature seeds, which may contribute to variation among populations.
Our intention in this study was to see whether there was an overall pattern in the longevity of rainforest-restricted species compared with rainforest-generalists and non-rainforest species. For this purpose, we believe the use of one p50AA value per species for a broad range of species was appropriate. A similar approach was adopted in previous studies, such as those conducted by Probert et al. (2009) and Merritt et al. (2014a). However, we acknowledge that considerable variation can occur among populations of the same species, and therefore the p50AA values obtained here should be considered a guide rather than an absolute.
Implications for seedbank management
Understanding seed longevity is a critical function of seedbank curation, and artificial aging experiments may be used to customise the frequency of viability tests (Davies et al. 2016; Hay and Whitehouse 2017). Species identified as potentially short-lived can be flagged for additional monitoring and possible re-collection (Chau et al. 2019), or for investigation of alternative storage temperatures that might improve longevity (Mira et al. 2019). Although the relative longevity of rainforest species determined by artificial aging was not well correlated with real-time longevity in this study, nine species with a p50AA of <10 showed a significant loss of viability in less than 12 years in storage. As some species predicted to be long-lived also showed a decline in this time period, we suggest that orthodox rainforest species should be managed as short-lived, with viability checked at least every 5 years, until real-time longevity data indicate otherwise.
Data availability
The data that support this study will be shared upon reasonable request to the corresponding author.
Declaration of funding
This study was funded by past and present supporters of the Rainforest Seed Conservation Project: Arcadia – a charitable fund of Lisbet Rausing and Peter Baldwin; The Maxwell Foundation; The Greatorex Foundation; HSBC Bank; Bloomberg; The Mindaroo Foundation and several private benefactors.
Acknowledgements
We thank staff and students of The Australian PlantBank, Botanic Gardens of Sydney, for data on initial seed viability and assistance with germination testing. In particular, we thank Martina Taylor, University of Sydney Honours student (2016), for conducting aging tests on 13 species. We thank Ricardo Maldonado of Power Stats Pty Ltd for preliminary modelling of p50AA against explanatory variables.
References
Brooks TM, Wright SJ, Sheil D (2009) Evaluating the success of conservation actions in safeguarding tropical forest biodiversity. Conservation Biology 23, 1448–1457.| Evaluating the success of conservation actions in safeguarding tropical forest biodiversity.Crossref | GoogleScholarGoogle Scholar |
Bureau of Meteorology (2023) Maps of average conditions. Australian Government Bureau of Meteorology, Canberra. Available at http://www.bom.gov.au/climate/averages/maps.shtml [Verified 21 March 2023]
Chau MM, Chambers T, Weisenberger L, Keir M, Kroessig TI, Wolkis D, Kam R, Yoshinaga AY (2019) Seed freeze sensitivity and ex situ longevity of 295 species in the native Hawaiian flora. American Journal of Botany 106, 1248–1270.
| Seed freeze sensitivity and ex situ longevity of 295 species in the native Hawaiian flora.Crossref | GoogleScholarGoogle Scholar |
Cochrane JA, Crawford AD, Monks LT (2007) The significance of ex situ seed conservation to reintroduction of threatened plants. Australian Journal of Botany 55, 356–361.
| The significance of ex situ seed conservation to reintroduction of threatened plants.Crossref | GoogleScholarGoogle Scholar |
Colville L, Pritchard HW (2019) Seed life span and food security. New Phytologist 224, 557–562.
| Seed life span and food security.Crossref | GoogleScholarGoogle Scholar |
Commander LE, Coates DJ, Broadhurst L, Offord CA, Makinson RO, Matthes M (2018) ‘Guidelines for the translocation of threatened plants in Australia.’ 3rd edn. (Australian Network for Plant Conservation: Canberra, ACT, Australia). Available at https://www.anpc.asn.au/translocation/ [Verified 31 August 2022]
Costion CM, Simpson L, Pert PL, Carlsen MM, John Kress W, Crayn D (2015) Will tropical mountaintop plant species survive climate change? Identifying key knowledge gaps using species distribution modelling in Australia. Biological Conservation 191, 322–330.
| Will tropical mountaintop plant species survive climate change? Identifying key knowledge gaps using species distribution modelling in Australia.Crossref | GoogleScholarGoogle Scholar |
Cotto O, Wessely J, Georges D, Klonner G, Schmid M, Dullinger S, Thuiller W, Guillaume F (2017) A dynamic eco-evolutionary model predicts slow response of alpine plants to climate warming. Nature Communications 8, 15399
| A dynamic eco-evolutionary model predicts slow response of alpine plants to climate warming.Crossref | GoogleScholarGoogle Scholar |
Crawford AD, Plummer JA, Probert RJ, Steadman KJ (2011) The influence of cone age on the relative longevity of Banksia seeds. Annals of Botany 107, 303–309.
| The influence of cone age on the relative longevity of Banksia seeds.Crossref | GoogleScholarGoogle Scholar |
Crawford AD, Steadman KJ, Plummer JA, Cochrane A, Probert RJ (2007) Analysis of seed-bank data confirms suitability of international seed-storage standards for the Australian flora. Australian Journal of Botany 55, 18–29.
| Analysis of seed-bank data confirms suitability of international seed-storage standards for the Australian flora.Crossref | GoogleScholarGoogle Scholar |
Davies RM, Newton RJ, Hay FR, Probert RJ (2016) 150-seed comparative longevity protocol – a reduced seed number screening method for identifying short-lived seed conservation collections. Seed Science and Technology 44, 569–584.
| 150-seed comparative longevity protocol – a reduced seed number screening method for identifying short-lived seed conservation collections.Crossref | GoogleScholarGoogle Scholar |
Dormann CF, Elith J, Bacher S, Buchmann C, Carl G, Carré G, Marquéz JRG, Gruber B, Lafourcade B, Leitão PJ, Münkemüller T, McClean C, Osborne PE, Reineking B, Schröder B, Skidmore AK, Zurell D, Lautenbach S (2013) Collinearity: a review of methods to deal with it and a simulation study evaluating their performance. Ecography 36, 27–46.
| Collinearity: a review of methods to deal with it and a simulation study evaluating their performance.Crossref | GoogleScholarGoogle Scholar |
Harden GJ, Nicholson HRW, McDonald WJF, Nicholson NJ, Tame T (2016) ‘Rainforest plants of Australia: Rockhampton to Victoria.’ (Gwen Harden Publishing and Terania Rainforest Publishing: NSW, Australia)
Hay FR, Probert RJ (2013) Advances in seed conservation of wild plant species: a review of recent research. Conservation Physiology 1, cot030
| Advances in seed conservation of wild plant species: a review of recent research.Crossref | GoogleScholarGoogle Scholar |
Hay FR, Whitehouse KJ (2017) Rethinking the approach to viability monitoring in seed genebanks. Conservation Physiology 5, cox009
| Rethinking the approach to viability monitoring in seed genebanks.Crossref | GoogleScholarGoogle Scholar |
Hay FR, Merritt DJ, Soanes JA, Dixon KW (2010) Comparative longevity of Australian orchid (Orchidaceae) seeds under experimental and low temperature storage conditions. Botanical Journal of the Linnean Society 164, 26–41.
| Comparative longevity of Australian orchid (Orchidaceae) seeds under experimental and low temperature storage conditions.Crossref | GoogleScholarGoogle Scholar |
Hutchinson MF, Kesteven JL, Xu T, Stein JL, Evans BJ (2015) ANUClimate Version 1.0. Ecosystem Modelling and Scaling Infrastructure (eMAST). [Dataset]. Macquarie Park, NSW, Australia.
Klein J (2003) Postharvest physiology: seed storage. In ‘Encyclopedia of applied plant sciences’. (Ed. B Thomas) pp. 862–865. (Elsevier: Amsterdam, Netherlands)
Kochanek J, Steadman KJ, Probert RJ, Adkins SW (2009) Variation in seed longevity among different populations, species and genera found in collections from wild Australian plants. Australian Journal of Botany 57, 123–131.
| Variation in seed longevity among different populations, species and genera found in collections from wild Australian plants.Crossref | GoogleScholarGoogle Scholar |
Liu U, Breman E, Cossu TA, Kenney S (2018) The conservation value of germplasm stored at the Millennium Seed Bank, Royal Botanic Gardens, Kew, UK. Biodiversity and Conservation 27, 1347–1386.
| The conservation value of germplasm stored at the Millennium Seed Bank, Royal Botanic Gardens, Kew, UK.Crossref | GoogleScholarGoogle Scholar |
Merritt DJ, Martyn AJ, Ainsley P, Young RE, Seed LU, Thorpe M, Hay FR, Commander LE, Shackelford N, Offord CA, Dixon KW, Probert RJ (2014a) A continental-scale study of seed lifespan in experimental storage examining seed, plant, and environmental traits associated with longevity. Biodiversity and Conservation 23, 1081–1104.
| A continental-scale study of seed lifespan in experimental storage examining seed, plant, and environmental traits associated with longevity.Crossref | GoogleScholarGoogle Scholar |
Merritt DJ, Hay FR, Swarts ND, Sommerville KD, Dixon KW (2014b) Ex situ conservation and cryopreservation of orchid germplasm. International Journal of Plant Sciences 175, 46–58.
| Ex situ conservation and cryopreservation of orchid germplasm.Crossref | GoogleScholarGoogle Scholar |
Merritt DJ, Whitehouse KJ, Hoyle GL, Crawford AD, Wood JA, Satyanti A, Norton S, Errington G, Martyn Yenson AJ (2021) Seed banking: orthodox seeds. In ‘Plant germplasm conservation in Australia: strategies and guidelines for developing, managing and utilising ex situ collections’. 3rd edn. (Eds AJ Martyn Yenson, CA Offord, PF Meagher, T Auld, D Bush, DJ Coates, LE Commander, LK Guja, SL Norton, RO Makinson, R Stanley, N Walsh, D Wrigley, L Broadhurst) pp 119–158. (Australian Network for Plant Conservation: Canberra, ACT, Australia) Available at https://www.anpc.asn.au/plant-germplasm/ [Verified 31 August 2022]
Mira S, Estrelles E, Gonzalez-Benito ME (2015) Effect of water content and temperature on seed longevity of seven Brassicaceae species after 5 years of storage. Plant Biology 17, 153–162.
| Effect of water content and temperature on seed longevity of seven Brassicaceae species after 5 years of storage.Crossref | GoogleScholarGoogle Scholar |
Mira S, Nadarajan J, Liu U, Gonzalez-Benito ME, Pritchard HW (2019) Lipid thermal fingerprints of long-term stored seeds of Brassicaceae. Plants 8, 414
| Lipid thermal fingerprints of long-term stored seeds of Brassicaceae.Crossref | GoogleScholarGoogle Scholar |
Mondoni A, Probert RJ, Rossi G, Vegini E, Hay FR (2011) Seeds of alpine plants are short lived: implications for long-term conservation. Annals of Botany 107, 171–179.
| Seeds of alpine plants are short lived: implications for long-term conservation.Crossref | GoogleScholarGoogle Scholar |
Newton R, Hay F, Probert R (2009) ‘Protocol for comparative seed longevity testing.’ (Royal Botanic Gardens, Kew: London, UK) Available at https://brahmsonline.kew.org/Content/Projects/msbp/resources/Training/01-Comparative-longevity.pdf [Verified 31 August 2022]
Offord CA, McKensy ML, Cuneo PV (2004) Critical review of threatened species collections in the New South Wales Seedbank: implications for ex situ conservation of biodiversity. Pacific Conservation Biology 10, 221–236.
| Critical review of threatened species collections in the New South Wales Seedbank: implications for ex situ conservation of biodiversity.Crossref | GoogleScholarGoogle Scholar |
Probert R, Adams J, Coneybeer J, Crawford A, Hay F (2007) Seed quality for conservation is critically affected by pre-storage factors. Australian Journal of Botany 55, 326–335.
| Seed quality for conservation is critically affected by pre-storage factors.Crossref | GoogleScholarGoogle Scholar |
Probert RJ, Daws MI, Hay FR (2009) Ecological correlates of ex situ seed longevity: a comparative study on 195 species. Annals of Botany 104, 57–69.
| Ecological correlates of ex situ seed longevity: a comparative study on 195 species.Crossref | GoogleScholarGoogle Scholar |
R Core Team (2021) ‘R: a language and environment for statistical computing.’ (R Foundation for Statistical Computing: Vienna, Austria). Available at https://www.R-project.org/ [Verified 31 August 2022]
RBGDT (2020) ‘PlantNET: the NSW plant information network system.’ (Royal Botanic Gardens and Domain Trust: Sydney, NSW, Australia). Available at http://plantnet.rbgsyd.nsw.gov.au [Verified 2 September 2022]
Sallon S, Solowey E, Cohen Y, Korchinsky R, Egli M, Woodhatch I, Simchoni O, Kislev M (2008) Germination, genetics, and growth of an ancient date seed. Science 320, 1464
| Germination, genetics, and growth of an ancient date seed.Crossref | GoogleScholarGoogle Scholar |
Satyanti A, Nicotra AB, Merkling T, Guja LK (2018) Seed mass and elevation explain variation in seed longevity of Australian alpine species. Seed Science Research 28, 319–331.
| Seed mass and elevation explain variation in seed longevity of Australian alpine species.Crossref | GoogleScholarGoogle Scholar |
Shen-Miller J, Mudgett MB, Schopf JW, Clarke S, Berger R (1995) Exceptional seed longevity and robust growth: ancient Sacred Lotus from China. American Journal of Botany 82, 1367–1380.
| Exceptional seed longevity and robust growth: ancient Sacred Lotus from China.Crossref | GoogleScholarGoogle Scholar |
Sommerville KD, Clarke B, Keppel G, McGill C, Newby Z-J, Wyse SV, James SA, Offord CA (2017) Saving rainforests in the South Pacific: challenges in ex situ conservation. Australian Journal of Botany 65, 609–624.
| Saving rainforests in the South Pacific: challenges in ex situ conservation.Crossref | GoogleScholarGoogle Scholar |
Sommerville KD, Errington G, Newby Z-J, Liyanage GS, Offord CA (2021) Assessing the storage potential of Australian rainforest seeds: a decision-making key to aid rapid conservation. Biodiversity and Conservation 30, 3185–3218.
| Assessing the storage potential of Australian rainforest seeds: a decision-making key to aid rapid conservation.Crossref | GoogleScholarGoogle Scholar |
Tuckett RE, Merritt DJ, Hay FR, Hopper SD, Dixon KW (2010) Comparative longevity and low-temperature storage of seeds of Hydatellaceae and temporary pool species of south-west Australia. Australian Journal of Botany 58, 327–334.
| Comparative longevity and low-temperature storage of seeds of Hydatellaceae and temporary pool species of south-west Australia.Crossref | GoogleScholarGoogle Scholar |
Tweddle JC, Dickie JB, Baskin CC, Baskin JM (2003) Ecological aspects of seed desiccation sensitivity. Journal of Ecology 91, 294–304.
| Ecological aspects of seed desiccation sensitivity.Crossref | GoogleScholarGoogle Scholar |
Vázquez-Yanes C, Orozco-Segovia A (1993) Patterns of seed longevity and germination in the tropical rainforest. Annual Review of Ecology and Systematics 24, 69–87.
| Patterns of seed longevity and germination in the tropical rainforest.Crossref | GoogleScholarGoogle Scholar |
Walters C, Pence VC (2020) The unique role of seed banking and cryobiotechnologies in plant conservation. Plants, People, Planet 3, 83–91.
| The unique role of seed banking and cryobiotechnologies in plant conservation.Crossref | GoogleScholarGoogle Scholar |
Walters C, Wheeler LM, Grotenhuis JM (2005) Longevity of seeds stored in a genebank: species characteristics. Seed Science Research 15, 1–20.
| Longevity of seeds stored in a genebank: species characteristics.Crossref | GoogleScholarGoogle Scholar |
Wyse SV, Dickie JB (2017) Predicting the global incidence of seed desiccation sensitivity. Journal of Ecology 105, 1082–1093.
| Predicting the global incidence of seed desiccation sensitivity.Crossref | GoogleScholarGoogle Scholar |
Zich FA, Hyland BPM, Whiffin T, Kerrigan RA (2018) ‘Australian tropical rainforest plants.’ 8th edn. Available at https://apps.lucidcentral.org/rainforest/text/intro/index.html [Verified 2 September 2020]
Zinsmeister J, Leprince O, Buitink J (2020) Molecular and environmental factors regulating seed longevity. Biochemical Journal 477, 305–323.
| Molecular and environmental factors regulating seed longevity.Crossref | GoogleScholarGoogle Scholar |