Australian salt basins – options for underground hydrogen storage
Marita Bradshaw A , Stephanie Rees A , Liuqi Wang A , Mike Szczepaniak A , Wayne Cook A , Sam Voegeli B , Christopher Boreham A , Carmine Wainman A , Sebastian Wong A , Chris Southby A and Andrew Feitz A *A Geoscience Australia, GPO Box 378, Canberra, ACT 2601, Australia.
B RESPEC, Rapid City, SD, USA.
The APPEA Journal 63 285-304 https://doi.org/10.1071/AJ22153
Submitted: 23 December 2022 Accepted: 3 March 2023 Published: 11 May 2023
© 2023 The Author(s) (or their employer(s)). Published by CSIRO Publishing on behalf of APPEA. This is an open access article distributed under the Creative Commons Attribution 4.0 International License (CC BY).
Abstract
As Australia and the world transition to net zero emissions, hydrogen will continue to grow in importance as a clean energy source, with underground hydrogen storage (UHS) expected to be a key component of this new industry. Salt (halite) caverns are a preferred storage option for hydrogen, given their scale, stability and the high injection and withdrawal rates they can support. The use of salt caverns for storing gas is an established industry in North America and Europe but not in Australia, where exploration for suitable storage locations is in the initial frontier stages. Australia’s known major halite deposits occur in Neoproterozoic and Paleozoic sequences and are predominantly located in western and central Australia. This analysis has identified potential in eastern Australia in addition to the proven thick halite in the Adavale Basin, Queensland. Building on Geoscience Australia’s previous salt studies in the Canning, Polda and Adavale basins, this study expands the portfolio of areas prospective for halite in onshore and offshore basins using both direct and indirect evidence. The study correlates paleogeography and paleoclimate reconstructions with evidence of salt in wells, and in geophysical and geochemical data. Salt cavern design for UHS, the solution mining process, and the preferred salt deposits are also discussed. The results will provide pre-competitive information through a comprehensive inventory of areas that may be prospective for UHS.
Keywords: Adavale Basin, Amadeus Basin, evaporites, halite, Officer Basin, Polda Basin, salt caverns, underground hydrogen storage, UHS.
Introduction
Hydrogen provides flexible options for transporting and storing energy that will enable deep penetration of renewables into the energy mix. It is a clean energy source especially suited for uses that are hard to electrify and/or abate, such as powering heavy vehicles and the manufacturing of iron and steel (BloombergNEF 2020). Australia’s energy security will be enhanced by a domestic hydrogen industry that will provide a more diverse and resilient energy supply and less reliance on fuel imports (DCCEEW 2022). Underground hydrogen storage (UHS) is an enabling technology that provides a flexible, short- to long-term energy storage option to complement short- to medium-term energy storage, such as batteries and pumped hydroelectricity schemes for grid stabilisation (Michael et al. 2021).
Hydrogen and salt are inextricably linked. Presently, all large-scale storage of commercially produced hydrogen (from unabated fossil fuels) occurs in underground salt (halite) caverns. Salt caverns are typically formed by solution mining cavities in salt rock. This process has been used to develop caverns for liquid-petroleum gas storage in Texas since the 1950s (Gill and Cowan 2008), and it is estimated that there are presently 104 salt caverns (as of 2017) used worldwide for energy storage (Cornot-Gandolphe 2019). For hydrogen, salt caverns provide the advantages of high gas deliverability, low cushion gas requirements and a high sealing capacity, making salt caverns competitive with depleted gas fields, surface tanks and hard rock caverns for large-scale UHS (BloombergNEF 2019). Globally, there are four salt caverns for hydrogen storage currently in operation (Hévin 2019; Caglayan et al. 2020; Ennis-King et al. 2021), with more caverns planned given the increasing global demand for hydrogen with low carbon emissions (International Energy Agency 2022). For example, the Advanced Clean Energy Storage Delta project in Utah (ACES DELTA 2022) plans to store green hydrogen in two large salt caverns, each capable of storing 150 GWh of energy (~5500 tonnes of hydrogen per cavern). The UK, Germany, Ireland and the Netherlands are pursuing UHS in both onshore and offshore salt accumulations (Caglayan et al. 2020; Chedwynd 2021; dCarbonX 2021; ESB 2021; RVO 2022).
In addition to providing sealing capacity for hydrogen storage, salt may act as a trap for natural (geologic) hydrogen sourced from deep subsurface abiotic processes (Haines and Allen 2020; Boreham et al. 2021). The discovery of a hydrogen gas field in Mali (Prinzhofer et al. 2018) has spurred exploration for this potentially new carbon-free source of energy, with Australia considered one of the most prospective locations (Boreham et al. 2021; Moretti et al. 2021). Free hydrogen and hydrogen in fluid inclusions within salt deposits have been attributed to it being effectively trapped by alternating layers of clays and salt and the inertness of halite to the highly reactive hydrogen. Additionally, the radioactive decay of potassium (present in potash) and its further radioactive decay products (40Ca from 40K) can reduce water to hydrogen (Zgonnik 2020) given suitable porosity and permeability within the salt, which can occur in deep sedimentary basins (Scribano et al. 2017). These associations suggest the possibility that the components of hydrogen plays (Stalker et al. 2022) may incorporate salt as source, reservoir and seal.
Salt caverns for UHS
When developing underground salt caverns for storage purposes, careful consideration to the target salt formation is required to ensure successful hydraulic containment and geomechanical behaviour. Ideally, the salt formation will consist of thick (>200 m) and pure (>90%) halite with minimal non-salt inclusions (e.g. interbedded anhydrite). The salt formation depth often controls the operating pressure envelope for gas (including hydrogen) storage, where deeper caverns can withstand higher storage pressures and higher storage densities than shallower caverns. In general, gas storage caverns target an optimal depth range of approximately 900–1500 m, which maximises the storage capacity while also avoiding problematic geomechanical conditions at deeper depths. Although there can be some site-specific flexibility, gas storage cavern fields are typically recommended to maintain a spacing to diameter ratio of at least four, where the spacing refers to the cavern centre-to-centre distance and the diameter is the average diameter of the two neighbouring caverns.
The solution mining of storage caverns requires both a water source (ideally fresh or brackish) and a disposal method for the produced brine. As a rule of thumb, a freshwater volume to cavern volume ratio of approximately seven will be required to solution mine a cavern. As an example, if the target storage cavern volume is 1 million m3, solution mining will require a total of 7 million m3 of freshwater. Consequently, 7 million m3 of brine will also be produced, which will need to be managed either through production of a commercial salt product (and recovery of water from the brine for the solution mining process) or through deep disposal injection or other methods (e.g. solar evaporation). Depending on the target development timelines, water source and disposal rates often exceeding 200 m3/h are required, which necessitates a robust water and disposal infrastructure. The typical time required to solution mine a storage cavern often ranges between 6 months and 3 years, depending on the target cavern volume and water injection rates. In the situation where a gas storage cavern gradually loses volume over time due to salt creep, it is often necessary to re-solution mine the cavern to regain the lost volume. Depending on the volumetric goals, these re-solution mining activities typically require a few months or up to a year to complete.
The deformation behaviour of salt is typically dominated by a viscoplastic (i.e. creep) mechanism that increases exponentially with stress and temperature. Therefore, the higher stress and temperature conditions in deeper salt formations will result in enhanced salt creep, which manifests as a more mobile salt that gradually ‘flows’ inward towards the cavern. This enhanced salt creep decreases the volume of the storage cavern, essentially returning the salt to a pre-cavern-development state. Although not a strict limit, storage caverns are typically limited to a maximum depth of approximately 2000 m. Storage cavern development and operation below this depth are complicated by significant salt creep that can exceed a volume loss rate of 20%/year, which is often not economical for storage operations. Also important is to consider and manage post-injection processes, including increased moisture content in the stored hydrogen with increasing confinement pressure (Lopez-Lazaro et al. 2019) and microbial utilisation of hydrogen with the addition of other gaseous species, such as methane (Schienteie et al. 2022).
Although it is well-established in Europe and North America, there is no gas storage in Australia that utilises salt caverns. Natural gas storage is limited to depleted reservoirs from eight previously producing gas fields and above ground LNG tank facilities.
A sustained exploration phase supported by pre-competitive geoscience data is required to find thick halite in useful locations in Australia. This paper aims to sketch out the search space for UHS in salt, show how our continent-wide geophysical and other datasets can be leveraged to high-grade areas in case studies at prospect, play and frontier reconnaissance levels of understanding.
Evaporite depositional environments
Evaporites are chemical sediments precipitated from concentrating brines in the familiar environments of salt lakes and coastal tidal flats (sabhkas) in warm, dry climates (Warren 2010). Evaporation of seawater yields, with increasing salinity, the marine evaporite sequence of carbonates, gypsum, halite and late-stage bitten salts (K and Mg salts, potash). Marine evaporites fall into two main types: (1) gypsum/anhydrite dominated platform sequences with no or only thin (>10 m) halite and (2) thick, basin-centred halite deposits, termed ‘mega-halites’ or saline giants (Warren 2010, 2021).
These marine, basin-wide deposits are ideal UHS targets with relatively pure halite beds from hundreds of metres to kilometres thick that can, with later halotectonics process, ductile flow into salt pillows, domes and diapirs, producing suitable geometries for constructing salt caverns. Examples of ancient saline giants that are used for gas storage and that are being developed for UHS include the Permian Rotliegend and Zechstein salts, North Sea, and the onshore Europe and Triassic Northwich Halite, Cheshire Basin, UK (Caglayan et al. 2020). In North America, the saline giants used for gas storage include the Silurian Salina Group, Michigan Basin; the Carboniferous Paradox Formation, Paradox Basin in Colorado–Utah, USA; the Permian Salado Salt, Midland Basin; and the Jurassic Louann Salt, Gulf of Mexico Basin (Bruno 2005; Warren 2021). The Cretaceous Maha Sarakham Formation, Khorat Basin, Thailand, is another basin-wide salt being considered for UHS projects (Pajonpai et al. 2022).
Today some of the thickest salts are accumulating in terrestrial environments in high altitude deserts, such as the Altiplano and Tibetan Plateau (Warren 2021), but with their mixed lithologies, they may be less suitable for UHS. There are no Quaternary analogues of ancient marine saline giants, although they are well represented in the past when thick bedded halite deposits accumulated in hydrographically isolated depressions well below sea-level that still had access to seawater in hot, dry and windy climates. A tectonic driver was needed to preserve kilometres of salt in an actively subsiding depression, such as a rift, foreland depression or intraplate sag (Warren 2021).
However, an alternative to the surface evaporative model has been proposed by Scribano et al. (2017), Hovland et al. (2018a, 2018b) and Debure et al. (2019), where salt deposits are derived from hydrothermal fluids associated with Wilson cycles. In the 1970s, new information from drilling the Messinian (Late Miocene) salt underlying the Mediterranean supported shallow water deposition of thick salts in deep desiccated basins as an alternative to deep water brine basin models (Hsu 1972). Similarly, the new information from drilling the sub-salt of offshore Brazil has provided new insights into the relationships between evaporite formation and volcanic activity (Szatmari et al. 2021). The hydrothermal model (Hovland et al. 2018b) allows for deep water halite environments (e.g. Dead Sea) and can help explain the common association of giant salt deposits and new ocean basins; perhaps saline giants form in different and hybrid ways beyond the current end-member models.
Australian salt basins
Building on the work of Boreham et al. (2021), this study attempts to identify all Australian basins that may be prospective for UHS in salt (Fig. 1). Included are basins with direct evidence of halite (well intersection) plus basins where there is only indirect structural or geophysical evidence of salt. Facies commonly associated with halite deposits (carbonates, especially dolomite, other evaporites and red beds) point to other prospective areas for UHS or at least where evaporite seals for natural hydrogen accumulations may occur.
Australian salt basin modified from Boreham et al. (2021). Grey indicates basins with either minor or limited known occurrences of evaporites. The Bremer Sub-basin is suspected of having Precambrian halite based on structures imaged in seismic data. Note that only the onshore extent of Canning Basin is shown.
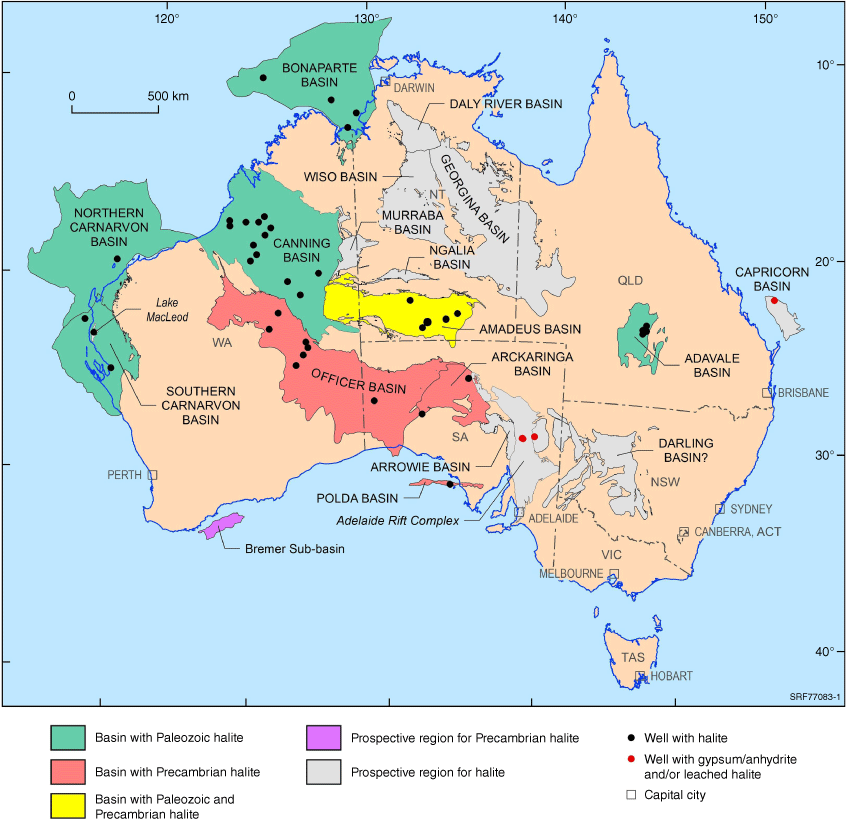
The inventory of Australian evaporites compiled by Wells (1980) highlighted potash resources for agriculture, and recently, lithium for batteries and other strategic resources in salt lakes has been the focus (Mernagh et al. 2013). Now, identifying thick halite deposits for UHS has become a new priority. The Tonian, Early Paleozoic and Devonian were peak times for evaporite deposition in Australia (Table 1). There are some indications of evaporitic facies in Mesoproterozoic and Palaeoproterozoic sequences, such as dolostones in the McArthur and Nathan groups of the greater McArthur Basin (Hall et al. 2020), but no thick halite has been reported. Fig. 2 shows some of Geoscience Australia’s collection of salt samples from cores and cuttings from petroleum wells.
Australian salt basins | |||||
Age | Basin | Salt unit | Evidence of salt (core, cuttings, well logs, seismic) | Facies | Reference |
Quaternary | Lake MacLeod | MacLeod Halite | Shallow wells, costeans | Marine platform | Logan (1987) |
Paleogene | Capricorn | None identified | Well (anhydrite at Aquarius 1) | Non-marine | Hill (1992) |
Early Cretaceous | Fairway – Lord Howe Rise | None identified | Seismic diapir | Marine? | Van de Beuque et al. (2003) |
Late Devonian | Darling | None identified | Restricted marine, red beds | Marine? | Alder et al. (1998) |
Givetian (mid Devonian) | Adavale | Boree Salt | Wells (Boree 1, Bury 1, etc.), core, seismic | Saline giant | Paterson et al. (2022) |
Silurian–Devonian | Bonaparte – Petrel Sub-basin | Silurian–Devonian salt | Wells (Kinmore 1, Pelican 1, Sandpiper 1, etc.), seismic | Saline giant? | Mory (1991) |
Pre-Permian | Bonaparte –Vulcan Sub-basin | Pre-Permian salt | Well (Paqualin 1), seismic | Saline giant? | Molyneux and Doyle (2021) |
Late Ordovician – Silurian | Carnarvon | Yaringa Salt | Wells (halite at Yaringa 1, anhydrite at Pendock 1, etc.) | Saline giant or marine platform? | Canadian superior oil (1976), Continental oil company of Australia (1966) |
Canning – Kidson Sub-basin | Mallowa Salt | Wells (Kidson 1, Fruitcake 1, etc.), seismic | Saline giant | Connors et al. (2022) | |
Late Ordovician | Canning – Kidson Sub-basin | Minjoo Salt | Wells (Kidson 1, Patience 2, Brooke 1, etc.), seismic, AEM | Saline giant | Connors et al. (2022) |
Cambrian | Darling –Bancannia Trough | None identified | Well (carbonates at Bancannia South 1) | Marine platform? | Khalifa and Mills (2022) |
Arrowie | None identified | Well (<10 m anhydrite at Moorowie 1) | Marine platform | Delhi Petroleum (1984) | |
Amadeus | Chandler Salt | Wells (Mt Charlotte 1, Magee 1, etc.), seismic | Saline giant | Bradshaw (1991) | |
Officer | Ouldburra Fm | Wells (<10 m halite in Manya 6, etc.) | Marine platform | Kovalevych et al. (2006) | |
Georgina | None identified | Dolostones | Marine platform | Dunster et al. (2007) | |
Tonian | Amadeus | Bitter Springs – Gillen Member | Wells (Magee 1, Mt Kitty 1, Magee 1 etc.), seismic | Saline giant | Plummer (2021) |
Murraba | None identified | Carbonates, salt structures? | Marine platform? | Haines and Allen (2017) | |
Adelaide Rift Complex | Callana Subgroup | Surface diapirs | Saline giant | Kernen et al. (2021) | |
Below Arckaringa | Callana Subgroup | Wells (Cootanoorina 1, etc.), seismic | Saline giant | Boult et al. (2012) | |
Eastern Officer | Alinya Salt | Well (Mulyawara 1), seismic | Saline giant | Rodinia Oil (2011) | |
Western Officer | Browne Fm | Wells (Browne 1, etc.), diapirs on seismic and AEM | Saline giant | Carr et al. (2012) | |
Polda | Kilroo Fm | Well (Mercury 1), seismic | Saline giant | Nelson et al. (1986) | |
Bremer | Seismic diapirs | Saline giant? | Bradshaw et al. (2013) | ||
Mesoproterozoic–Paleoproterozoic | Greater McArthur | None identified | Dolostones | Marine platform? | Hall et al. (2020) |
Photographs of salt core and cutting samples stored at Geoscience Australia, showing (a) Ordovician Mallowa Salt, Kidson 1, Canning Basin; (b) Neoproterozoic Kilroo Formation, cuttings from Mercury 1, Polda Basin; (c) Cambrian Chandler Formation and (d) Neoproterozoic Gillen Formation, Mt Charlotte 1, Amadeus Basin; and (e) Middle Devonian Boree Salt, Boree 1, Adavale Basin.
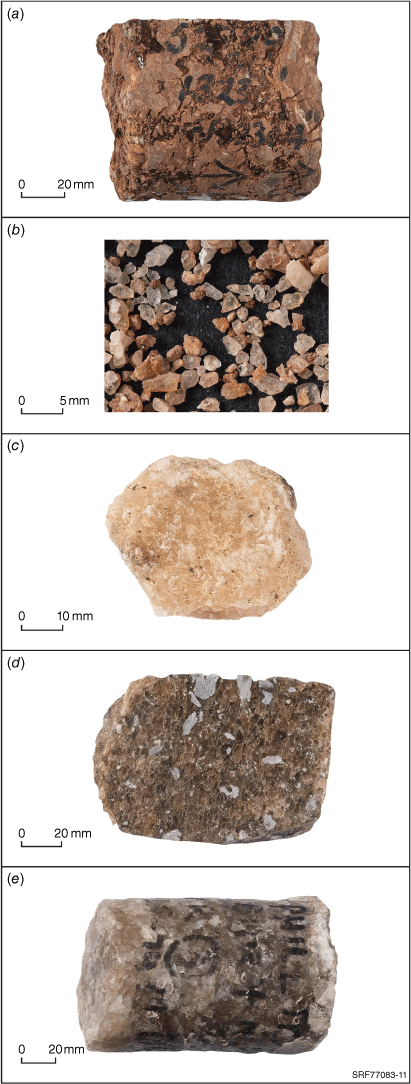
Tonian evaporites
Salt deposition was most extensive across Australia in the Tonian (~1000–720 Ma) when other saline giants were deposited across Rodinia (Fig. 3; Schmid 2017). The Gillen Formation (Amadeus Basin, Fig. 2d), the Browne Formation (Officer Basin) and the Kilroo Formation (Polda Basin, Fig. 2b) are all proven thick halite deposits of Tonian age that are discussed in more detail below. The possible salt in the Bremer Sub-basin (Bradshaw et al. 2013; Cunneen et al. 2019) is also interpreted to be part of this major episode of Neoproterozoic evaporite deposition (Fig. 3). The Amadeus and Officer basins are part of the Centralian Superbasin, (Walter et al. 1995) along with the Georgina, Ngalia and Murraba basins (Fig. 3). The known thick Tonian salts (Gillen and Browne formations) are a minor part of the Centralian 1 Supersequence that fills rifts and sags associated with the break-up of the Rodinia supercontinent (Bradshaw et al. 2020).
Neoproterozoic basins and the Centralian Superbasin modified from Haines and Allen (2017) shown in (a). The Bremer Sub-basin pre-rift strata (interpreted to contain evaporites) is suspected to be equivalent to the Polda Basin or Officer Basin (Bradshaw et al. 2013). Insert (b) is distribution of known Tonian evaporite basins and their likely position at 780 Ma (Li et al. 2008, as cited in Schmid 2017).
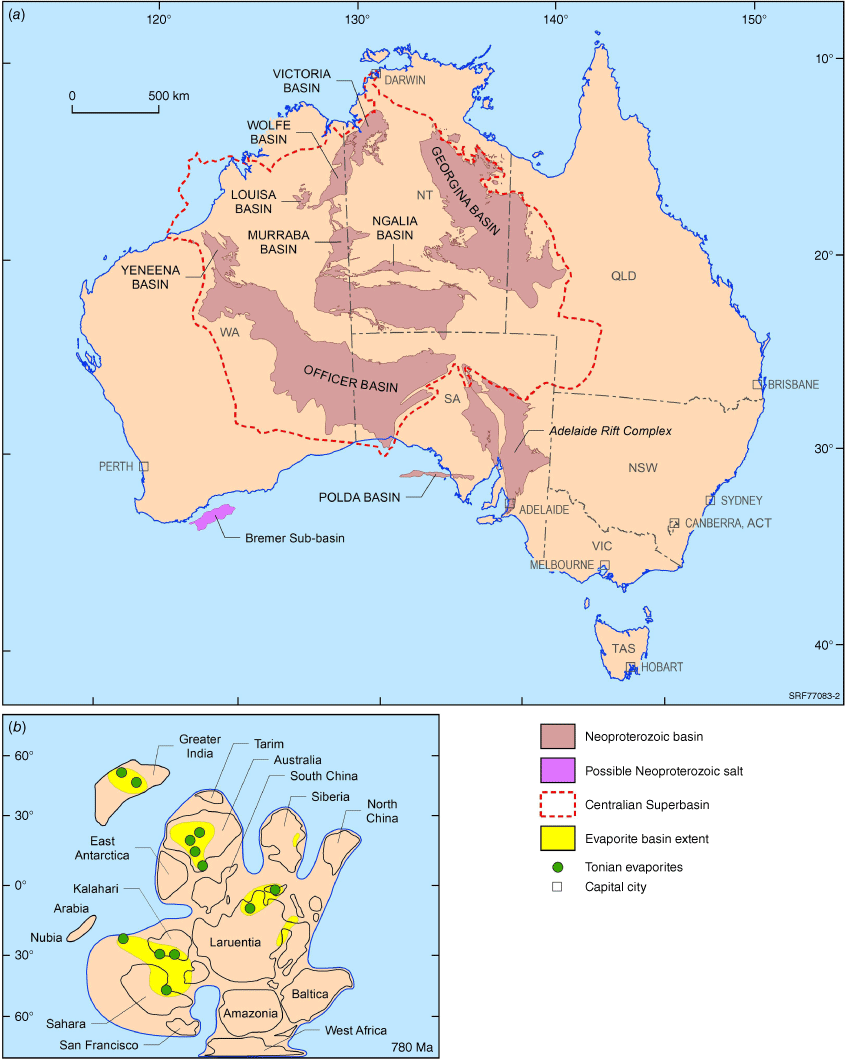
The Adelaide Rift Complex was initiated during this time and was once contiguous with the Centralian Superbasin (Preiss 2000). Numerous diapiric structures (Kernen et al. 2021) and modelling by Backé et al. (2010) indicate that a relatively thick and extensive halite was once within the Tonian section of the rift. The question remains as to whether any significant salt remains in the subsurface given a post-depositional history of tectonism and dissolution; however, Mernagh et al. (2013) suggest that evaporitic units (Callana Group) could be the source of anomalous hydrogeo-chemistry around Lake Torrens today.
The salt features interpreted on seismic data underlying the Arckaringa Basin (eastern Officer Basin extending to the west of the Adelaide Rift Complex) may also be Tonian in age. Halite has been intersected in Cootanoorina 1 and Wilkinson 1 (Kovalevych et al. 2006). Mulyawara 1, also drilled in the eastern Officer Basin (Rodinia Oil 2011; Boult et al. 2012), intersects two Neoproterozoic halite units. The Murraba Basin (Fig. 3) also has a Tonian section as well as having a structural style that is suggestive of salt tectonics in some local areas (Haines and Allen 2017).
Paleozoic evaporites
Sporadic salt deposition occurred during the Cambro–Ordovician when platform carbonates were a common lithology and some evaporitic facies developed, such as dolomites (Red Hart Dolomite, Georgina Basin; Kalladeina Formation, Warburton Basin). Thin halite beds occur in the Early Cambrian Ouldburra Formation, Officer Basin, (Kovalevych et al. 2006) and anhydrite is reported in the early Cambrian of the Arrowie Basin (Moorowie 1, see Table 1). The carbonate facies in Arrowie Basin extends further east into Bancannia Trough underlying the Darling Basin (Khalifa and Mills 2022).
True saline giants occur in the Amadeus (Cambrian Chandler Salt, Fig. 2c) and Canning (Late Ordovician Minjoo Salt and Late Ordovician–Silurian Mallowa Salt: Table 1, Fig. 2a) basins. Structural control was the key factor in creating the conditions to accumulate and preserve these thick salts, with the Lasseter Shear Zone forming a potential structural barrier restricting circulation between the Canning and Amadeus basins (Bradshaw et al. 2020). In the Southern Carnarvon Basin, there are evaporitic facies in the Late Ordovician–Silurian Dirk Hartog Formation, including the Yaringa Salt, which has been penetrated in wells and is up to 37 m thick (Table 1). These occurrences may point to other thick salt bodies, perhaps on the poorly explored Bernier Platform.
By the Devonian, marine conditions had retreated to the western and eastern margins of the continent, though tropical climates still held sway as indicated by the reefal carbonates found in both the Canning and Adavale basins. The Givetian Boree Salt in the Adavale Basin is thick halite that may be suitable for UHS and is discussed further in a case study. On trend to the south are Late Devonian red beds in the Darling Basin (Wilga Downs Group), where palaeographic interpretations of tenuous marine connections (Alder et al. 1998; Young and Lu 2020) suggest some potential for evaporitic facies to be present.
Thick salt occurs in the offshore Bonaparte Basin, where salt structures have been drilled in the Petrel (Sandpiper 1, Pelican Is 1, Kinmore 1) and Vulcan (Paqualin 1) sub-basins (Molyneux and Doyle 2021). Investigating the Canning Basin, McNee et al. (2021) present seismic data to indicate that the salt diapir drilled by Frome Rocks 1 may be Devonian in age and point to a third thick salt interval in the basin.
Mesozoic and Cenozoic evaporites
For much of the Late Palaeozoic and Mesozoic, Australia was near the South Pole and mostly beyond the mid-latitude arid belt. Clastic sediments deposited in fluvial, deltaic, glacial and marine environments dominate, carbonates are rare, and salt is absent (Boucot et al. 2013). However, diapiric features have been noted in seismic data collected on the Lord Howe Rise, in a section interpreted as possibly Early Cretaceous in age (Van de Beuque et al. 2003), and a thin anhydrite bed was intersected in Aquarius 1, Capricorn Basin (Fig. 1, Table 1), in a section considered to be Paleogene in age (Struckmeyer et al. 1994). One of the largest modern examples of a platform marine evaporite is Lake MacLeod, a coastal playa in Western Australia underlain by a graben where a 3–5 m thick Early Holocene bedded halite is overlain by an extensive (2–6 m thick, ~2000 km2) gypsum deposit (Logan 1987; Mernagh et al. 2013).
Recognising salt in the subsurface
The review of Australian salt basins above shows that there are specific time periods and basin settings where evaporite deposition is favoured. However, the uncertainty surrounding the modes of formation of thick halite deposits increases the importance of empirical evidence rather than conceptual models to find salt in the subsurface.
Geophysical evidence
Geophysical methods such as airborne electromagnetic (AEM), seismic, gravity and magnetics can be used as indirect evidence of salt. The AEM method detects electrical conductivity variations (from rock and pore fluid) in the subsurface (Wilford 2022), whereas seismic reflection data measures acoustic impedance contrast (density and velocity) (Bain et al. 1991). Gravity and magnetic data estimate lateral changes in density and magnetic mineral content respectively (Bain et al. 1991).
Geoscience Australia’s recent study in the Canning Basin shows how AEM data can be used to image localised folding/faulting of a shallow conductive unit that may have formed above isolated salt domes (Connors et al. 2022). Although the Canning Basin salt units are deeper than the AEM depth of investigation (salt identified at depth >500 m), distinct zones of disruption within the shallow conductive unit from salt dissolution and mobilisation were observed along the margins of the present-day salt occurrence (Connors et al. 2022).
Although seismic data has been successfully used to image salt in the Gulf of Mexico (Winker 1996), the Norwegian North Sea (Jones and Davison 2014), the western and central United Arab Emirates (Stewart 2018) and the Canning Basin in Australia (Zhan 2019), there are some cases when seismic data fails to image salt. Reasons for this include poor seismic coverage, which is inadequate to capture salt geometry that may change rapidly in space; only a slight acoustic impedance contrast at the salt-sediment interface; and complex salt structures that dip steeply or are surrounded by faults (Jackson and Hudec 2017). In these cases, gravity gradiometry and high-resolution magnetic data can be used to improve salt imaging (Stadtler et al. 2010; Debacker et al. 2016).
Other subsurface features, such as mud diapirs, granitic intrusions or gas chimneys, can also be mistaken for salt diapirs. Gas chimneys are seen as vertically disturbed zones with a chaotic reflection pattern inside where the seismic reflections are discontinuous and its amplitudes are weaker (Løseth et al. 2009; Virs 2015; Singh et al. 2016) (Fig. 4). One major characteristic of gas chimneys that differs from salt is that they are often associated with low-velocity anomalies in seismic profile from the presence of free gas in the sediment, which is identified by the velocity ‘push down’ feature observed above the chimneys (Virs 2015). The main factor distinguishing igneous bodies from salt is the geometry of sediments around the two features. Salt structures are associated with syn-depositional growth into the structure (e.g. mini basins/rim synclines) or collapse structures (e.g. half turtle structures) where accommodation space is being created while the structure forms (Mauduit et al. 1997; Banham and Mountney 2013; Duffy et al. 2017) (Fig. 4). In contrast, igneous intrusions have no associated growth or collapse of adjacent sediments. Although mud diapirs can have similar shape to salt diapirs, they usually have no impedance contrast (from lithology) to their surrounding sediment (Jackson and Hudec 2017). Where a mud diapir is over-pressured, the interface may have a negative reflection coefficient and is commonly gradational, unlike the top salt structures that usually have strong reflection (Jackson and Hudec 2017).
Seismic signatures of various subsurface events that may be mistaken as salt. (a) Gas chimneys seismic signature with push-down feature and chaotic reflection pattern inside the chimneys modified from Virs (2015). (b) Vertical seismic section showing mud/shale diapir (non-reflective) and salt diapirs (strong reflection at the top of salt) modified from Jackson and Hudec (2017). (c) Vertical seismic section showing volcanic intrusion (yellow) modified from Magee et al. (2013). (d) Interpreted seismic cross-section of salt and associated minibasins feature in southern Precaspian Basin from Duffy et al. 2017). VE, vertical exaggeration.
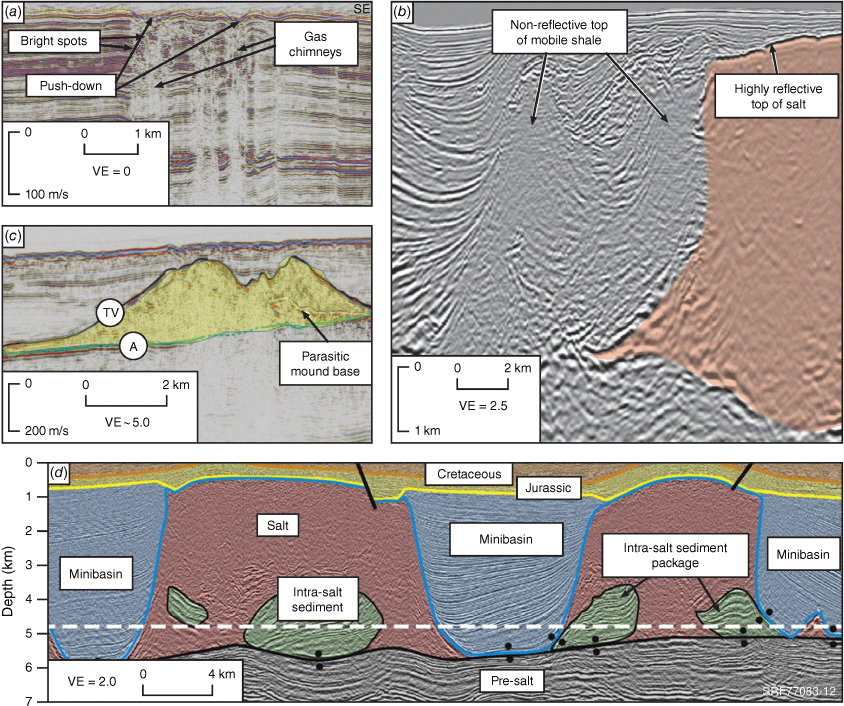
Case studies
The case studies below show how geophysical evidence combined with well data and other regional geological information can be used to develop prospects and plays for UHS.
Polda Basin – UHS prospect in a proven thick salt in an offshore basin
Hydrogen storage in offshore basins salt caverns has received little attention in Australia but is under active consideration in the Netherlands (Innovation Origins 2022), and Ireland is developing three offshore UHS projects (Chedwynd 2021; dCarbonX 2021; ESB 2021). In Australia, the Polda Basin has the potential for UHS in the Neoproterozoic Kilroo Formation. The intracratonic Polda Basin extends 350 km from the Eyre Peninsula to the centre of the Great Australian Bight and contains Proterozoic–Jurassic sedimentary fill (>5000 m), including halite of the Kilroo Formation (Fig. 5) (South Australia Department for Energy and Mining 2021). The Kilroo Formation was deposited in an arid terrestrial fluvial and endorheic playa lakes environment (South Australia Department for Energy and Mining 2021) and is another Tonian saline giant roughly coeval with those in the Amadeus and Officer basins. Salt interpreted from seismic data in the undrilled Bremer Sub-basin (west of the Polda Basin) is also proposed to be part of the Tonian evaporite deposition (Bradshaw et al. 2013; Cunneen et al. 2019) (Fig. 6). The salt was first identified on seismic data pre-drill (Nelson et al. 1986). Three wells drilled down to the Kilroo Formation (Columbia 1, Mercury 1 and Kilroo 1A), but only Mercury 1 penetrated the halite body with a net halite thickness of 1000 m (Feitz et al. 2022).
(a) and (b) are the Polda Basin cross‐section from west to east and wells locations (South Australia Department for Energy and Mining 2021). (c) Map showing the Polda Basin location and the cross‐section A‐B‐C line shown in (a) and (b). Mercury 1 is located offshore, approximately 60 km from the Eyre Peninsula.
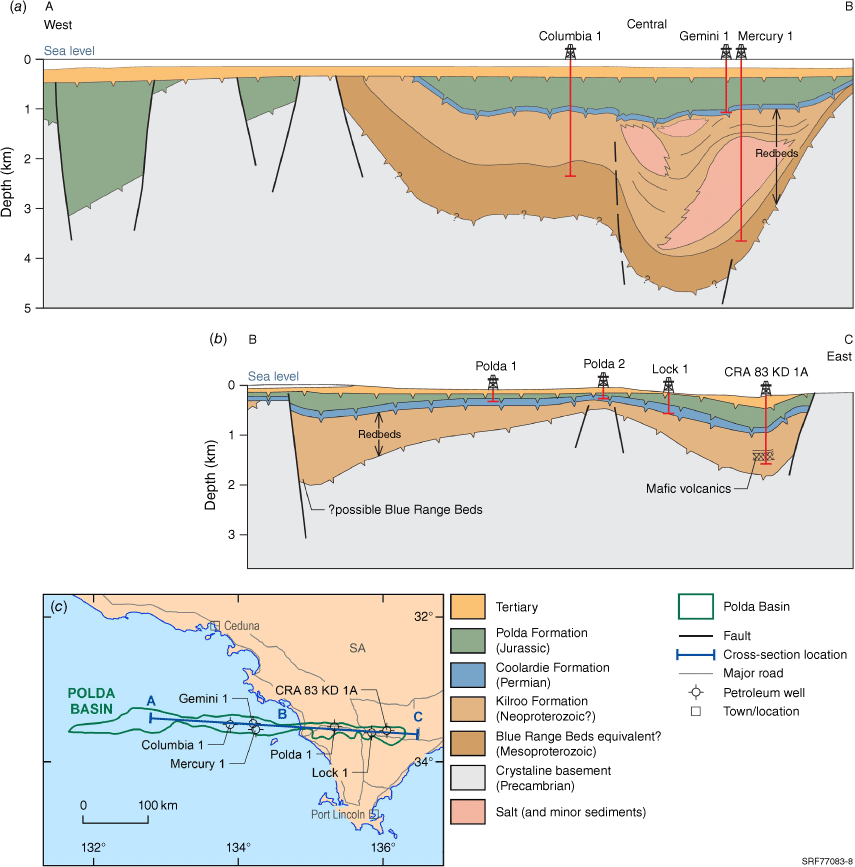
(a) and (b) are salt‐lead features on 2D seismic cross‐sections identified in deep‐water (>2.2 km depth) in the central Bremer Sub‐basin. These salt‐lead structures are suspected to be older than the six Mesozoic seismic stratigraphic units (Bremer 1–6) identified by Bradshaw (2005). (c) Shows 2009 Arcadia 2D seismic survey over the Bremer Sub‐basin in offshore Western Australia. TWT (s), two-way time in seconds.
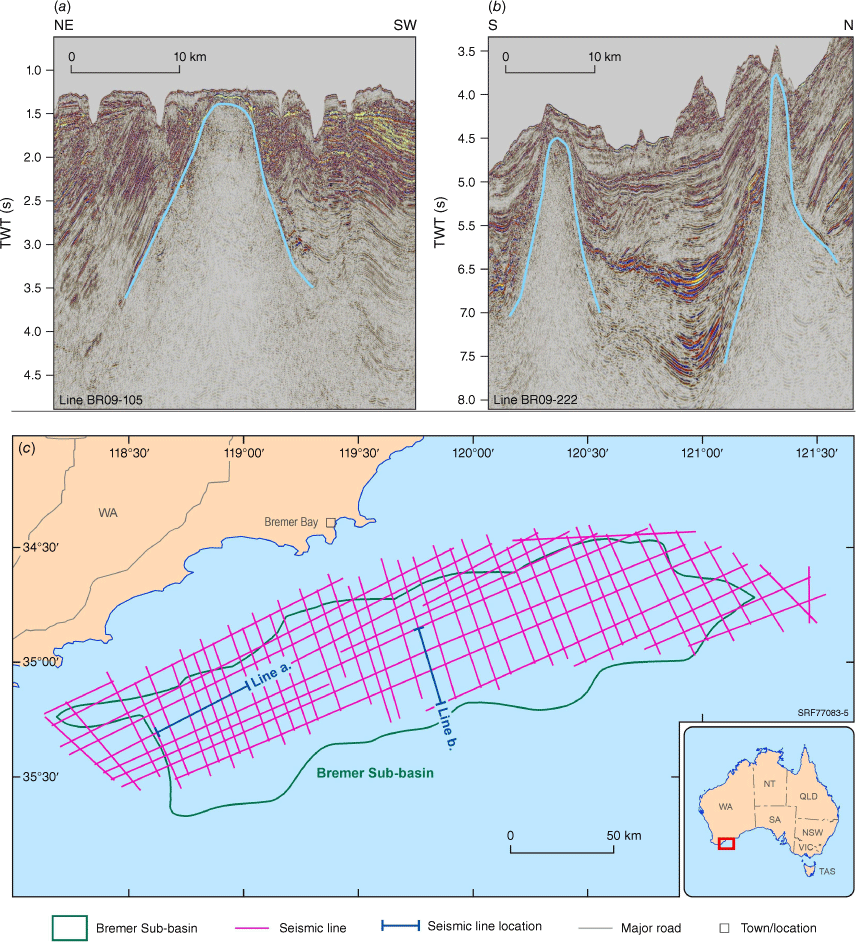
Feitz et al. (2022) conducted UHS feasibility analyses on the Kilroo Formation in the anticlinal Mercury structure in the central Polda Basin (Fig. 7). The Mercury structure, located 60 km offshore and 200 km away from Port Lincoln, has a net halite thickness >1000 m and total potential area of 217 km2 (Feitz et al. 2022). Well data from Mercury 1 suggests the basin has a low thermal gradient (1.7–2.1°C/100 m) and an overburden pressure gradient of approximately 18 pounds per gallon, providing effective gas operation pressure for UHS. The Caglayan et al. (2020) methodology was used to estimate a UHS capacity for a conceptual design of cylindrical halite caverns with 60 m and 100 m diameters for a depth range of 1650–2000 m with a water depth of 77 m. The storage capacities of these conceptual caverns were estimated to be 240 GWh and 665 GWh, respectively (Feitz et al. 2022). To put energy storage scale into perspective, one of Australia’s largest energy storage resources, the Snowy 2.0 pumped hydro project, will have a storage capacity of 350 GWh once operating in 2026 (Snowy Hydro 2020).
True vertical depth of the Kilroo Formation in the offshore Polda Basin. Red polygons represent three potential UHS areas within the Mercury structure salt deposits identified by Feitz et al. (2022).
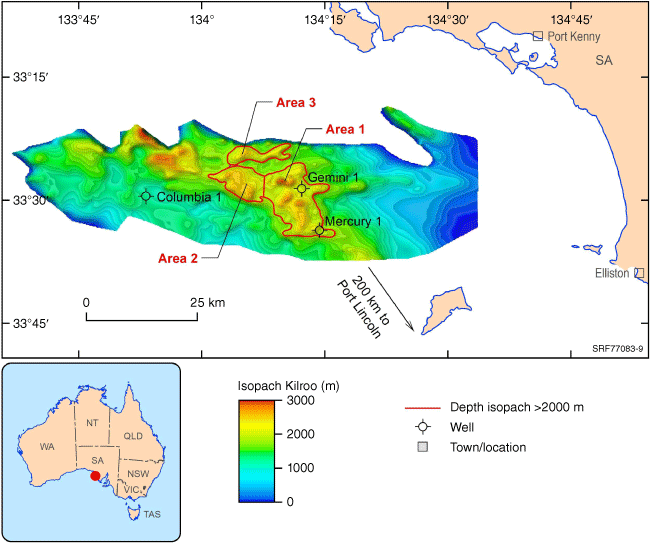
Adavale Basin – UHS prospects in a proven thick salt in an onshore basin
The Boree Salt in the Adavale Basin is the only proven thick halite in eastern Australia and presents a potential option for onshore UHS, given the basin’s strategic location relatively close to industrial infrastructure, existing pipelines and good renewable energy sources. A preliminary 3D model by Paterson et al. (2022) identified three main salt bodies that may be suitable for salt cavern construction and UHS (Fig. 8a). The 3D model was generated by integrating well log interpretations, time-based maps from the 1960s, 2D seismic lines from the 1980s and the results of Boyd Petrosearch’s (2010) investigation on potash and salt in the region (Paterson et al. 2022). The largest salt body, intersected by four wells (Bury 1, Alva 1, Bonnie 1 and Stafford 1, Fig. 8b), appears to be a suitable target for further characterisation and potential construction of salt caverns (Paterson et al. 2022). Additional salt bodies were intersected at Rosebank 1 and Boree 1. The salt in Boree 1 is >500 m, but its depth (1923–2426 m) requires careful planning for constructing UHS at this location. Ennis-King et al. (2021) reported that high pressure and temperature below 1800 m might lead to salt deformation and cavern instability issues.
(a) Adavale Basin 3D view of geological transects, three Boree Salt bodies and faults from Paterson et al. (2022). Bury 1 well intersected the main salt body. (b) Thickness of Boree Salt in the Adavale Basin.
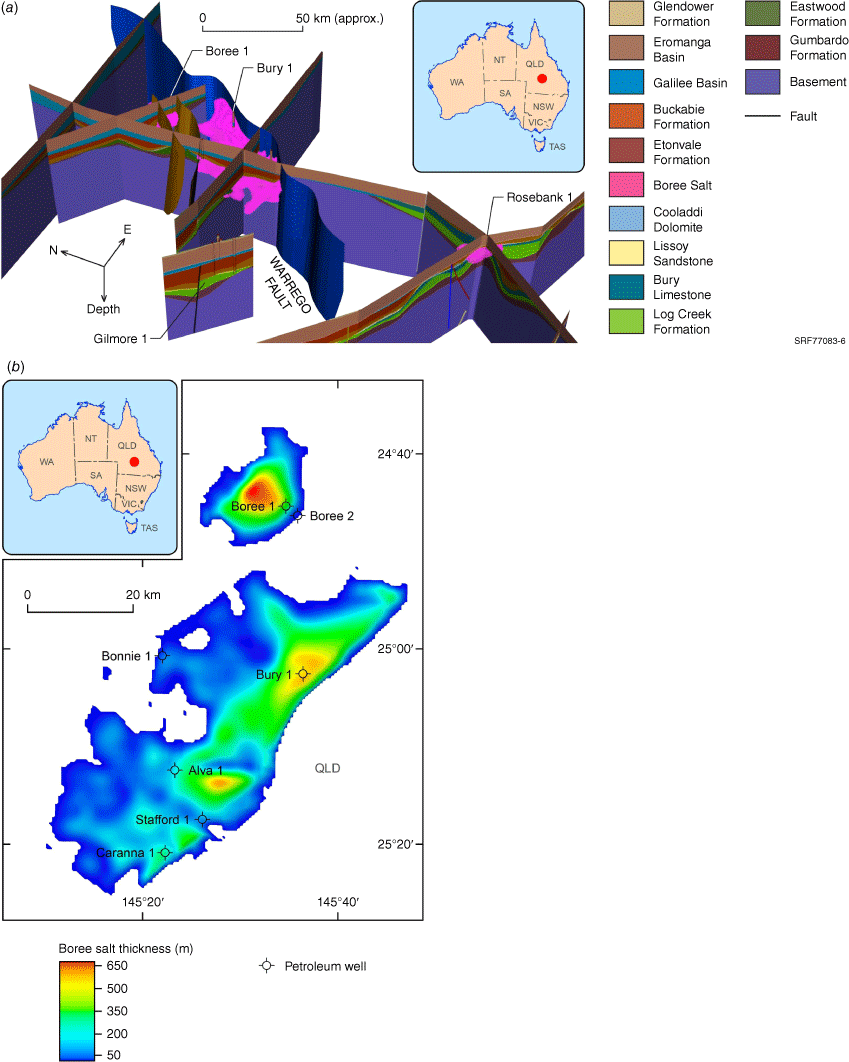
Officer Basin – onshore frontier basin for UHS
Evaporites in the Tonian Browne Formation have been intersected in seven wells in the western Officer Basin (Browne 1, BMR Madley 1, GSWA Lancer 1, GSWA Empress 1, Kanpa 1, Yowalga 3 and Kutjara 1), and halite has also been drilled in the eastern Officer Basin. Previous studies (Simeonova and Iasky 2005; Carr et al. 2012) have identified shallow expressions of possible salt diapirism in the western Officer Basin. Frontier, regional-scale reconnaissance of 2D seismic profiles identified a first-pass inventory of possible large salt leads suitable for UHS. Although the area is only covered by broadly spaced 2D seismic profiles of mixed recording vintage and image processing quality, the sampling was sufficient to reveal the scale and depth of potential salt structures (Fig. 9). To date, the crests of the shallowest possible salt-related features occur around 500 m depth in the subsurface.
(a) and (b) are salt‐lead features on 2D seismic cross‐sections identified in the western and eastern regions of the Officer Basin. (c) A map showing 2D seismic surveys over the Officer Basin and Arckaringa Sub‐basin with OZ SEEBASE (Geognostics 2021) map in the background. MSL, mean sea level; TWT (s), two-way time in seconds.
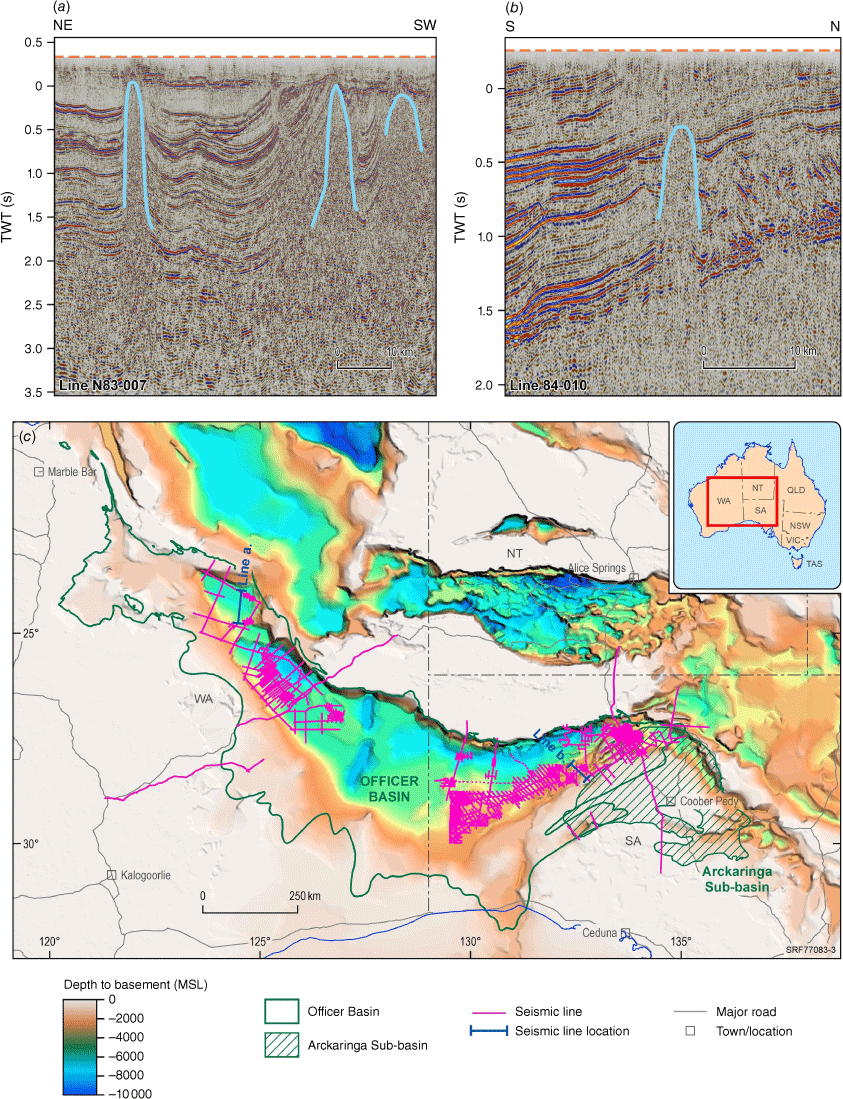
A preliminary investigation on the recently acquired Geoscience Australia AEM dataset (Ley-Cooper 2020, 2021) has shown a correlation between anomalies in AEM conductivity sections to the areas of known and potential salt features over the western Officer Basin. The AEM data was compared to potential salt leads identified in seismic (Fig. 9) and the Salt Intrusions dataset from the Geological Survey of Western Australia’s Western Australian Petroleum and Geothermal Management System (WAPIMS). Other supporting datasets, such as borehole data, geological maps, gravity, magnetics and elevation data, were included in the assessment. Areas identified as hosting salt intrusions in the WAPIMS dataset correlated with potential salt-related features observable in the seismic and AEM data (Fig. 10). Several AEM conductivity sections showed truncation of horizontal conductive horizons by more resistive geology in areas highlighted by the Salt Intrusions dataset and seismic interpretation. In most cases, these features also correlated with anomalous features observed in the gravity and magnetics datasets.
The Woolnough diapir that was identified as hosting salt intrusions in the seismic dataset correlated with potential salt-related features observable in the AEM data.
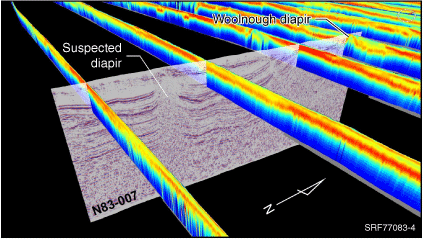
Integration of seismic and AEM methods has helped to refine the subsurface distribution of such features, where they can be observed extending across several of the nominally 20 km-spaced AusAEM (Ley-Cooper 2021) lines. In some cases, the distribution of the potential salt features can be extended a significant distance (over 40 km) from the current extent highlighted in the Salt Intrusions dataset by integrating the AEM data with other datasets.
Given the regional sparsity of subsurface data, a multi-parameter, play-based, common-risk segment mapping approach, similar to that used with other resource types, could elucidate key subsurface uncertainties and risks. De-risking requires a multi-disciplinary approach that includes a combination of geophysical methods and an increase in survey density and perhaps regional hydrogeo-chemistry. Integrated geophysical data interpretation (seismic, AEM, gravity and magnetic with shallow seismic images) could help change the subsurface understanding at both a play and prospect level. Although new seismic acquisition would improve the current subsurface understanding, a considerable cost-effective uplift could be gained through selected reprocessing of key seismic profiles, particularly given that most seismic data is over 20 years old.
Amadeus Basin – options for UHS and natural hydrogen play development in an onshore basin
The Amadeus Basin has two thick halite sequences: (1) the Cambrian Chandler Formation and (2) the Tonian Gillen Formation (Livesey 2015). The Chandler Formation salt is an extensive evaporite deposit with an average halite grade of 88.6%, with individual layers of high-grade halite (~98%) (Livesey 2015; Scrimgeour 2015). Although its structure can be flat-lying at Mt Charlotte 1 and Magee 1 (thickness varying between 200 and 261 m) (Livesey 2015), the Chandler Formation salt also exists as diapirs at Bluebush 1 (>400 m) (Fig. 11). This salt deposit has been targeted as an underground salt mine and deep geological waste repository under the Chandler Project (Scrimgeour 2015; Tellus Holdings 2022).
Petroleum wells with halite in the Amadeus Basin with OZ SEEBASE (Geognostics 2021) map on the background.
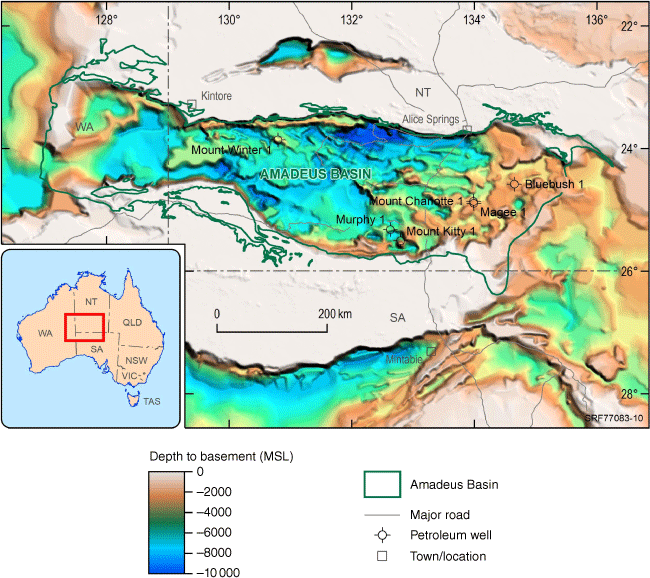
The Tonian Gillen Formation contains a massive halite in between anhydrite/dolostone units (Plummer 2021). The evaporites outcrop in the northern Amadeus Basin and occur as diapiric structures and salt pillows at depths below 1900 m at Magee 1, Murphy 1 and Mt Charlotte 1 in the southern Amadeus Basin (Plummer 2021) (Fig. 11). The thickness also varies from approximately 200 m at Magee 1 and Mt Charlotte 1 to >700 m at Murphy 1 (Livesey 2015).
The potential for UHS construction in the Amadeus Basin is likely to be in the diapiric structures. However, further analysis is required to determine UHS feasibility in the Gillen Formation and Chandler Formation salts. Natural hydrogen and helium have been reservoired in the Amadeus Basin at Mt Kitty where sealed by thin anhydrite (Boreham et al. 2018).
Discussion and conclusions
Australia has many examples of saline giants with thick halite sequences and salt structures that may be suitable for UHS. The age, facies and structural setting of rock packages can give a guide to where evaporites may extend beyond the proven well intersections. However, geophysics (seismic, AEM) will be the primary exploration tool, followed by drilling to test the thickness and purity of the interpreted salt bodies. Some basins (Amadeus and Canning) have more than one proven salt ‘play’ as well as the potential for natural hydrogen accumulations. The subsurface data sets, skills and exploration strategies developed by the petroleum industry can be repurposed for these new targets – thick salt for UHS and salt as a seal for natural hydrogen accumulations.
Economic and engineering considerations can high-grade identified prospects, and the Hydrogen Economic Fairways Tool (Geoscience Australia 2022) can be used for an initial screening of locations. For example, the Darling Basin in western NSW has no proven salt in the subsurface except for two intervals (Cambrian and Devonian) where evaporitic facies may have developed, and it is located closer to where UHS may be needed than proven salts in the western Officer Basin.
Data availability
Data utilised in this study were obtained from the Government of Western Australia’s Petroleum & Geothermal Information Management System (WAPIMS), National Offshore Petroleum Information Management System (NOPIMS), National Petroleum Wells Database, Geoscience Australia Product Catalogue (https://ecat.ga.gov.au/geonetworks) and South Australian Resources Information Gateway.
Acknowledgements
The authors wish to thank Aleks Kalinowski, Barry Bradshaw, Kristina Anastasi and two anonymous reviewers for their thoughtful comments on the paper as well as Chris Evenden, Malcolm Nicoll and Errol Fries for figures and photographs. All authors (besides S. V.) publish with the permission of the CEO, Geoscience Australia.
References
ACES DELTA (2022) Advanced clean energy storage hub. Available at https://aces-delta.com/hubs/Alder JD, Bembrick C, Hartung-Kagi B, Mullard B, Pratt DA, Scott J, Shaw RD (1998) A re-assessment of the petroleum potential of the Darling Basin: a Discovery 2000 initiative. The APPEA Journal 38, 278–312.
| A re-assessment of the petroleum potential of the Darling Basin: a Discovery 2000 initiative.Crossref | GoogleScholarGoogle Scholar |
Backé G, Baines G, Giles D, Preiss W, Alesci A (2010) Basin geometry and salt diapirs in the Flinders Ranges, South Australia: Insights gained from geologically-constrained modelling of potential field data. Marine and Petroleum Geology 27, 650–665.
| Basin geometry and salt diapirs in the Flinders Ranges, South Australia: Insights gained from geologically-constrained modelling of potential field data.Crossref | GoogleScholarGoogle Scholar |
Bain JE, Weyand J, Weber M (1991) Resolving complex salt features using gravity and magnetics. American Association of Petroleum Geologist (AAPG) Invited Lecture Series.
Banham SG, Mountney NP (2013) Evolution of fluvial systems in salt-walled mini-basins: a review and new insights. Sedimentary Geology 296, 142–166.
| Evolution of fluvial systems in salt-walled mini-basins: a review and new insights.Crossref | GoogleScholarGoogle Scholar |
BloombergNEF (2019) Hydrogen: the economics of storage – storing clean molecules at scale. Technical Report. (Bloomberg)
BloombergNEF (2020) Hydrogen economy outlook. Available at https://data.bloomberglp.com/professional/sites/24/BNEF-Hydrogen-Economy-Outlook-Key-Messages-30-Mar-2020.pdf
Boreham CJ, Edwards DS, Poreda RJ, Darrah TH, Zhu R, Grosjean E, Main P, Waltenberg K, Henson PA (2018) Helium in the Australian liquefied natural gas economy. The APPEA Journal 58, 209–237.
| Helium in the Australian liquefied natural gas economy.Crossref | GoogleScholarGoogle Scholar |
Boreham CJ, Edwards DS, Czado K, Rollet N, Wang L, van der Wielen S, Champion D, Blewett R, Feitz A, Henson PA (2021) Hydrogen in Australian natural gas: occurrences, sources and resources. The APPEA Journal 61, 163–191.
| Hydrogen in Australian natural gas: occurrences, sources and resources.Crossref | GoogleScholarGoogle Scholar |
Boucot AJ, Xu C, Scotese CR, Morley RJ (2013) ‘Phanerozoic paleoclimate: an atlas of lithologic indicators of climate’. Society for Sedimentary Geology: Concepts in Sedimentology and Paleontology 11. 478 p. (Society for Sedimentary Geology: Tulsa, Oklahoma, USA)
Boult P, Bennett PJ, Freeman A (2012) Structure, Stratigraphy and Petroleum Potential of the Central Officer Basin, South Australia. American Association of Petroleum Geologists (AAPG) Search and Discovery Article #10469. Available at https://www.searchanddiscovery.com/pdfz/documents/2012/10469boult/ndx_boult.pdf.html
Bradshaw J (1991) Description and depositional model of the Chandler Formation: a Lower Cambrian evaporite and carbonate sequence, Amadeus Basin, central Australia. In ‘Geological and Geophysical Studies in the Amadeus Basin, Central Australia, Bulletin 236’, pp. 227–244 (Geoscience Australia, Canberra) Available at http://pid.geoscience.gov.au/dataset/ga/33
Bradshaw BE (Compiler) (2005) ‘Geology and petroleum potential of the Bremer Sub-basin, offshore southwestern Australia’. Record, 2005/21. (Geoscience Australia)
Bradshaw BE, Salman A, Bradshaw J, Doyle S (2013) The influence of pre-rift salt on petroleum systems and plays in the Bremer Basin, Australia. In ‘Proceedings of the 2013 South East Asia Petroleum Exploration Society (SEAPEX) Conference’, April 2013, Singapore. pp. 1–37. (American Association of Petroleum Geologist (AAPG)/Datapages Combined Publication Database) Available at https://archives.datapages.com/data/southeast-asia-petroleum-exploration-society/data/026/026001/1_seapex0260017.htm
Bradshaw BE, Khider K, MacFarlane S, Rollet N, Carr L, Henson P (2020) ‘Tectonostratigraphic evolution of the Centralian Superbasin (Australia) revealed by three-dimensional well correlations.’ (Geoscience Australia: Canberra)
| Crossref |
Bruno MS (2005) ‘Geomechanical analysis and design considerations for thin-bedded salt caverns.’ (Terralong Technologies: USA)
Caglayan DG, Weber N, Heinrichs HU, Linßen J, Robinius M, Kukla PA, Stolten D (2020) Technical potential of salt caverns for hydrogen storage in Europe. International Journal of Hydrogen Energy 45, 6793–6805.
| Technical potential of salt caverns for hydrogen storage in Europe.Crossref | GoogleScholarGoogle Scholar |
Canadian Superior Oil (1976) Well completion report Pendock 1. (Government of Western Australia Department of Mines, Industry Regulation and Safety: WA, Australia) Available at https://wapims.dmp.wa.gov.au/WAPIMS/Search/Wells
Carr L, Korsch R, Mory A, Hocking R, Marshall S, Costelloe R, Holzschuh J, Maher J (2012) Structural and stratigraphic architecture of Australia’s frontier onshore sedimentary basins: the Western Officer and Southern Carnarvon basins, Western Australia. The APPEA Journal 52, 670
| Structural and stratigraphic architecture of Australia’s frontier onshore sedimentary basins: the Western Officer and Southern Carnarvon basins, Western Australia.Crossref | GoogleScholarGoogle Scholar |
Chedwynd G (2021) ‘Irish green hydrogen potential spurs ESB to subsea storage partnership.’ (Upstream Energy Explored) Available at https://www.upstreamonline.com/energy-transition/irish-green-hydrogen-potential-spurs-esb-to-subsea-storage-partnership/2-1-1016687
Connors KA, Wong S, Vilhena JFM, Rees S, Feitz A (2022) ‘Canning Basin AusAEM interpretation: hydrogen storage potential and multilayered mapping.’ (Geoscience Australia: Canberra)
| Crossref |
Continental oil company of Australia (1966) Yaringa No. 1 well, West Australia stratigraphic drilling project. Well completion report. (Government of Western Australia Department of Mines, Industry Regulation and Safety: WA, Australia) Available at https://wapims.dmp.wa.gov.au/WAPIMS/Search/Wells
Cornot-Gandolphe S (2019) Underground Gas Storage in the World - 2018 Status. Rueil Malmaison. Available at https://cdn2.hubspot.net/hubfs/1982707/Overview of underground gas storage in the world 2018 (1).pdf
Cunneen J, Buckingham A, D’Ercole C (2019) Rift initiation on Australia’s southern margin: insights from the Bremer Sub-basin. ASEG Extended Abstracts 2019, 1–3.
| Rift initiation on Australia’s southern margin: insights from the Bremer Sub-basin.Crossref | GoogleScholarGoogle Scholar |
dCarbonX (2021) ‘Large scale offshore hydrogen storage to enable Ireland’s Energy Transition.’ (Hydrogen Ireland) Available at https://hydrogenireland.org/2021/10/06/working-paper-dcarbonx-large-scale-offshore-hydrogen-storage-to-enable-irelands-energy-transition/
DCCEEW (2022) Growing Australia’s hydrogen industry. Available at https://www.dcceew.gov.au/energy/hydrogen
Debacker T, Connors K, Lee JU, Watters S, Clifford J, Plummer P, Menpes S (2016) Exploring the sub-salt play in the frontier Amadeus Basin – Insights from potential field data analysis. In ‘Annual Geoscience Exploration Seminar Proceeding’, March 2016, Alice Springs, NT. pp. 15–16. (Northern Territory Geological Survey: Darwin)
Debure M, Lassin A, Marty NC, Claret F, Virgone A, Calassou S, Gaucher EC (2019) Thermodynamic evidence of giant salt deposit formation by serpentinization: an alternative mechanism to solar evaporation. Scientific Reports 9, 11720
| Thermodynamic evidence of giant salt deposit formation by serpentinization: an alternative mechanism to solar evaporation.Crossref | GoogleScholarGoogle Scholar |
Delhi Petroleum (1984) Moorowie 1 – Mudlog. Report. Available at https://sarigbasis.pir.sa.gov.au/WebtopEw/ws/samref/sarig1/cat0/MSearch
Duffy OB, Fernandez N, Hudec MR, Jackson MPA, Burg G, Dooley TP, Jackson CA-L (2017) Lateral mobility of minibasins during shortening: Insights from the SE Precaspian Basin, Kazakhstan. Journal of Structural Geology 97, 257–276.
| Lateral mobility of minibasins during shortening: Insights from the SE Precaspian Basin, Kazakhstan.Crossref | GoogleScholarGoogle Scholar |
Dunster JN, Kruse PD, Duffett ML, et al. (2007) ‘Geology and resource potential of the southern Georgina Basin’. Digital information package DIP007. (Northern Territory Geological Survey: Darwin)
Ennis-King J, Michael K, Strand J, Sander R, Green C (2021) Underground storage of hydrogen: mapping out the options for Australia. Project RP1-1.04 Deliverable 5: Final Summary Report. (Future Fuels CRC)
ESB (2021) ESB announces Green Atlantic at Moneypoint. Electricity Supply Board. Available at https://esb.ie/tns/press-centre/2021/2021/04/09/esb-announces-green-atlantic-@-moneypoint
Feitz A, Wang L, Rees S, Carr L (2022) ‘Feasibility of underground hydrogen storage in a salt cavern in the offshore Polda Basin.’ (Geoscience Australia: Canberra)
| Crossref |
Geognostics (2021) ‘OZ SEEBASE® 2021 (March 2021).’ (Geognostics Australia Pty Ltd) Available at https://www.geognostics.com/oz-seebase-2021
Geoscience Australia (2022) Hydrogen Economic Fairways Tool. Available at https://www.ga.gov.au/scientific-topics/energy/resources/hydrogen/hydrogen-tools
Gill G, Cowan G (2008) ‘Adavale Basin, Queensland underground salt cavern potential.’ (Underground Storage Solutions: Brisbane, Australia)
Haines PW, Allen HJ (2017) ‘Geological reconnaissance of the southern Murraba Basin, Western Australia: revised stratigraphic position within the Centralian Superbasin and hydrocarbon potential’. Record 2017/4. 38 p. (Geological Survey of Western Australia) Available at https://dmp.wa.gov.au/Documents/Geological-Survey/GSWA-TheMurrabaBasin_PeterHaines.pdf
Haines PW, Allen HJ (2020) ‘World’s oldest regional salt seal in the Amadeus and Officer Basins: implications for subsalt helium and hydrocarbons. GSWA 2020 extended abstracts.’ (Geological Survey of Western Australia)
Hall LS, Wang L, Bailey AHE, Orr ML, Owens R, Jarrett AJM, Lech ME, Skeers N, Reese B, Woods M (2020) ‘Petroleum prospectivity of the Beetaloo Sub-basin. Technical appendix for the Geological and Bioregional Assessment Program: Stage 2.’ (Department of the Environment and Energy, Bureau of Meteorology, CSIRO and Geoscience Australia: Australia)
Hévin G (2019) Underground storage of hydrogen in salt caverns. In ‘European Workshop on Underground Energy Storage’, November 7–8 2019, Paris. (The European Network for Research in Geo‐Energy (ENeRG)) Available at https://energnet.eu/european‐workshop‐on‐underground‐energy‐storage‐presentations/
Hill PJ (1992) Capricorn and Northern Tasman Basins: Structure and Depositional Systems. Exploration Geophysics 23, 153–161.
| Capricorn and Northern Tasman Basins: Structure and Depositional Systems.Crossref | GoogleScholarGoogle Scholar |
Hovland M, Rueslåtten H, Johnsen HK (2018a) Large salt accumulations as a consequence of hydrothermal processes associated with Wilson cycles: A review Part 1: Towards a new understanding. Marine and Petroleum Geology 92, 987–1009.
| Large salt accumulations as a consequence of hydrothermal processes associated with Wilson cycles: A review Part 1: Towards a new understanding.Crossref | GoogleScholarGoogle Scholar |
Hovland M, Rueslåtten H, Johnsen HK (2018b) Large salt accumulations as a consequence of hydrothermal processes associated with ‘Wilson cycles’: A review, Part 2: Application of a new salt-forming model on selected cases. Marine and Petroleum Geology 92, 128–148.
| Large salt accumulations as a consequence of hydrothermal processes associated with ‘Wilson cycles’: A review, Part 2: Application of a new salt-forming model on selected cases.Crossref | GoogleScholarGoogle Scholar |
Hsu KJ (1972) Origin of saline giants: a critical review after the discovery of the Mediterranean evaporite. Earth-Science Reviews 8, 371–396.
| Origin of saline giants: a critical review after the discovery of the Mediterranean evaporite.Crossref | GoogleScholarGoogle Scholar |
Innovation Origins (2022) EBN and TNO research: Offshore hydrogen storage seems feasible’. Available at https://innovationorigins.com/en/selected/ebn-and-tno-research-offshore-hydrogen-storage-seems-feasible/
International Energy Agency (2022) Global Hydrogen Review 2022. Available at https://www.iea.org/reports/global-hydrogen-review-2022
Jackson M, Hudec M (2017) Seismic Interpretation of Salt Structures. In ‘Salt Tectonics: Principles and Practice’. (Eds MPA Jackson, MR Hudec) pp. 364–398. (Cambridge University Press: Cambridge)
| Crossref |
Jones IF, Davison I (2014) Seismic imaging in and around salt bodies. Interpretation. 2, SL1–SL20.
| Seismic imaging in and around salt bodies.Crossref | GoogleScholarGoogle Scholar |
Kernen R, Lehrmann A, Poe P (2021) Lithostratigraphy and chemostratigraphy of salt diapir sedimentary inclusions: unravelling Ediacaran salt–sediment interaction in the Flinders Ranges. MESA Journal 95(2), 4–29. (Government of South Australia, Department for Energy and Mining)
Khalifa MKH, Mills KJ (2022) Predicting sequence stratigraphic architecture and its implication for hydrocarbon reservoir potential of the uppermost Silurian through Lower Devonian Winduck Interval, central Darling Basin of western New South Wales, SE Australia. Marine and Petroleum Geology 142, 105725
| Predicting sequence stratigraphic architecture and its implication for hydrocarbon reservoir potential of the uppermost Silurian through Lower Devonian Winduck Interval, central Darling Basin of western New South Wales, SE Australia.Crossref | GoogleScholarGoogle Scholar |
Kovalevych VM, Zang WL, Peryt TM, Khmelevska OV, Halas S, Iwasinska-Budzyk I, Boult PJ, Heithersay PS (2006) Deposition and chemical composition of Early Cambrian salt in the eastern Officer Basin, South Australia. Australian Journal of Earth Sciences 53, 577–593.
| Deposition and chemical composition of Early Cambrian salt in the eastern Officer Basin, South Australia.Crossref | GoogleScholarGoogle Scholar |
Ley-Cooper Y (2020) ‘AusAEM 02 WA/NT 2019-20 Airborne Electromagnetic Survey.’ (Geoscience Australia: Canberra) Available at http://pid.geoscience.gov.au/dataset/ga/140156
Ley-Cooper Y (2021) ‘AusAEM (WA) 2020-21, Earaheedy & Desert Strip Airborne Electromagnetic Survey Blocks TEMPEST® airborne electromagnetic data and GALEI conductivity estimates.’ (Geoscience Australia: Canberra) Available at http://pid.geoscience.gov.au/dataset/ga/145265
Livesey J (2015) Geophysical interpretation of the Chandler Salt Deposit, Amadeus Basin. In ‘Annual Geoscience Exploration Seminar (AGES) 2015. Record of abstracts’. pp. 93–94. (Northern Territory Geological Survey)
Logan BW (1987) The MacLeod evaporite basin, Western Australia. AAPG Memoir 44, 140
Lopez-Lazaro C, Bachaud P, Moretti I, Ferrando N (2019) Predicting the phase behavior of hydrogen in NaCl brines by molecular simulation for geological applications. BSGF-Earth Sciences Bulletin 190, 7
| Predicting the phase behavior of hydrogen in NaCl brines by molecular simulation for geological applications.Crossref | GoogleScholarGoogle Scholar |
Løseth H, Gading M, Wensaas L (2009) Hydrocarbon leakage interpreted on seismic data. Marine and Petroleum Geology 26, 1304–1319.
| Hydrocarbon leakage interpreted on seismic data.Crossref | GoogleScholarGoogle Scholar |
Magee C, Hunt-Stewart E, Jackson CAL (2013) Volcano growth mechanisms and the role of sub-volcanic intrusions: Insights from 2D seismic reflection data. Earth and Planetary Science Letters 373, 41–53.
| Volcano growth mechanisms and the role of sub-volcanic intrusions: Insights from 2D seismic reflection data.Crossref | GoogleScholarGoogle Scholar |
Mauduit T, Gaullier V, Brun JP, Guerin G (1997) On the asymmetry of turtle-back growth anticlines. Marine and Petroleum Geology 14, 763–771.
| On the asymmetry of turtle-back growth anticlines.Crossref | GoogleScholarGoogle Scholar |
McNee F, Gorter J, Glass F (2021) New age dating of evaporites in Canning Basin, WA, Australia. A case study based on samples from the Frome Rocks Salt Diapir. In ‘Proceedings of the 3rd AEGC: Geosciences for Sustainable World Conference’, 15–20 September 2021.
Mernagh TP, Bastrakov EN, Clarke JDA, de Caritat P, English PM, Howard FJF, Jaireth S, Magee JW, McPherson AA, Roach IC, Schroder IF, Thomas M, Wilford JR (2013) ‘A Review of Australian Salt Lakes and Assessment of their Potential for Strategic Resources’. Record 2013/039. (Geoscience Australia: Canberra) Available at http://pid.geoscience.gov.au/dataset/ga/76454
Michael K, Ennis-King J, Strand J, Sander R, Green C (2021) Underground storage of hydrogen: mapping out the options for Australia. In ‘RISC 2021, Hydrogen storage potential of depleted oil and gas fields in Western Australia: literature review and scoping study’. Report 221. 179 p. (Geological Survey of Western Australia)
Molyneux S, Doyle S (2021) Salt in the Vulcan sub-basin, NW Australia: observations from high-quality 3D seismic data and implications for palaeogeography. The APPEA Journal 61, 684–687.
| Salt in the Vulcan sub-basin, NW Australia: observations from high-quality 3D seismic data and implications for palaeogeography.Crossref | GoogleScholarGoogle Scholar |
Moretti I, Brouilly E, Loiseau K, Prinzhofer A, Deville E (2021) Hydrogen emanations in intracratonic areas: new guidelines for early exploration basin screening. Geosciences 11, 145
| Hydrogen emanations in intracratonic areas: new guidelines for early exploration basin screening.Crossref | GoogleScholarGoogle Scholar |
Mory AJ (1991) ‘Geology of the offshore Bonaparte Basin, northwestern Australia. Vol. 1.’ (Geological Survey of Western Australia)
Nelson RG, Crabb TN, Gerdes RA (1986) A review of geophysical exploration in the Polda Basin, South Australia. The APPEA Journal 26, 319–333.
| A review of geophysical exploration in the Polda Basin, South Australia.Crossref | GoogleScholarGoogle Scholar |
Pajonpai N, Bissen R, Pumjan S, Henk A (2022) Shape design and safety evaluation of salt caverns for CO2 storage in northeast Thailand. International Journal of Greenhouse Gas Control 120, 103773
| Shape design and safety evaluation of salt caverns for CO2 storage in northeast Thailand.Crossref | GoogleScholarGoogle Scholar |
Paterson R, Feitz A, Wang L, Rees S, Keetley J (2022) ‘A preliminary 3D model of the Boree Salt in the Adavale Basin, Queensland.’ (Geoscience Australia: Canberra)
| Crossref |
Plummer PS (2021) The Neoproterozoic Gillen Formation, Amadeus Basin, central Australia: an intra-salt petroleum system and viable exploration target? The APPEA Journal 61, 236–252.
| The Neoproterozoic Gillen Formation, Amadeus Basin, central Australia: an intra-salt petroleum system and viable exploration target?Crossref | GoogleScholarGoogle Scholar |
Preiss WV (2000) The Adelaide Geosyncline of South Australia and its significance in Neoproterozoic continental reconstruction. Precambrian Research 100, 21–63.
| The Adelaide Geosyncline of South Australia and its significance in Neoproterozoic continental reconstruction.Crossref | GoogleScholarGoogle Scholar |
Prinzhofer A, Cissé CST, Diallo AB (2018) Discovery of a large accumulation of natural hydrogen in Bourakebougou (Mali). International Journal of Hydrogen Energy 43, 19315–19326.
| Discovery of a large accumulation of natural hydrogen in Bourakebougou (Mali).Crossref | GoogleScholarGoogle Scholar |
Rodinia Oil (2011) Mulyawara-1. Well completion report. Available at https://sarigbasis.pir.sa.gov.au/WebtopEw/ws/samref/sarig1/image/DDD/MULYAWARA 001.zip
RVO (2022) Excelling in hydrogen - Dutch technology for a climate-neutral world. Available at https://www.rvo.nl/sites/default/files/2022-05/NL-Dutch-solutions-for-a-hydrogen-economy-V-April-2022-DIGI.pdf
Schienteie R, Campbell B, Greenfield P, Tran-Dinh N, Ennis-King J, Midgley D (2022) Microbial implications of subsurface hydrogen gas storage: Impacts from an Australian context. In ‘Conference Proceeding of the 21st Australian Organic Geochemistry Conference: Resources, Environments and Renewables’, Sydney, 27−29 November 2022.
Schmid S (2017) Neoproterozoic evaporites and their role in carbon isotope chemostratigraphy (Amadeus Basin, Australia). Precambrian Research 290, 16–31.
| Neoproterozoic evaporites and their role in carbon isotope chemostratigraphy (Amadeus Basin, Australia).Crossref | GoogleScholarGoogle Scholar |
Scribano V, Carbone S, Manuella FC, Hovland M, Rueslåtten H, Johnsen HK (2017) Origin of salt giants in abyssal serpentinite systems. International Journal of Earth Sciences 106, 2595–2608.
| Origin of salt giants in abyssal serpentinite systems.Crossref | GoogleScholarGoogle Scholar |
Scrimgeour IR (2015) Overview of mineral and petroleum exploration and production in 2014. In ‘Annual Geoscience Exploration Seminar (AGES) 2015. Record of abstracts’. pp. 1–16. (Northern Territory Geological Survey)
Simeonova AP, Iasky RP (2005) Seismic mapping, salt deformation, and hydrocarbon potential of the central western Officer Basin, Western Australia. Report 98. 51 p. (Western Australia Geological Survey)
Singh D, Kumar PC, Sain K (2016) Interpretation of gas chimney from seismic data using artificial neural network: A study from Maari 3D prospect in the Taranaki basin, New Zealand. Journal of Natural Gas Science and Engineering 36, 339–357.
| Interpretation of gas chimney from seismic data using artificial neural network: A study from Maari 3D prospect in the Taranaki basin, New Zealand.Crossref | GoogleScholarGoogle Scholar |
Snowy Hydro (2020) Snowy 2.0 project update, August 2020. Available at https://www.snowyhydro.com.au/snowy-20/about/
South Australia Department for Energy and Mining (2021) Polda Basin. (Government of South Australia) Available at https://www.petroleum.sa.gov.au/geology-and-prospectivity/mesozoic-basins/polda-basin
Stadtler C, Fichler C, Hokstad K, Fotland B, Gram C, Hanssen P, Myrlund EA, Wienecke S (2010) Salt Imaging with Gravity Gradiometry and Magnetic Data – Nordkapp Basin, Barents Sea. In ‘72nd EAGE Conference and Exhibition incorporating SPE EUROPEC 2010’. cp-161-00151. (European Association of Geoscientists & Engineers)
Stalker L, Talukder A, Strand J, Josh M, Faiz M (2022) Gold (hydrogen) rush: risks and uncertainties in exploring for naturally occurring hydrogen. The APPEA Journal 62, 361–380.
| Gold (hydrogen) rush: risks and uncertainties in exploring for naturally occurring hydrogen.Crossref | GoogleScholarGoogle Scholar |
Stewart SA (2018) Hormuz salt distribution and influence on structural style in NE Saudi Arabia. Petroleum Geoscience 24, 143–158.
| Hormuz salt distribution and influence on structural style in NE Saudi Arabia.Crossref | GoogleScholarGoogle Scholar |
Struckmeyer HIM, Symonds PA, Fellows M, Scott DL (1994) ‘Structural and Stratigraphic Evolution of the Townsville Basin, Townsville Trough, Offshore Northeastern Australia.’ (Australian Geological Survey Organisation) Available at http://pid.geoscience.gov.au/dataset/ga/14757
Szatmari P, Moré de Lima C, Fontaneta G, de Melo Lima N, Zambonato E, Menezes MR, Bahniuk J, Coelho SL, Figueiredo M, Florencio CP, Gontijo R (2021) Petrography, geochemistry and origin of South Atlantic evaporites: the Brazilian side. Marine and Petroleum Geology 127, 104805
| Petrography, geochemistry and origin of South Atlantic evaporites: the Brazilian side.Crossref | GoogleScholarGoogle Scholar |
Tellus Holdings (2022) Chandler Facility Description. Available at https://tellusholdings.com/projects/chandler-facility/chandler-facility-description/
Van de Beuque S, Stagg HMJ, Sayers J, Willcox JB, Symonds PA (2003) ‘Geological Framework of the Northern Lord Howe Rise and Adjacent Areas’. Record 2003/001. (Geoscience Australia: Canberra) Available at http://pid.geoscience.gov.au/dataset/ga/41856
Virs R (2015) A comparative seismic study of gas chimney structures from active and dormant seepage sites offshore mid-Norway and west-Svalbard. GEO-3900 Master Thesis in Geology, The Arctic University of Norway, Tromsø, Norway.
Walter MR, Veevers JJ, Calver CR, Grey K (1995) Neoproterozoic stratigraphy of the Centralian superbasin, Australia. Precambrian Research 73, 173–195.
| Neoproterozoic stratigraphy of the Centralian superbasin, Australia.Crossref | GoogleScholarGoogle Scholar |
Warren JK (2010) Evaporites through time: Tectonic, climatic and eustatic controls in marine and nonmarine deposits. Earth-Science Reviews 98, 217–268.
| Evaporites through time: Tectonic, climatic and eustatic controls in marine and nonmarine deposits.Crossref | GoogleScholarGoogle Scholar |
Warren JK (2021) Evaporite deposits. In ‘Encyclopedia of Geology’, 2nd Edn. (Eds D Alderton, SA Elias) pp. 945–977. (Academic Press)
| Crossref |
Wells AT (1980) Evaporites in Australia. Record 198. Geoscience Australia, Canberra. http://pid.geoscience.gov.au/dataset/ga/51
Wilford J (2022) ‘High resolution conductivity mapping using regional AEM survey and machine learning.’ (Geoscience Australia: Canberra)
| Crossref |
Winker CD (1996) High-resolution seismic stratigraphy of a late Pleistocene submarine fan ponded by salt-withdrawal mini-basins on the Gulf of Mexico continental slope. In ‘Offshore Technology Conference’. (OnePetro)
Young GC, Lu J (2020) Asia–Gondwana connections indicated by Devonian fishes from Australia: palaeogeographic considerations. Journal of Palaeogeography 9, 8
| Asia–Gondwana connections indicated by Devonian fishes from Australia: palaeogeographic considerations.Crossref | GoogleScholarGoogle Scholar |
Zgonnik V (2020) The occurrence and geoscience of natural hydrogen: A comprehensive review. Earth-Science Reviews 203, 103140
| The occurrence and geoscience of natural hydrogen: A comprehensive review.Crossref | GoogleScholarGoogle Scholar |
Zhan Y (2019) ‘A seismic interpretation of the Broome Platform, Willara Sub-basin and Munro Arch of the Canning Basin, Western Australia.’ (Geological Survey of Western Australia)
![]() Marita Bradshaw is a Petroleum Geologist with over 40 years of experience in government and industry, at Geoscience Australia, Esso Australia and WMC Oil and Gas. She is a member of PESA, the Geological Society of Australia and the Steering Committee of the National Rock Garden. |
![]() Stephanie Rees is an Energy Analyst in the Low Carbon Geoscience and Advice at Geoscience Australia. She holds an undergraduate degree in Petroleum Geoscience and a PhD in Geophysics from The University of Adelaide. Besides geophysics, her research interests include hydrogen production, storage and economy. |
![]() Liuqi Wang is a Well Analyst at Geoscience Australia, working in the Minerals, Energy and Groundwater Division. He received his PhD in Petroleum Engineering and worked as a Research Fellow at the University of New South Wales before joining Geoscience Australia. Liuqi is a member of PESA and the European Association of Geoscientists and Engineers. |
![]() Michael Szczepaniak is an Exploration and Development Geophysicist, with over 25 years’ experience, having worked with several major operators across a diverse range of regional settings. Michael currently works as a Seismic Analyst with the Minerals, Energy and Groundwater Division of Geoscience Australia. |
![]() Wayne Cook is a Geophysical Project Officer at Geoscience Australia, with a B.Sc. in Resource and Environmental Management (the Australian National University; ANU) and a 30-year career in the earth science field. Wayne provides GIS and technical support to the geophysics program at Geoscience Australia. |
![]() Sam Voegeli is the Energy Services Lead at RESPEC with 13 years of experience focused on underground energy storage, particularly in salt caverns. His areas of focus include rock mechanics; thermodynamics; laboratory testing; and the design, construction and operation of underground storage facilities throughout the world. |
![]() Christopher J. Boreham is a Principal Organic Geochemist at Geoscience Australia, working in the Minerals, Energy and Groundwater Division. He obtained a PhD in Chemistry at ANU. Chris applies his skills to understand the evolution of petroleum and abiogenic gas in Australian basins. Chris is a member of PESA and the American Association of Petroleum Geologists. |
![]() Carmine Wainman holds an MSci in Geology from the University of Southampton and a PhD in Geosciences from the University of Adelaide. His professional expertise includes sedimentology, stratigraphy, palynology and basin analysis. He currently works at Geoscience Australia as a Basin Analyst in the Minerals, Energy and Groundwater Division. |
![]() Sebastian Wong is the Activity Leader of Geoscience Australia’s 3D Geoscience Integration Team, where he oversees the national-scale acquisition, integration and interpretation of 3D geological and geophysical data. Sebastian studied Geology at the University of Newcastle, and he has prior geoscience experience working for a state geological survey and in the minerals and energy industries. |
![]() Chris Southby is a Geoscientist in the Geoscience Australia’s Energy Systems Branch. He completed his Honours at ANU in 2004. Chris joined Geoscience Australia in 2008 and is now part of the Onshore Energy Systems team currently working under the Exploring for the Future program initiative. |
![]() Andrew Feitz is an Environmental Engineer and Director of Low Carbon Geoscience and Advice at Geoscience Australia. He holds a PhD from the University of New South Wales (UNSW) and worked as a Senior Researcher at UNSW and the Karlsruhe Institute of Technology (Germany). Andrew leads Geoscience Australia’s efforts in supporting the implementation of the National Hydrogen Strategy. |