Behavioural responses of Australian lizards towards visual cues of feral cats
Owen T. Lishmund

A
B
C Present address:
Abstract
Invasive mammalian predators have caused population declines and extinctions of wildlife worldwide. Many of these species exhibit some form of prey naïveté, which heightens their vulnerability to novel predators. In Australia, introduced feral cats (Felis catus) and red foxes (Vulpes vulpes) have had a particularly negative effect on native fauna, with the impacts of cats on mammals and birds well documented. Although feral cats are known to regularly prey on Australian reptiles, little is known about the behavioural responses of reptiles to cats, including whether native reptiles can recognise cats as a predation risk, and if so, which cues they use.
We investigated behavioural responses of two Australian lizard species, the shrubland morethia skink (Morethia obscura) and eastern striped skink (Ctenotus robustus), to the visual cues of feral cats in semiarid, south-eastern Australia.
We used arena trials to test lizards for predator recognition by using visual cues of an alien mammal predator (taxidermied cat, Felis catus), a native mammal predator (taxidermied western quoll, Dasyurus geoffroyi) and a mammal non-predator (taxidermied European rabbit, Oryctolagus cuniculus), as well as a procedural control (bucket) and a negative control (nothing).
We found little evidence of behavioural change when lizards were exposed to the taxidermied cat. Morethia obscura basked less when exposed to all treatments and C. robustus increased vigilance when in the presence of the taxidermied cat, but overall responses were similar among treatments.
Our findings suggest that stationary visual cues of cats do not trigger behavioural responses in these two lizard species.
Future research should assess behavioural responses to combinations of cat cues (e.g. movement, scent). Developing a deeper understanding of predator recognition systems and prey naïveté in reptile communities will be crucial for conservation of Australian reptiles that are negatively affected by feral cats.
Keywords: antipredator responses, behavioural ecology, Felis catus, feral cat, invasive predators, lizard, predator–prey, prey naïveté.
Introduction
Predators are drivers of evolution in prey species worldwide (Lima and Dill 1990; Nunes et al. 2014). While the most readily observed predator–prey mechanism is killing of prey animals by predators, fear alone has strong effects on prey behaviour, which flows through to foraging efficiency, habitat selection and many other aspects of the daily lives of animals (Vucetich et al. 2002; Creel and Christianson 2008; Choi and Kim 2010; Wang et al. 2016). Prey species have developed a wide range of adaptations to reduce their risk of predation, which are generally referred to as ‘antipredator responses’. These include behaviours that avoid capture and secondary defence mechanisms to reduce predation risk, including physiological, morphological and behavioural traits (Kavaliers and Choleris 2001; Creel 2018).
Effective antipredator responses rely on the capacity of prey to detect and appropriately respond to the threat of predation. Prey naïveté is the inability of prey species to recognise alien predators as a threat and to respond appropriately, owing to a lack of co-evolutionary history (Sih et al. 2010; Carthey and Banks 2014; Anton et al. 2020). Naïveté takes several forms, which Banks and Dickman (2007) defined according to the following three levels: Level 1 naïveté, when prey are simply unable to recognise predators and therefore show no response; Level 2 naïveté, when prey species can recognise threats posed by predators but display inappropriate antipredator responses to successfully reduce predation; and Level 3 naïveté, when prey species recognise predators, employ suitable antipredator responses for the type of predator, but are nonetheless out-manoeuvred by the predator. Prey naïveté is often invoked to explain the impact of invasive predators on native prey populations, particularly during initial phases of invasion (Schoener et al. 2001; Clavero and García-Berthou 2005). Indeed, invasive predators are implicated in more than 50% of modern bird, mammal and reptile extinctions (Doherty et al. 2016).
The domestic cat (Felis catus) was introduced into Australia in the late 18th century, and soon spread throughout the continent (Abbott 2008). The proliferation of feral cats in Australia, along with the introduction of the red fox (Vulpes vulpes), contributed towards numerous declines and extinctions of native wildlife (Burbidge and Manly 2002; Woinarski et al. 2015). Whereas the influence of feral cats on Australian mammals and birds is relatively well documented (see Woinarski et al. 2015, 2017), impacts on Australia’s reptiles are poorly understood. Approximately 466 million individual reptiles are estimated to be eaten by cats annually, comprising upwards of 263 species (Doherty et al. 2015; Woinarski et al. 2018; Chapple et al. 2019). Cats are considered a threatening process for at least 18 of the continent’s 60 most threatened reptile species, and for 5 of the 20 reptile species at highest risk of extinction (Geyle et al. 2021). Furthermore, exclusion of feral mammalian predators can increase reptile diversity, abundance, and survival rates (Lettink et al. 2010; but see Moseby et al. 2009; Roshier et al. 2020). It remains unclear whether reptile species co-existing with cats have developed antipredator responses, or whether certain levels of prey naïveté exist within populations.
Research into antipredator responses in Australian reptiles has produced varying results. A study of Murray River turtles (Emydura mcquarii) found that they did not alter nesting sites when exposed to olfactory cues of the red fox, but significantly altered them when presented with cues of a native but locally extinct predator, the spotted-tailed quoll (Dasyurus maculatus) (Spencer 2002). By contrast, Webster et al. (2018) demonstrated that two native lizard species, Boulenger’s skink (Morethia boulengeri) and southern marbled gecko (Christinus marmoratus), reduced their feeding activity when presented with olfactory cues of the red fox. Lizards can also respond to the visual cues of introduced predators. McCann’s skinks (Oligosoma mccanni) in New Zealand responded more strongly to the combination of visual (live caged cat) and olfactory cues of cats than to olfactory cues alone (Cliff et al. 2022).
Understanding whether native Australian reptiles recognise cats as predators and, if they do, which cues they use to detect them and how they respond is crucial for conservation of lizard species threatened by cats. The capacity to recognise slow-moving or stationary visual cues might be particularly important, given the hunting strategies of feral cats, which often engage in stalk and pounce behaviours to ambush their target prey (Moseby and McGregor 2022). Although olfactory and auditory cues may also be important, either alone or in combination, testing for recognition of individual sensory cues (in this case visual cues) is an important first step in assessing antipredator responses towards cats. Such knowledge could then inform the development of novel conservation strategies aimed at reducing cat predation on lizards, for instance, by selectively exposing them to predators or cues to facilitate the development of learned antipredator responses. Training lizards to display antipredator behaviour towards visual cat cues has been used for captive El Hierro giant lizards (Gallotia simonyi), which provides a proof of concept for trialling such measures for other reptiles (Burunat-Pérez et al. 2018).
The aim of this study was to investigate predator recognition behaviours in two Australian lizards, the shrubland morethia skink (Morethia obscura) and the eastern striped skink (Ctenotus robustus) in a semi-arid region of western Victoria, Australia. The specific objectives of this study were to (i) determine whether native lizards can recognise feral cats as predators by using stationary visual cues, and (ii) quantify differences in lizard behaviour in the presence of cat cues relative to a native predator (quoll) and appropriate controls, namely, non-predator (rabbit), procedural control (bucket) and negative control (nothing). We hypothesised that if native lizards are unable to recognise feral cats as a threat, they will display more cautious behaviours (e.g. sheltering) and fewer risky behaviours (e.g. basking and exploring) when presented with visual cues of a native predator than of a feral cat. If lizards fail to identify feral cats as a threat, they will display similar behaviours for feral cat cues and non-predator cues. Conversely, if lizards can recognise feral cats as a predation threat, they will display increased caution and vigilance towards both feral cat and native predator cues relative to non-predator cues and controls.
Materials and methods
Study area
This study was conducted in the Wimmera region of Victoria near Little Desert National Park (Fig. 1). The Wimmera experiences a semiarid climate and mean annual rainfall of 440 mm year−1 (Bureau of Meteorology 2022a). Fieldwork was conducted during the austral spring and summer (September 2022–January 2023) to target peak reptile activity (Spence-Bailey et al. 2010). However, this period coincided with concurrent La Niña and negative Indian Ocean Dipole events, which produced significantly cooler and wetter conditions than the long-term averages and reduced reptile activity (Bureau of Meteorology 2022b).
Map of study area, arena location and trapping sites, with location in western Victoria shown (top right). Satellite imagery shows areas of native vegetation (darker green). Map data ©2023 Google, Imagery ©2023 Airbus.
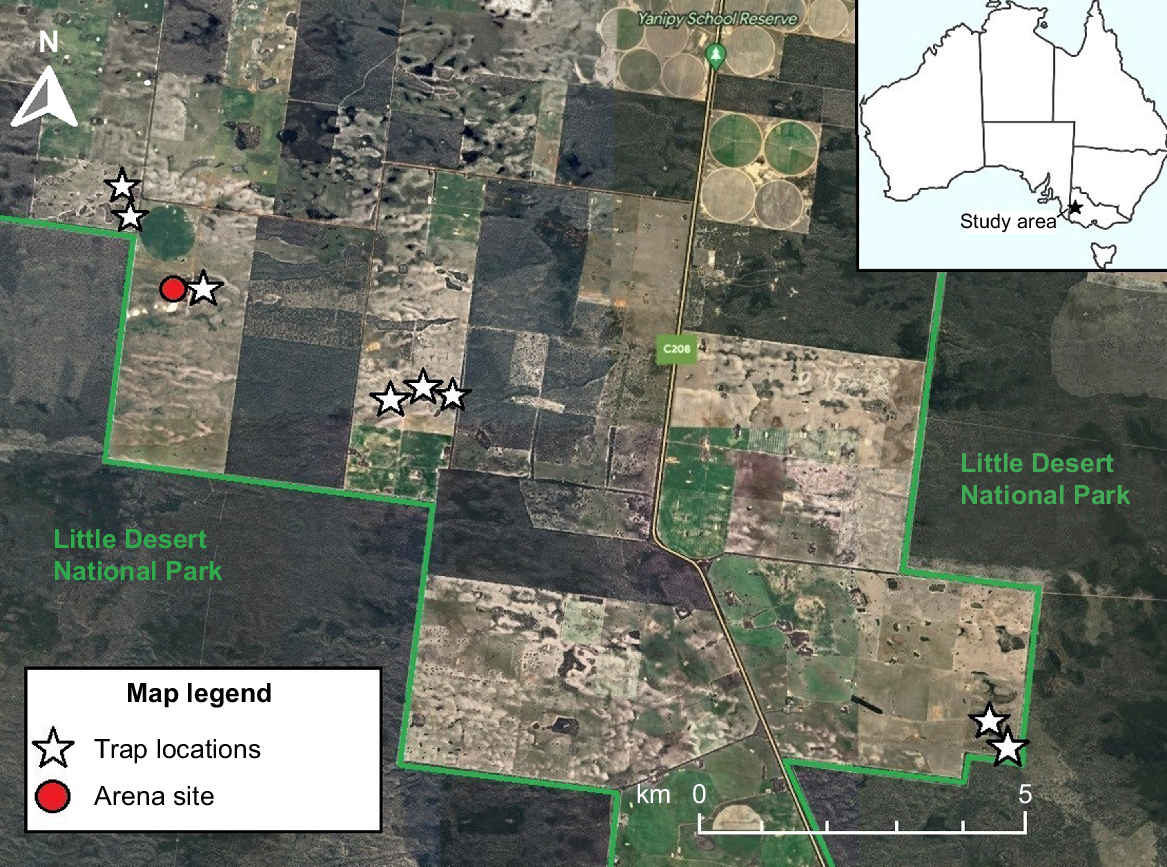
The study area contains remnant patches of woodland surrounded by agricultural land largely used for sheep grazing and dryland cropping and is north of Little Desert National Park (Fig. 1; Gardner et al. 1992). The remnant patches consist of heathy woodlands where the dominant canopy species are desert stringybark (Eucalytpus arenacea) in the undulating sandy dunes and yellow gum (Eucalyptus leucoxylon) in the low-lying clay-based areas. The area has ~28 reptile species recorded (Robertson and Coventry 2019). Feral cats were present in the study area, although we did not quantify their activity.
Study species and capture methods
This study used two skink species: the shrubland morethia skink (Morethia obscura) and the eastern striped skink (Ctenotus robustus) (Fig. 2). Morethia obscura is a small skink (mean snout–vent length = 51 mm), common across much of southern Australia in woodlands and heath on lighter soils, and is a terrestrial heliotherm that feeds on small invertebrates (Robertson and Coventry 2019). Ctenotus robustus is a medium-sized skink (110 mm), which is common and widespread throughout much of eastern Australia. It uses a variety of habitats and is also a terrestrial heliotherm that feeds on invertebrates and plant materials (Robertson and Coventry 2019). These two species were selected because of their abundance within the study area and because they are known prey species of feral cats (Read and Bowen 2001; Stobo-Wilson et al. 2021).
Depiction of lizard species and arena trial set-up used in our study including: (a) shrubland morethia skink; (b) eastern striped skink; (c) model taxidermied cat; (d) model taxidermied rabbit; (e) model taxidermied western quoll; (f) plastic bucket; (g) arena set-up showing basking platform and hide cave on each side, corflute dividing wall with radiation shield containing temperature logger at its near end, and (h) full arrangement of the five arenas including frames with mounted cameras, each containing one of the four treatment objects outlined, with the fifth containing no treatment. Note that photo h was taken before plastic lining was applied to arena walls. Photographs supplied by Owen Lishmund.
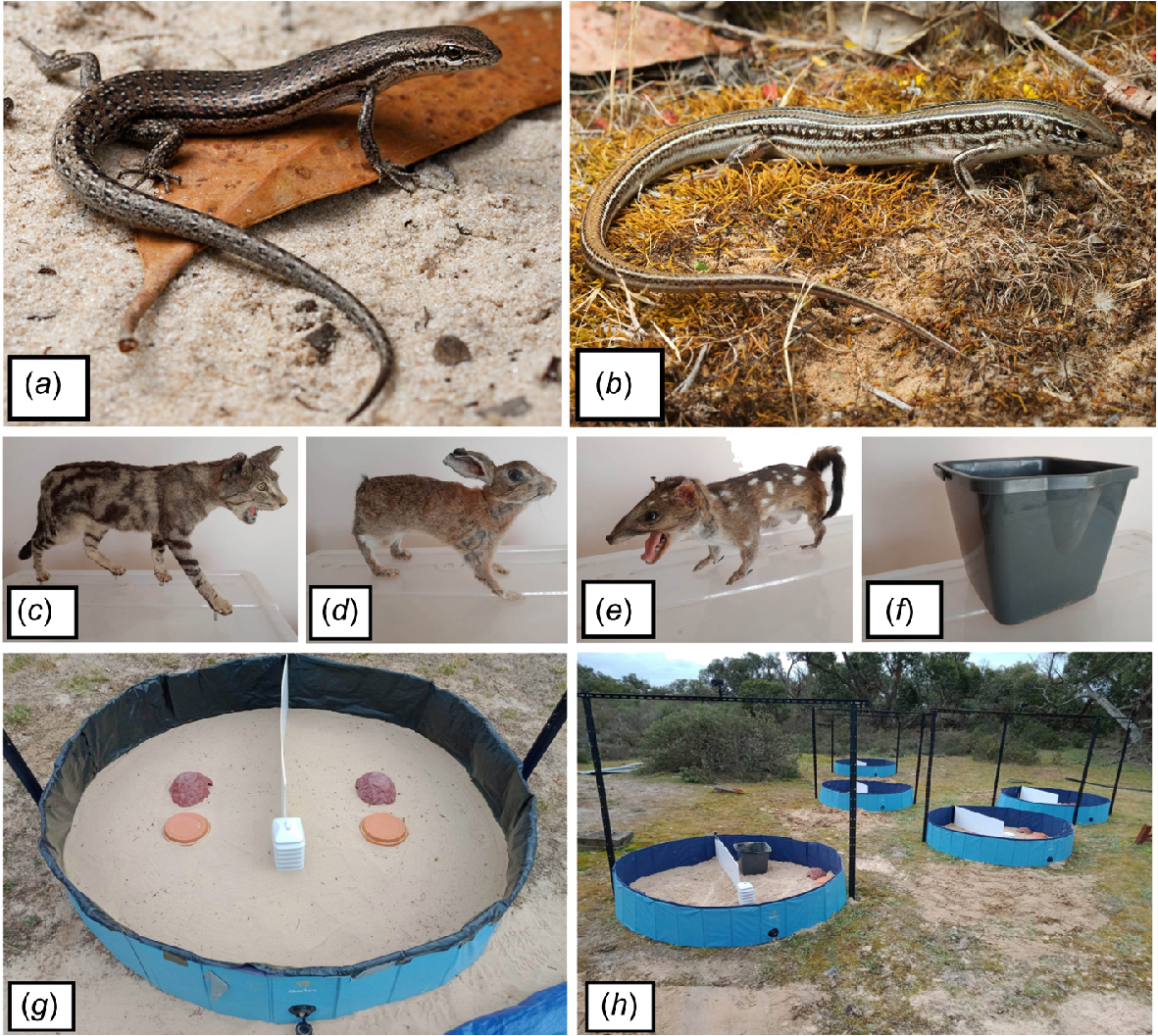
Lizards were predominantly captured using pitfall and funnel traps located in remnant patches. Each trap line consisted of three 20-L buckets and two funnel traps with a 30 cm high and 30 m long drift fence running through these traps. On either side of each trap line, there were two artificial refuge stations, each containing two stacked 1-m2 corrugated tin sheets and two terracotta roof tiles. In total, 34 trap lines were used and these were spread across eight remnant habitat patches of varying size. Lizards were also captured by opportunistically searching underneath pieces of abandoned building materials and woody debris. Lizards were individually placed in small plastic tubs lined with sand, and holes were punched in the lids for air. Tubs were placed inside a portable chest cooler and transported to the arena site for trials. Lizards were not used for trials if they were juvenile, gravid or had recently autotomised tails.
Arena trials
We used five experimental arenas, each with a different treatment condition. These treatments were (i) a taxidermied feral cat, (ii) a taxidermied western quoll (Dasyurus geoffroii), (iii) a taxidermied European rabbit (Oryctolagus cuniculus), (iv) a bucket, and (v) nothing (Fig. 2). The arena trials took place between September 2022 and February 2023, with 60 M. obscura sampled across the cat (n = 13), quoll (n = 10), rabbit (n = 12), bucket (n = 13), and nothing (n = 12) treatments, and 100 C. robustus sampled across the cat (n = 22), quoll (n = 15), rabbit (n = 22), bucket (n = 21), and nothing (n = 21) treatments. The cat and quoll models were taxidermied in a threatening pose with their jaws agape because evidence exists that Australian reptile species can recognise shapes of predators, demonstrated by Carlile et al. (2006) in their study with Jacky dragons (Amphibolurus muricatus). Further, cats are often stationary during ambush hunting, and hence, the use of a stationary model represents a phase of the feral cat predation sequence.
The quoll was included to assess whether lizards show differential responses when exposed to a native predator with which they have a long evolutionary history (the quoll), or an invasive predator with which they have a short evolutionary history (the cat). The eastern quoll (Dasyurus viverrinus) is thought to have occurred across much of Victoria’s Wimmera region up until the early 1900s, so we considered this model to represent a predator archetype with which both M. obscura and C. robustus should have shared eco-evolutionary experience (Peacock and Abbott 2014; Wallis 2021). The quoll represents a valid comparison with the cat because both species are primarily nocturnal (McGregor et al. 2015; Linley et al. 2021). We were unable to obtain a taxidermied eastern quoll, but the western quoll is very similar in size and appearance. There are no extant medium-sized native mammalian predators of lizards in this system and the quoll represents one of the most recently occurring species. Although largely nocturnal, quolls have flexible diets and depredate on a range of diurnal animals, including skink species (Dunlop et al. 2017). Previous research has shown that prey species may recognise extinct native predators after lengthy periods of absence, including Australian reptiles altering behaviour patterns in the presence of sensory cues for locally extinct quoll species (Spencer 2002; Blumstein et al. 2009; Dalerum and Belton 2015; Webster et al. 2018). The quoll could be obtained only after sampling begun, which resulted in slightly fewer animals being tested against it than in other treatments. The inclusion of a herbivore, the European rabbit, was intended to ascertain whether skinks are exhibiting a generalised response to mammalian models. Rabbits are common throughout the study area and have been present in southern Australia since the mid-1800s (Zenger et al. 2003). The bucket was included as a procedural control to determine whether lizards are displaying a generalised response to the presence of a novel object, whereas the arena containing nothing as a treatment was included as a negative control.
Five identical portable pet paddling pools (1.6 m in diameter, 40 cm high) were used as experimental arenas to conduct trials using the captured lizards. The arenas were established in a flat, cleared area and remained in situ for the entire field season (Fig. 1). The bases of the arenas were lined with a thin layer of sand to simulate a natural substrate. The walls of each arena were lined with plastic pond-lining sheet affixed with tape to prevent lizards from escaping. The pools contained a drainage hole on one side that were used to introduce lizards into the arena. A corflute dividing wall spanning 1.1 m was secured across the centre of arenas, leaving a gap of ~50 cm, creating two separate compartments which lizards could freely move between (Fig. 2). The dividing walls were attached to the arena walls with tape on the opposite side to the drainage hole, presenting lizards with a choice between compartments. Inside each arena, one compartment contained a treatment condition (cat, quoll, rabbit, bucket, or nothing), whereas the other contained no treatment (Fig. 2). In each compartment, a terracotta basking plate (15 cm in diameter) and a reptile hide cave (20 cm in length) were placed in a central location (Fig. 2). The arenas were angled so that both compartments received similar amounts of sunlight, and to reduce the effects of shadows. Small action cameras (Akaso Brave 7 LE) were mounted in the centre ~1.6 m above the arena floors and pointing downwards (Fig. 2). Temperature loggers (HOBO MX2202 Pendant) were placed inside each arena under a small radiation shield (Fig. 2), recording ambient air temperature once per minute during each trial.
Each trial was conducted over 1 h during the middle of the day, during dry and calm conditions. Wind did not cause the sides of the arenas to move or make noise. Trials were filmed at 2.7k resolution and 30 frames per second, with cameras capturing the entire floor area from a birds’-eye view. Lizards were introduced to the arenas at the beginning of this period by releasing them through the drainage hole of the paddling pool and then tightening the cap. Once the 60-min filming period had finished, lizards were recaptured, processed (described below), and released at their point of capture. Individual lizards were used for one trial only and all animals were marked to avoid re-testing them if recaptured. The treatments were continually rotated around the arenas on a schedule and swapped to the opposite sides of the arenas after each trial to minimise potential selection biases (i.e. the taxidermied models and the bucket were switched between the left and right side of the arenas and between arenas each trial). Lizards were also randomly allocated to arenas before commencing their trials. We did not use an acclimatisation period for the skinks because we were unable to place the taxidermied animals (or bucket) in the arenas without being seen by the skinks. The sudden presence of a human standing over the arena may itself have triggered anti-predator behaviours and thus confounded the results. Although the behaviours we recorded may have been a response to the animals being placed in a novel environment, it is important to note that any such effect would have applied equally across treatments.
After the trials, lizards were processed to record morphometric data (snout–vent length and weight) and tagged for identification. Lizards were given a generic marking by using visual implant elastomer (VIE), which was injected using a small syringe inserted just below the skin surface on the ventral side adjacent to the forelimbs. Sampling data were recorded in custom mobile apps created in Fulcrum.
Video processing
Videos of arena trials were downloaded and copied onto The University of Sydney data server. We used BORIS (ver. 8.9.4, see https://www.boris.unito.it/), an open-source, event-logging software to score each video (Friard and Gamba 2016). One observer scored the first 30 min of every video. Because of time constraints, the second 30 min of each trial was not scored, although full videos have been retained for future analysis. A behavioural ethogram was created with behaviour categories, which was used to score the duration of lizard behaviours for the 30 min, whereas the compartment of the arena (treatment or non-treatment) was specified for all scored behavioural events. If the compartment could not be confidently determined for an individual behavioural event, it was scored as ‘neither’. Start and stop times for each behavioural event were recorded.
The behaviours in the ethogram were as follows:
bask – lizard clearly visible atop either the terracotta saucers or hide caves and not actively moving;
explore – lizard is clearly seen to be actively moving within the arena;
attempted escape – lizard attempts to escape the arena by either trying to climb up or jumping against the walls;
motionless – lizard is seen to remain in the same place and not actively moving for a duration of at least 5 s, but not on a terracotta saucer or hide cave;
shelter – lizard is fully concealed underneath the hide cave with no part of the animal visible to the camera;
partially emerged – lizard is sheltering inside the hide cave but part of the animal’s head, or the head and some of the body is visible outside the entrance (also referred to as ‘leaning out’);
bury – lizard buries itself in or attempts to dig down into the substrate on the arena floor (this behaviour was only observed for M. obscura);
hidden – lizard is not visible to the camera and is also known to not be inside either of the hide caves;
obscured by treatment – lizard is not visible, owing to the animal being completely obscured from view by the treatment object.
Statistical analysis
We used generalised linear mixed-effects models to test whether the proportion of time lizards spent performing each behaviour varied among the treatments (cat, quoll, rabbit, bucket, nothing), and between the compartments of the arena they were in (treatment or non-treatment side). The total time was calculated in seconds for each behavioural category in each compartment, then converted into proportions of total trial time. Behavioural events coded as ‘neither’ for compartment were not used in the analyses, but this time was still included in the total trial times for the above calculations. Statistical analyses were conducted using the statistical computing software R (ver. 4.2.2, R Foundation for Statistical Computing, Vienna, Austria, see https://www.r-project.org/). We used the R package glmmTMB (ver. 1.1.3, see https://github.com/glmmTMB/glmmTMB) to perform zero-inflated beta regression (see Brooks et al. 2017), with a logit-link function, which is appropriate for proportional data bounded by zero and one (Ferrari and Cribari-Neto 2004; Douma and Weedon 2019). Models were created for every behavioural category for each species (including ‘shelter total’, which represents the combination ‘shelter’ and ‘partially emerged’ because these behaviours were very similar and combining them aided model convergence when modelling behaviours for Morethia obscura). All models included fixed effects of treatment, compartment, and their interaction, as well as random effects of trial identity nested with trial date to account for repeated sampling within trials (i.e. the two compartments) and on individual days (i.e. different arenas and trials).
‘Nothing’ was used as the reference level for treatments and ‘non-treatment’ was used as the reference level for compartment. Therefore, when interpreting results for treatment, parameter estimates for each treatment are relative to that observed for the ‘nothing’ treatment, and for compartment effects, parameter estimates represent the proportion of time spent in the treatment compartment relative to the non-treatment compartment. The fixed-effects component of the model for basking in C. robustus did not converge when trial date was included as a random effect; so, the date was excluded and the model was refitted. This is likely to have had no impact on results because the trial date accounted for zero variance in the original model. We ensured that models were an appropriate fit for the data by inspecting residual plots created using the DHARMa package (ver. 0.4.6, F. Hartig, see https://cran.r-project.org/package=DHARMa) in R.
All models also included a fixed effect of mean temperature (°C) during each trial, to account for any possible temperature effects on lizard behaviour. There were 10 trials where temperature data were not recorded or stored correctly. For these trials, we used a statistical model to estimate the relationship between logger temperatures and air temperatures that were concurrently measured every minute at the nearest Bureau of Meteorology (BOM) weather station (Nhill, ~60 km away). Using the loggers that functioned correctly, we matched each logger measurement to the closest BOM measurement based on times and fitted a general linear model that predicted logger measurement as a function of BOM temperature (Pearson’s r = 0.79). We then calculated the mean BOM temperature for the trials with missing data and predicted the logger temperature measurements by using the relationship from this statistical model.
Results
A total of 160 arena trials took place in this study; 60 for M. obscura and 100 for C. robustus. Lizards spent the greatest amount of time being motionless (39.6% for M. obscura and 48.3% for C. robustus) and exploring (17.7% for M. obscura and 29.3% for C. robustus). Lizards spent less time basking (8.6% for M. obscura and 5.5% for C. robustus). Sheltering behaviours (including ‘shelter’ and ‘partially emerged’) comprised only 3.8% of time for M. obscura, but 10.3% for C. robustus. Attempted escape took up a miniscule proportion of time (≤2% for both species). Burying was observed only for M. obscura and comprised 12.3% of their total trial time. The behaviour categories ‘hidden’ and ‘obscured by treatment’ were rare for both species and were excluded from the models.
In summary, lizards spent large amounts of the total trial time displaying behaviours that may be considered as anti-predatory (sheltering, partially emerged, attempted escape, motionless, burying), totalling 56.7% for M. obscura and 60.1% for C. robustus. Meanwhile, time spent displaying behaviours that may be considered as bold (basking, exploring) was lower, at 26.3% for M. obscura and 34.8% for C. robustus respectively.
Morethia obscura
The models for each behaviour category showed support for differences in behaviours among the treatments and between compartments for five tests (Table 1). Morethia obscura spent less time basking in the presence of the bucket (β = −1.64, 95% CI [−2.68, −0.6]), rabbit (β = −1.25, 95% CI [−2.16, −0.35]), and the cat (β = −1.86, 95% CI [−2.96, −0.77]) relative to nothing (Table 1, Fig. 3). A similar pattern was observed for the quoll but the confidence intervals overlapped zero slightly. The interaction between treatment and compartment was statistically significant for attempted escape and the rabbit treatment, indicating less time spent attempting escape in the treatment compartment of the rabbit arena than in the nothing arena (β = −1.12, 95% CI [−2.23, −0.01]). Morethia obscura spent less time buried in the treatment compartment than in non-treatment in the presence of the bucket, rabbit and quoll (Table 1, Fig. 3), with a strong effect for the latter (β = −3.61, 95% CI [−7.19, −0.03]). They spent significantly more time buried in the non-treatment compartment for the quoll treatment, with a significant interaction (β = −3.61, 95% CI [−7.19, −0.03]) between treatment and compartment (Table 1, Fig. 3). There was a decrease in basking behaviour for M. obscura as mean ambient temperature increased, although confidence intervals slightly overlapped zero (Table 1, Fig. 4). Otherwise, ambient temperature did not have a significant effect on other behaviours displayed by this species.
Predictor variable | Attempted escape β (95% CI) | Bask β (95% CI) | Bury β (95% CI) | Explore β (95% CI) | Motionless β (95% CI) | Shelter (total) β (95% CI) | |
---|---|---|---|---|---|---|---|
Intercept | −5.73 (−7.02 to −4.44) | 1.79 (−1.55 to 5.12) | −1.71 (−5.49 to 2.06) | −1.71 (−2.70 to −0.71) | −1.24 (−2.60 to 0.13) | −0.17 (−3.03 to 2.69) | |
Treatment | |||||||
Bucket | 0.17 (−0.69 to 1.03) | −1.64 (−2.68 to −0.60) | 1.89 (−0.76 to 4.54) | −0.32 (−0.99 to 0.34) | −0.22 (−1.05 to 0.60) | 0.87 (−1.05 to 2.79) | |
Rabbit | 0.49 (−0.32 to 1.30) | −1.25 (−2.16 to −0.35) | 1.99 (−1.06 to 5.04) | −0.08 (−0.69 to 0.52) | −0.31 (−1.07 to 0.45) | −0.49 (−2.09 to 1.12) | |
Quoll | 0.06 (−0.76 to 0.88) | −1.03 (−2.13 to 0.08) | 1.87 (−0.69 to 4.44) | −0.21 (−0.85 to 0.42) | −0.17 (−0.97 to 0.62) | 0.35 (−1.18 to 1.88) | |
Cat | 0.53 (−0.28 to 1.33) | −1.86 (−2.96 to −0.77) | 1.55 (−1.05 to 4.14) | −0.20 (−0.82 to 0.43) | −0.07 (−0.88 to 0.74) | 0.88 (−0.81 to 2.57) | |
Compartment | 0.69 (−0.07 to 1.45) | −0.53 (−1.42 to 0.36) | 1.58 (−0.90 to 4.05) | −0.67 (−1.36 to 0.03) | −0.32 (−1.18 to 0.55) | 0.72 (−1.01 to 2.44) | |
Temperature | −0.01 (−0.06 to 0.04) | −0.12 (−0.25 to 0.01) | −0.01 (−0.16 to 0.14) | −0.01 (−0.05 to 0.03) | 0.00 (−0.05 to 0.05) | −0.04 (−0.15 to 0.08) | |
Treatment × compartment | |||||||
Bucket | −0.97 (−2.21 to 0.28) | 0.91 (−0.51 to 2.33) | −2.47 (−5.78 to 0.84) | 0.80 (−0.12 to 1.73) | 0.42 (−0.76 to 1.60) | −1.30 (−3.88 to 1.28) | |
Rabbit | −1.12 (−2.23 to −0.01) | 0.58 (−0.45 to 1.61) | −3.04 (−6.70 to 0.61) | 0.33 (−0.57 to 1.23) | 0.87 (−0.28 to 2.01) | −0.84 (−3.36 to 1.68) | |
Quoll | 0.27 (−0.82 to 1.36) | −1.01 (−2.30 to 0.27) | −3.61 (−7.19 to −0.03) | 0.92 (−0.05 to 1.88) | 0.59 (−0.63 to 1.80) | −1.45 (−4.32 to 1.42) | |
Cat | −0.66 (−1.72 to 0.40) | 0.62 (−0.78 to 2.01) | −0.05 (−3.65 to 3.55) | 0.39 (−0.57 to 1.35) | −0.06 (−1.25 to 1.13) | −0.52 (−2.95 to 1.92) |
Results of mixed effects models testing the effects of treatment, compartment, treatment × compartment, and temperature on behaviours of M. obscura. Values represent parameter estimates and 95% confidence intervals (CI). Values in bold represent parameters where the confidence intervals do not overlap zero. The reference levels for treatment and compartment were ‘nothing’ and ‘non-treatment’ respectively.
Modelled proportions of time (of total trial time) that M. obscura spent engaging in each behaviour for the five treatment conditions, with a direct comparison of treatment and non-treatment compartments (zone). Points and triangles represent estimated means and whiskers represent the 95% confidence intervals. Note that scales vary for the y-axes.
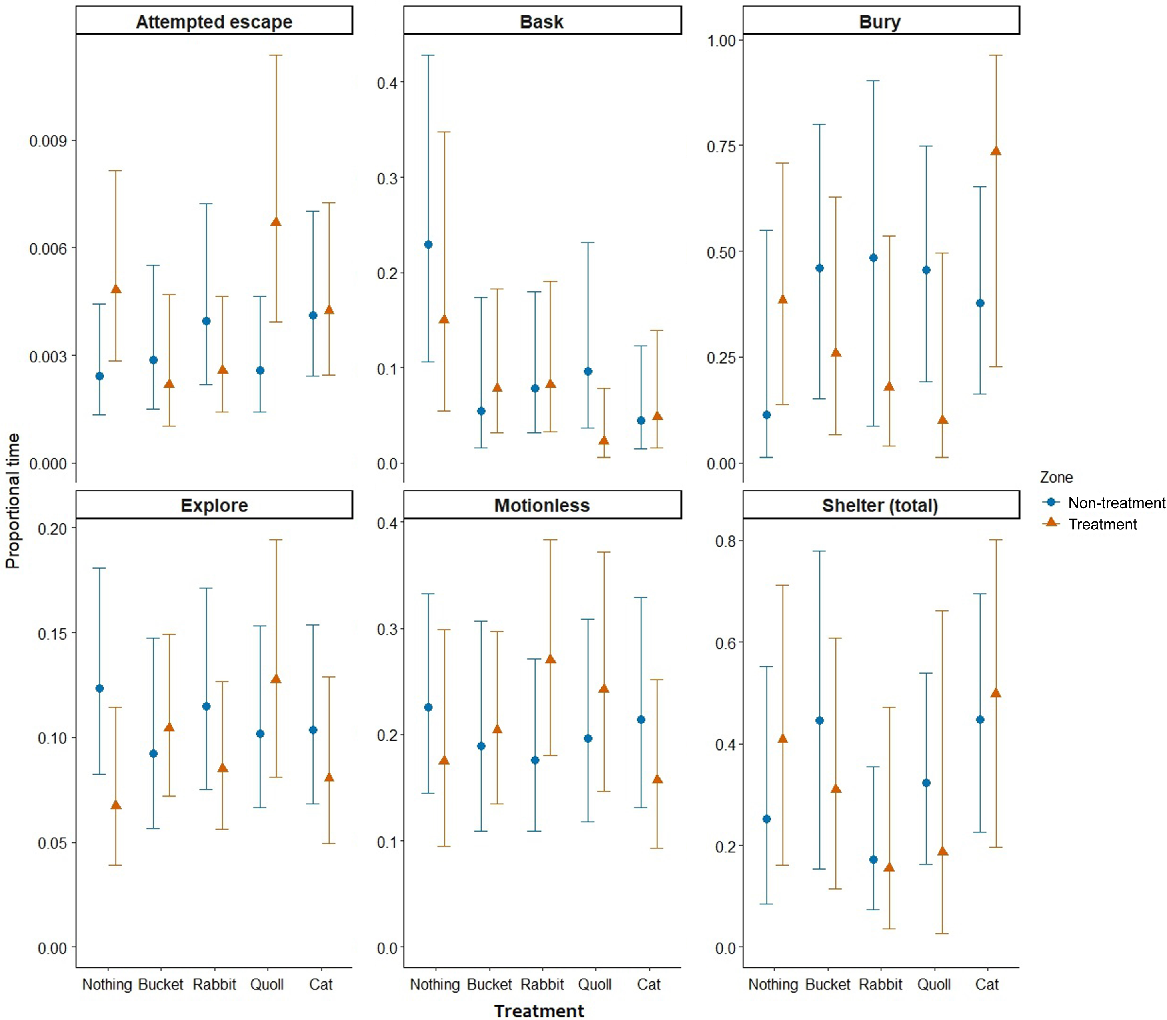
Modelled effect of average ambient air temperature (°C) during arena trials for M. obscura on predicted proportion of time (of total trial time) lizards spent engaging in each behaviour. Trend lines display estimated mean proportion of time across continuous temperature values and shaded areas depict the 95% confidence intervals. Note that scales vary for the y-axes.
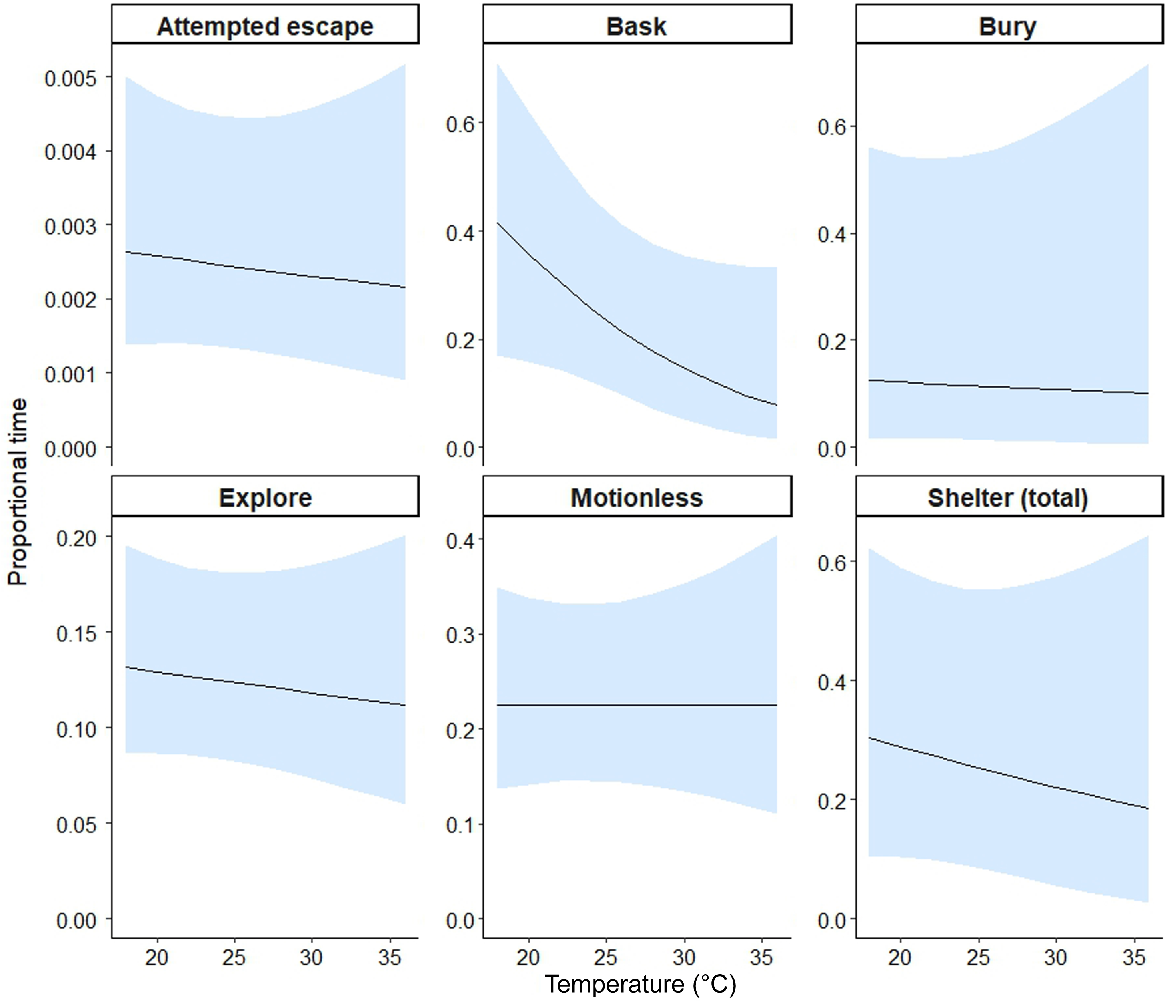
Ctenotus robustus
The models for each behaviour category showed support for differences in behaviours between the treatments and compartments for three tests (Table 2). Ctenotus robustus spent more time partially emerged in the treatment compartment of the cat arena than in the non-treatment compartment, whereas there was no difference between compartments when the treatment was nothing (β = 2.45, 95% CI [1.23, 3.67]) (Table 2, Fig. 5). Ctenotus robustus spent the most time exploring in the arena containing the rabbit, and the least time exploring in the arena containing the quoll, but these differences were not statistically different across the treatments (Table 2, Fig. 5). Otherwise, behaviours for this species largely showed similarity for the remaining behaviour categories across the treatment conditions and compartments when compared with the control treatment (nothing) (Table 2, Fig. 5). There was a statistically significant effect of ambient temperature on basking behaviour of C. robustus, with time spent basking decreasing as temperature increased (β = −0.09, 95% CI [−0.15, −0.03]) (Table 2, Fig. 6). Proportion of time spent sheltering and partially emerged also decreased with an increasing temperature, whereas exploring increased, but these confidence intervals slightly overlapped zero.
Predictor variable | Attempted escape β (95% CI) | Bask β (95% CI) | Explore β (95% CI) | Motionless β (95% CI) | Partially emerged β (95% CI) | Shelter (separate) β (95% CI) | Shelter (total) β (95% CI) | |
---|---|---|---|---|---|---|---|---|
Intercept | −4.22 (−5.04 to −3.40) | −0.48 (−2.00 to 1.03) | −2.50 (−3.31 to −1.69) | −1.20 (−2.10 to −0.30) | −1.84 (−4.10 to 0.42) | −1.24 (−2.93 to 0.44) | −0.84 (−2.53 to 0.85) | |
Treatment | ||||||||
Bucket | −0.05 (−0.55 to 0.45) | 0.29 (−0.60 to 1.18) | −0.05 (−0.48 to 0.39) | 0.21 (−0.35 to 0.78) | −0.42 (−1.67 to 0.82) | 0.07 (−0.96 to 1.10) | 0.03 (−1.00 to 1.05) | |
Rabbit | −0.13 (−0.63 to 0.37) | 0.74 (−0.15 to 1.62) | 0.26 (−0.16 to 0.69) | −0.14 (−0.72 to 0.44) | 0.18 (−0.90 to 1.25) | 0.05 (−0.89 to 1.00) | 0.05 (−0.89 to 0.99) | |
Quoll | −0.21 (−0.76 to 0.35) | 0.14 (−0.83 to 1.12) | −0.17 (−0.66 to 0.31) | 0.06 (−0.56 to 0.67) | −0.88 (−2.15 to 0.40) | 0.25 (−0.79 to 1.29) | 0.15 (−0.88 to 1.19) | |
Cat | −0.15 (−0.65 to 0.34) | 0.51 (−0.37 to 1.39) | −0.05 (−0.49 to 0.38) | 0.09 (−0.48 to 0.67) | −1.51 (−2.63 to −0.38) | −0.08 (−0.97 to 0.81) | −0.22 (−1.11 to 0.67) | |
Compartment | −0.14 (−0.62 to 0.34) | 0.49 (−0.28 to 1.26) | 0.21 (−0.20 to 0.63) | −0.08 (−0.65 to 0.50) | −0.60 (−1.28 to 0.09) | −0.29 (−1.23 to 0.66) | −0.34 (−1.28 to 0.60) | |
Temperature | −0.00 (−0.03 to 0.03) | −0.09 (−0.15 to −0.03) | 0.03 (−0.00 to 0.06) | 0.00 (−0.03 to 0.03) | −0.07 (−0.16 to 0.02) | −0.03 (−0.10 to 0.03) | −0.04 (−0.11 to 0.03) | |
Treatment × compartment | ||||||||
Bucket | −0.10 (−0.77 to 0.57) | −0.62 (−1.80 to 0.55) | −0.33 (−0.93 to 0.27) | −0.15 (−0.95 to 0.64) | 0.55 (−0.52 to 1.62) | 0.32 (−1.11 to 1.76) | 0.40 (−1.03 to 1.83) | |
Rabbit | −0.04 (−0.71 to 0.64) | −1.08 (−2.32 to 0.15) | −0.25 (−0.83 to 0.32) | 0.17 (−0.63 to 0.97) | 0.50 (−0.42 to 1.42) | 0.30 (−1.02 to 1.62) | 0.35 (−0.96 to 1.67) | |
Quoll | −0.15 (−0.94 to 0.63) | −0.55 (−1.91 to 0.81) | −0.27 (−0.94 to 0.41) | 0.22 (−0.64 to 1.09) | 1.25 (−0.17 to 2.68) | 0.62 (−0.94 to 2.18) | 0.68 (−0.88 to 2.24) | |
Cat | −0.03 (−0.70 to 0.64) | −0.48 (−1.69 to 0.74) | −0.23 (−0.82 to 0.35) | 0.09 (−0.71 to 0.88) | 2.45 (1.23 to 3.67) | 0.71 (−0.68 to 2.09) | 0.91 (−0.47 to 2.29) |
Results of mixed-effects models testing the effects of treatment, compartment, treatment × compartment, and temperature on behaviours of C. robustus. Values represent parameter estimates and 95% confidence intervals (CI). Values with bold text represent parameters where the confidence intervals do not overlap zero. The reference levels for treatment and compartment were ‘nothing’ and ‘non-treatment’ respectively.
Modelled proportions of time (of total trial time) that C. robustus spent engaging in each behaviour for the five treatment conditions, with a direct comparison of treatment and non-treatment compartments (zone). Points and triangles represent estimated means and whiskers represent the 95% confidence intervals. Note that scales vary for the y-axes.
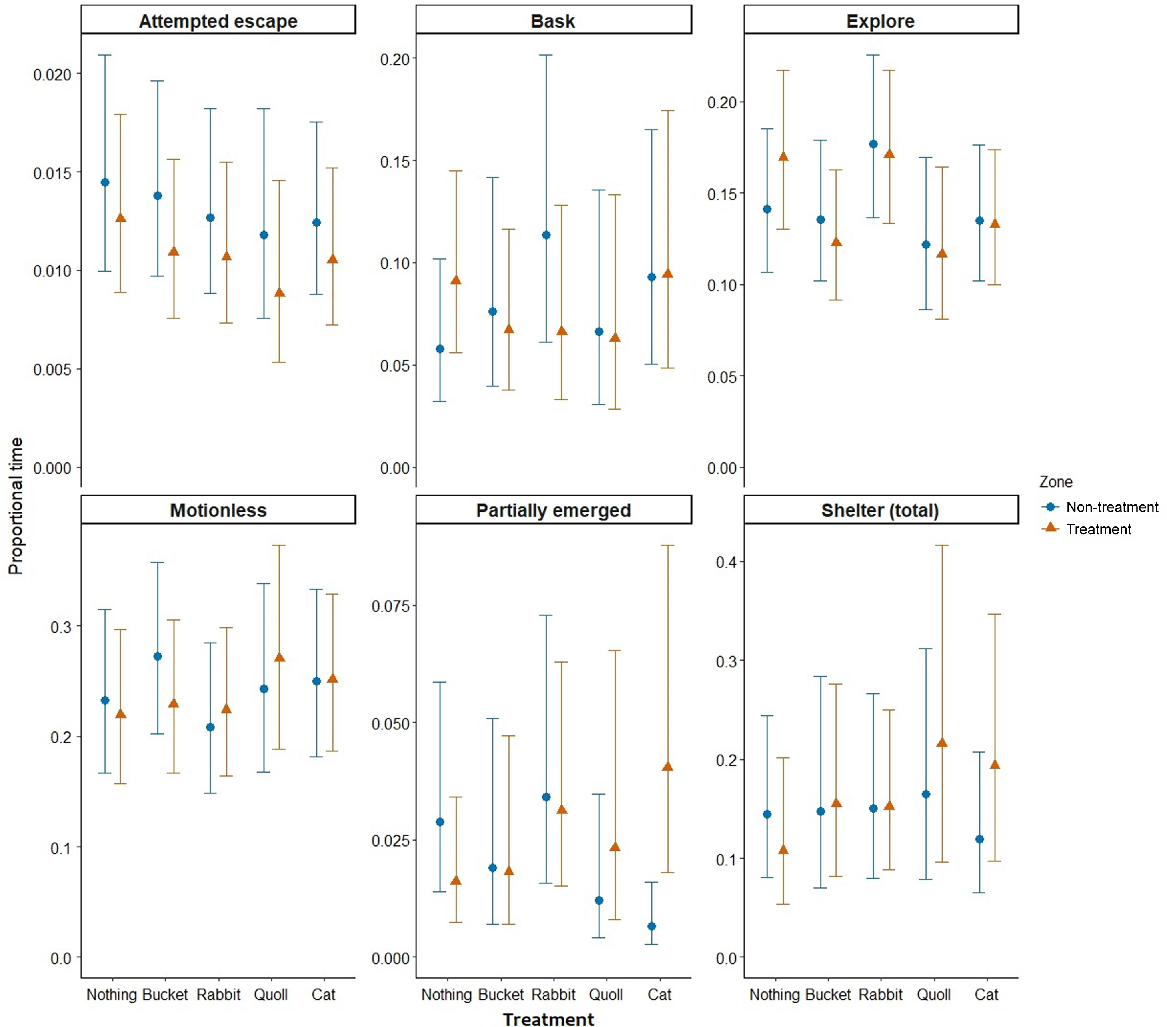
Modelled effect of average ambient air temperature (°C) during arena trials for C. robustus on predicted proportion of time (of total trial time) lizards spent engaging in each behaviour. Trend lines display estimated mean proportion of time across continuous temperature values and shaded areas depict the 95% confidence intervals. Note that scales vary for the y-axes.
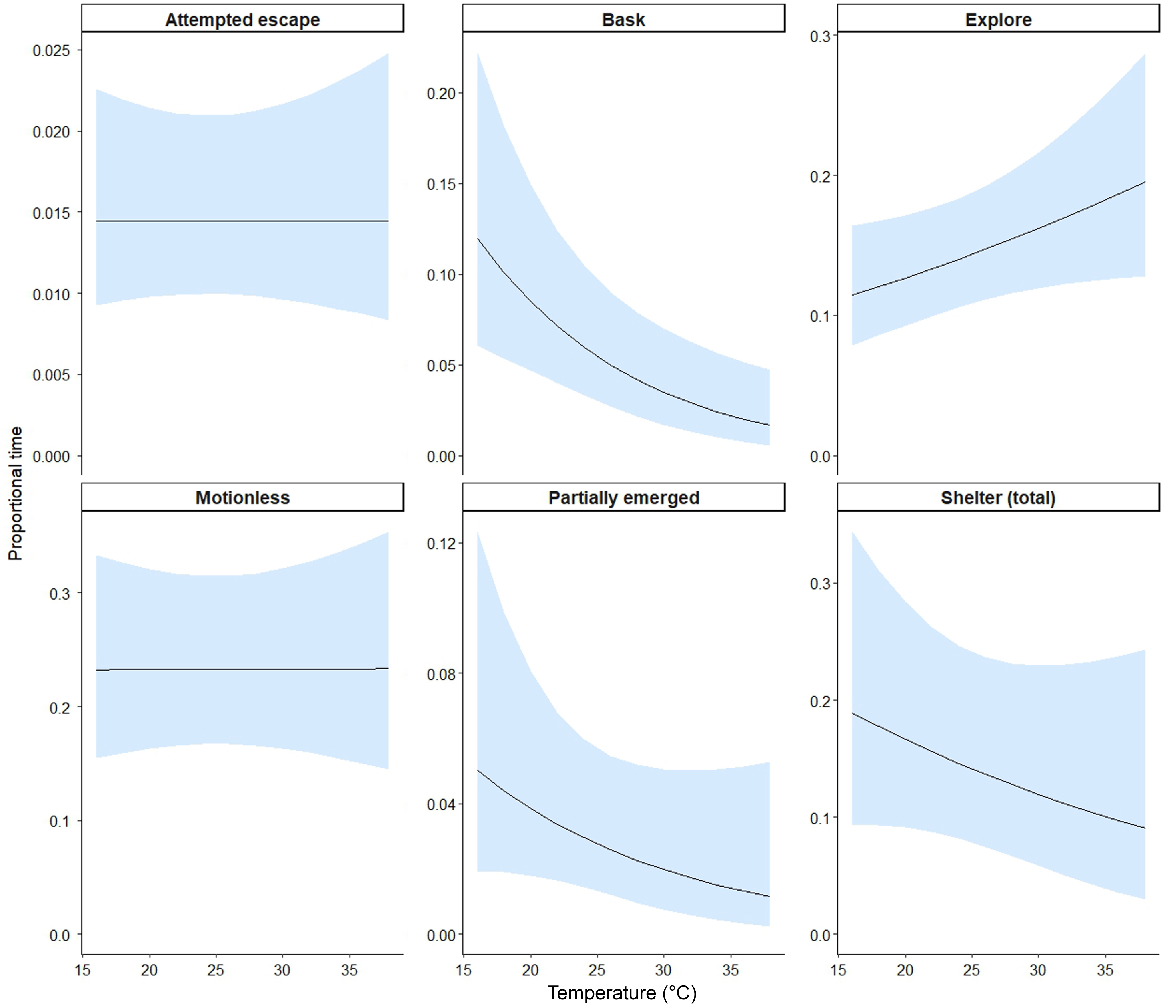
Discussion
We used arena trials to test the behavioural responses of native lizards towards the visual cues of feral cats. Our findings largely support the hypothesis that lizards fail to recognise feral cats as predators by using stationary visual cues because their behaviours were similar for cat and non-predator cues. The responses measured also suggest that lizards fail to recognise native quolls as predators. There is little evidence to support the hypothesis that lizards recognise cats as a predation threat and behave more cautiously towards their cues than towards non-predator cues. The overall lack of responses to both feral (cat) and native but locally extinct (quoll) predator models suggest an inability to recognise mammalian predators by using stationary visual cues. This could be driven by the hunting strategies of the lizards’ current native avian and reptilian predators contrasting those of mammalian predators. The lack of behavioural responses to stationary predator models may increase their vulnerability to predators that hunt by stealth and ambush. We discuss these results in the context of previous research before making recommendations for additional experiments on this topic.
Reduced basking time was found for M. obscura towards all treatment objects, particularly the cat and quoll, albeit with high variation. This finding aligns with that of Cliff et al. (2022), who measured lizard basking by using visual cat cues (including scent) and found similar reductions. Heliothermic lizards typically decrease their basking when faced with predation risk to reduce their vulnerability. (van Damme et al. 1990; Downes 2001; Herczeg et al. 2008). For M. obscura, this may indicate wariness to novel objects. This species co-occurs with multiple predators including cats, foxes, snakes, lizards and birds, potentially causing maintenance of antipredator behaviour towards new stimuli, as predicted by the multipredator hypothesis (Blumstein 2006). Webb et al. (2010) recorded velvet geckos (Oedura lesuerii) responding to cues of both a dangerous predatory snake, the swamp snake (Hemiaspis signata) and a non-predatory snake, the golden-crowned snake (Cacophis squamulosus), likely because they co-exist with nine snake predator species; so, generalising responses to all snakes is advantageous to survival.
Vigilance behaviour increased for C. robustus during the cat treatment, whereby lizards spent more time partially emerged in the treatment compartment. This may indicate increased wariness after initial exposure to cat cues, with greater monitoring of their surroundings before emerging from refuges. For example, common wall lizards (Podarcus muralis) spend more time partially emerged after simulated predatory attacks (Pellitteri-Rosa et al. 2017). Higher occurrence of this behaviour has been recorded for low-risk cues than high-risk simulated attacks in Iberian rock lizards (Iberolacerta monticola), suggesting that lizards increase their surveillance of predators when not directly threatened (Polo et al. 2011). Ctenotus robustus may have perceived the cat model as a low-level risk and increased their vigilance towards it.
Similarities observed across treatments for most behaviours exhibited by M. obscura and C. robustus may stem from these lizards exhibiting Level 1 naïveté towards visual cues of introduced mammalian predators (Banks and Dickman 2007). Feral cats have existed in this region for ~200 years and are known to prey on Ctenotus and Morethia skinks; so, it can be reasonably assumed that these lizards have previous and continuing exposure to cat predation (Read and Bowen 2001; Legge et al. 2017; Stobo-Wilson et al. 2021). Movement, olfactory and auditory cues may be important to cue discrimination templates used by these lizards to recognise predators. Bird and reptile predators are common in this system and actively hunt lizards, whereas feral cats use stalk and pounce methods, which include long sedentary periods (Schwarzkopf and Shine 1992; Anderson and Burgin 2008; Bateman et al. 2017; Moseby and McGregor 2022). Thus, there may be a mismatch between the other cues that lizards use to recognise native predators which they share co-evolutionary experience with, and the visual cues presented here by stationary predators models (Carthey and Banks 2014). These lizards’ visual acuity may be insufficient to recognise predators from stationary visual cues alone. Diurnal lizard species typically have laterally positioned eyes with centrally positioned foveae, relying on monocular vision to perceive longer-range objects, whereas motion detection is important for identification of prey items and predators (Fleishman 1986; Fleishman 2024). For reptiles with high exposure to aerial predators, specific movement cues may be required for antipredator responses to visual stimuli, whereas the spatiotemporal context of these cues is likely to influence whether or not a response is triggered (Carlile et al. 2006).
Another possible explanation for lizards potentially failing to recognise cat cues is that alien mammalian predators may prey on lizards in this system less frequently than do native predators, resulting in weaker selection pressure. Specific antipredator responses are costly and should only exist if their benefits in reducing predation outweigh the costs (Blumstein and Daniel 2005). Lizards may also produce differential antipredator responses according to predator risk levels as defined by the threat sensitivity hypothesis (Helfman 1989; Lloyd et al. 2009).
Ambient temperature affected multiple behaviours displayed by lizards. Basking behaviour increased at lower temperatures for both M. obscura and C. robustus, demonstrating the influence of climatic conditions on activity, consistent with findings for many other lizard species (Hertz 1992; Koenig et al. 2001). Abnormally cool conditions during our sampling may have forced lizards in the predator treatments to trade-off perceived predation risk for thermoregulation to remain within safe body temperature thresholds, because antipredator responses become more costly. Ctenotus robustus also spent more time sheltering and partially emerged during lower ambient temperatures and more time exploring during higher temperatures, aligning with findings for other heliothermic lizards (Grant and Dunham 1990; Beck and Lowe 1991). Climatic conditions may have inhibited activity and resulted in adoption of a thermoconforming strategy, restricting their ability to escape from and respond to predators (Huey and Slatkin 1976; Herczeg et al. 2006).
Experimental considerations and future research
Presentation of visual cues alone may be insufficient to elicit antipredator responses from lizards exposed to mammalian predators. Monks et al. (2019) found varying responses in New Zealand lizards according to degree of coexistence with invasive rats; however, they failed to respond to chemical cues alone. Concurrently presenting multiple predator sensory cues may be required to trigger antipredator responses resembling those of lizards experiencing natural predation (Amo et al. 2004). The models in our study may also have presented lizards with additional cues to those intended, such as unique scents or appearing intimidating, which may cause generalised threat responses to novel olfactory or visual cues, potentially contributing to the high occurrence of inferred antipredatory behaviours (Kass and McGann 2017; Szabo and Ringler 2023). Further, lizards may exhibit neophobia towards unfamiliar cues presented by the treatment objects and avoid them (Carthey and Banks 2014; Szabo and Ringler 2023).
Olfactory cues have also been used in previous studies that tested lizard behavioural responses to invasive mammalian predators. Cliff et al. (2022) found reductions in basking behaviour for McCann’s skinks exposed to cat scent, and Webster et al. (2018) recorded altered foraging behaviour in Boulenger’s skinks and southern marbled geckos when exposed to fox scent. Lizards in the wild would presumably frequently encounter cat odours when they are exposed to this predator, so olfactory cues may be a more reliable means for lizards to detect cats, along with auditory cues. Cats are known to use dense cover when hunting which often conceals their presence, so their visual cues may sometimes be absent when they pose a direct predation threat to lizards (Moseby and McGregor 2022).
Future studies should consider testing other sensory cues in concert with visual cues, to allow a more complete test for predator recognition of feral cats in Australian lizards and greater confidence in identifying recognition and naïveté. Acclimatising lizards to controlled environments before commencing trials and introducing treatments may encourage lizards to perform more natural behaviours. The lack of an acclimatisation period before the arena trials may have affected lizard behaviours, potentially resulting in more fearful and unnatural responses to an unfamiliar environment, and may have partially explained behaviours that could also be considered antipredatory. Future experimental research into visual cue detection may also consider incorporating other extant native predator models (e.g. snakes, raptors) to compare responses to alien predators against those that have long existed in this system and frequently prey on lizards, and adding in movement of predator models to test whether it elicits different responses to stationary objects. Testing sensory cue recognition in arid environments where cats occur in greatest densities and consume the most reptiles may allow greater probability of detecting antipredator behaviours because selection pressure applied by cats to reptiles may be higher (Woinarski et al. 2018). This may also enable comparisons between behavioural responses for lizard populations with differential levels of exposure to cats.
Conclusions
Feral cats are an ongoing threat to Australia’s biodiversity, including by frequently preying on Australia’s diverse herpetofauna. It appears that two Australian lizard species possess little ability to recognise and respond to cats on the basis of stationary visual cues. This may indicate Level 1 naïveté, potentially driven by cue mismatch, low predation rates of cats compared with native predators, or lack of recent co-evolutionary history with native mammalian predators. Predator cue presentation and context are important considerations, where lizards may recognise cats as predators in the wild but not display this during our experiments. Future studies should test other sensory cues of cats, consider including cues for extant native predators, and compare populations with differing cat densities. This should improve confidence in findings for tests of predator recognition and prey naïveté and could be useful in determining their occurrence in reptile communities at a continental scale. Our findings are important because they imply that two lizard species largely do not use stationary visual cues to detect cats as predators, which suggests that they rely on other cues if they can recognise them, potentially imperilling them to alien predators with hunting strategies different from those of native predators. Assessing whether animals respond to introduced predators will enable exposure to predator stimuli to be used as a conservation tool, which has proved effective in facilitating learned antipredator responses, leading to higher rates of reproduction and survival in native fauna (Moseby et al. 2016; West et al. 2018). Developing a more thorough understanding of how lizard sensory ecology relates to feral cat predation will assist with identification of reptile species or communities with the greatest imperilment, enabling novel management strategies (e.g. disruption of sensory cues used by cats to hunt reptiles) to be targeted to these populations. Elucidating the mechanisms underpinning reptile–feral cat interactions will be essential for conserving Australian reptiles threatened by cats, because management actions aimed at reducing their impact will require an understanding of how lizards respond to cat predation.
Declaration of funding
This project was funded by an Australian Research Council (ARC) Discovery Project Grant: DP210100323 Quantifying the threat posed by feral cats to Australian reptiles. T. S. Doherty was supported by an ARC Discovery Early Career Researcher Award (DE200100157).
Acknowledgements
We acknowledge the Wotjobaluk people as the traditional custodians of the Wimmera region in Victoria. We thank Dylan Westaway for his collaboration and assistance with coordinating the sampling effort, and also thank Bim Nash, Ben Nash, Rommi Crouch, Shane Dodson and Michael Shalders for granting access to their properties to conduct fieldwork. We thank Adrian Wayne and Marika Maxwell for their assistance in obtaining the quoll specimen. We acknowledge the substantial voluntary contributions to our field sampling from Charlie Sonnemann, Jamie Smith-Morvell, Nicholas Gale, Kristin Semmens, Isaac Clarey, Ruben Clarey, Kurtise Templeton and Sage Runciman. We also thank Flo Burgwin, Mirinda Thorpe and Iestyn Hosking for generously providing accommodation and hospitality during fieldwork. We thank two anonymous reviewers for their feedback on an earlier version of the paper.
References
Abbott I (2008) The spread of the cat, Felis catus, in Australia: re-examination of the current conceptual model with additional information. Conservation Science Western Australia 7(1), 1-17.
| Google Scholar |
Amo L, López P, Martı́n J (2004) Wall lizards combine chemical and visual cues of ambush snake predators to avoid overestimating risk inside refuges. Animal Behaviour 67, 647-653.
| Crossref | Google Scholar |
Anderson L, Burgin S (2008) Patterns of bird predation on reptiles in small woodland remnant edges in peri-urban north-western Sydney, Australia. Landscape Ecology 23, 1039-1047.
| Crossref | Google Scholar |
Anton A, Geraldi NR, Ricciardi A, Dick JTA (2020) Global determinants of prey naiveté to exotic predators. Proceedings of the Royal Society of London – B. Biological Sciences 287, 20192978.
| Crossref | Google Scholar | PubMed |
Banks PB, Dickman CR (2007) Alien predation and the effects of multiple levels of prey naiveté. Trends in Ecology & Evolution 22, 229-230.
| Crossref | Google Scholar | PubMed |
Bateman PW, Fleming PA, Wolfe AK (2017) A different kind of ecological modelling: the use of clay model organisms to explore predator–prey interactions in vertebrates. Journal of Zoology 301, 251-262.
| Crossref | Google Scholar |
Beck DD, Lowe CH (1991) Ecology of the beaded lizard, Heloderma horridum, in a tropical dry forest in Jalisco, Mexico. Journal of Herpetology 25, 395-406.
| Crossref | Google Scholar |
Blumstein DT (2006) The multipredator hypothesis and the evolutionary persistence of antipredator behavior. Ethology 112, 209-217.
| Crossref | Google Scholar |
Blumstein DT, Daniel JC (2005) The loss of anti-predator behaviour following isolation on islands. Proceedings of the Royal Society of London – B. Biological Sciences 272, 1663-1668.
| Crossref | Google Scholar |
Blumstein DT, Ferando E, Stankowich T (2009) A test of the multipredator hypothesis: yellow-bellied marmots respond fearfully to the sight of novel and extinct predators. Animal Behaviour 78, 873-878.
| Crossref | Google Scholar |
Brooks ME, Kristensen K, van Benthem KJ, Magnusson A, Berg CW, Nielsen A, Skaug HJ, Maechler M, Bolker BM (2017) glmmTMB balances speed and flexibility among packages for zero-inflated generalized linear mixed modeling. The R Journal 9, 378-400.
| Crossref | Google Scholar |
Burbidge AA, Manly BFJ (2002) Mammal extinctions on Australian islands: causes and conservation implications. Journal of Biogeography 29, 465-473.
| Crossref | Google Scholar |
Bureau of Meteorology (2022a) Climate statistics for Australian locations. Monthly climate statistics: all years of record – Summary statistics for NHILL. (The Bureau) Available at http://www.bom.gov.au/climate/averages/tables/cw_078031.shtml [Verified 11 February 2025]
Bureau of Meteorology (2022b) Climate driver update archive: climate drivers in the Pacific, Indian and Southern oceans and the Tropics. (The Bureau) Available at http://www.bom.gov.au/climate/enso/wrap-up/archive/20221011.archive.shtml [Verified 11 February 2025]
Burunat-Pérez G, Suárez-Rancel M, Molina-Borja M (2018) Predator avoidance training of the endangered lizard from El Hierro (Canary Islands): a new management strategy before reintroduction into the wild. Behavioural Processes 157, 192-198.
| Crossref | Google Scholar | PubMed |
Carlile PA, Peters RA, Evan CS (2006) Detection of a looming stimulus by the Jacky dragon: selective sensitivity to characteristics of an aerial predator. Animal Behaviour 72, 553-562.
| Crossref | Google Scholar |
Carthey AJR, Banks PB (2014) Naïveté in novel ecological interactions: lessons from theory and experimental evidence. Biological Reviews 89, 932-949.
| Crossref | Google Scholar | PubMed |
Choi JS, Kim JJ (2010) Amygdala regulates risk of predation in rats foraging in a dynamic fear environment. Proceedings of the National Academy of Sciences 107, 21773-21777.
| Crossref | Google Scholar |
Clavero M, García-Berthou E (2005) Invasive species are a leading cause of animal extinctions. Trends in Ecology & Evolution 20, 110.
| Crossref | Google Scholar | PubMed |
Cliff HB, Jones ME, Johnson CN, Pech RP, Biemans BT, Barmuta LA, Norbury GL (2022) Rapid gain and loss of predator recognition by an evolutionarily naïve lizard. Austral Ecology 47, 641-652.
| Crossref | Google Scholar |
Creel S (2018) The control of risk hypothesis: reactive vs. proactive antipredator responses and stress-mediated vs. food-mediated costs of response. Ecology Letters 21, 947-956.
| Crossref | Google Scholar | PubMed |
Creel S, Christianson D (2008) Relationships between direct predation and risk effects. Trends in Ecology & Evolution 23, 194-201.
| Crossref | Google Scholar | PubMed |
Dalerum F, Belton L (2015) African ungulates recognize a locally extinct native predator. Behavioral Ecology 26, 215-222.
| Crossref | Google Scholar |
Doherty TS, Davis RA, van Etten EJB, Algar D, Collier N, Dickman CR, Edwards G, Masters P, Palmer R, Robinson S (2015) A continental-scale analysis of feral cat diet in Australia. Journal of Biogeography 42, 964-975.
| Crossref | Google Scholar |
Doherty TS, Glen AS, Nimmo DG, Ritchie EG, Dickman CR (2016) Invasive predators and global biodiversity loss. Proceedings of the National Academy of Sciences 113, 11261-11265.
| Crossref | Google Scholar |
Douma JC, Weedon JT (2019) Analysing continuous proportions in ecology and evolution: a practical introduction to beta and Dirichlet regression. Methods in Ecology and Evolution 10, 1412-1430.
| Crossref | Google Scholar |
Downes S (2001) Trading heat and food for safety: costs of predator avoidance in a lizard. Ecology 82, 2870-2881.
| Crossref | Google Scholar |
Dunlop JA, Rayner K, Doherty TS (2017) Dietary flexibility in small carnivores: a case study on the endangered northern quoll, Dasyurus hallucatus. Journal of Mammalogy 98, 858-866.
| Crossref | Google Scholar |
Ferrari S, Cribari-Neto F (2004) Beta regression for modelling rates and proportions. Journal of Applied Statistics 31, 799-815.
| Crossref | Google Scholar |
Fleishman LJ (1986) Motion detection in the presence and absence of background motion in an Anolis lizard. Journal of Comparative Physiology A 159, 711-720.
| Crossref | Google Scholar |
Fleishman LJ (2024) Lizard visual ecology. Frontiers in Amphibian and Reptile Science 2, 1426675.
| Crossref | Google Scholar |
Friard O, Gamba M (2016) BORIS: a free, versatile open-source event-logging software for video/audio coding and live observations. Methods in Ecology and Evolution 7, 1325-1330.
| Crossref | Google Scholar |
Gardner WK, Carter J, Flood R, Young T, Drum M, Jasper M (1992) Agriculture in the Wimmera; looking back and planning ahead. In ‘Proceedings of the 6th Australian Agronomy Conference’, 10–14 February 1992, Armidale, NSW, Australia. pp. 146–149. (Victorian Department of Agriculture: Horsham, Vic, Australia)
Geyle HM, Tingley R, Amey AP, Cogger H, Couper PJ, Cowan M, Craig MD, Doughty P, Driscoll DA, Ellis RJ, Emery JP, Fenner A, Gardner MG, Garnett ST, Gillespie GR, Greenlees MJ, Hoskin CJ, Keogh JS, Lloyd R, Melville J, McDonald PJ, Michael DR, Mitchell NJ, Sanderson C, Shea GM, Sumner J, Wapstra E, Woinarski JCZ, Chapple DG (2021) Reptiles on the brink: identifying the Australian terrestrial snake and lizard species most at risk of extinction. Pacific Conservation Biology 27, 3-12.
| Crossref | Google Scholar |
Grant BW, Dunham AE (1990) Elevational covariation in environmental constraints and life histories of the desert lizard Sceloporus merriami. Ecology 71, 1765-1776.
| Crossref | Google Scholar |
Helfman GS (1989) Threat-sensitive predator avoidance in damselfish-trumpetfish interactions. Behavioral Ecology and Sociobiology 24, 47-58.
| Crossref | Google Scholar |
Herczeg G, Gonda A, Saarikivi J, Merilä J (2006) Experimental support for the cost–benefit model of lizard thermoregulation. Behavioral Ecology and Sociobiology 60, 405-414.
| Crossref | Google Scholar |
Herczeg G, Herrero A, Saarikivi J, Gonda A, Jäntti M, Merilä J (2008) Experimental support for the cost–benefit model of lizard thermoregulation: the effects of predation risk and food supply. Oecologia 155, 1-10.
| Crossref | Google Scholar | PubMed |
Hertz PE (1992) Temperature regulation in Puerto Rican Anolis lizards: a field test using null hypotheses. Ecology 73, 1405-1417.
| Crossref | Google Scholar |
Huey RB, Slatkin M (1976) Cost and benefits of lizard thermoregulation. The Quarterly Review of Biology 51, 363-384.
| Crossref | Google Scholar | PubMed |
Kass MD, McGann JP (2017) Persistent, generalized hypersensitivity of olfactory bulb interneurons after olfactory fear generalization. Neurobiology of Learning and Memory 146, 47-57.
| Crossref | Google Scholar | PubMed |
Kavaliers M, Choleris E (2001) Antipredator responses and defensive behavior: ecological and ethological approaches for the neurosciences. Neuroscience & Biobehavioral Reviews 25, 577-586.
| Crossref | Google Scholar | PubMed |
Koenig J, Shine R, Shea G (2001) The ecology of an Australian reptile icon: how do blue-tongued lizards (Tiliqua scincoides) survive in suburbia? Wildlife Research 28, 214-227.
| Crossref | Google Scholar |
Legge S, Murphy BP, McGregor H, Woinarski JC, Augusteyn J, Ballard G, Baseler M, Buckmaster T, Dickman CR, Doherty TS, Edwards GP, Eyre T, Fancourt BA, Ferguson D, Forsyth DM, Gear WL, Gentle M, Gillespie G, Greenwood L, Hohnen R, Hume S, Johnson CN, Maxwell M, McDonald PJ, Morris K, Moseby K, Newsome T, Nimmo D, Paltridge R, Ramsey D, Read J, Rendall A, Rich M, Ritchie E, Rowland J, Short J, Stokeld D, Sutherland DR, Wayne AF, Woodford L, Zewe F (2017) Enumerating a continental-scale threat: how many feral cats are in Australia? Biological Conservation 206, 293-303.
| Crossref | Google Scholar |
Lettink M, Norbury G, Cree A, Seddon PJ, Duncan RP, Schwarz CJ (2010) Removal of introduced predators, but not artificial refuge supplementation, increases skink survival in coastal duneland. Biological Conservation 143, 72-77.
| Crossref | Google Scholar |
Lima SL, Dill LM (1990) Behavioral decisions made under the risk of predation: a review and prospectus. Canadian Journal of Zoology 68, 619-640.
| Crossref | Google Scholar |
Linley GD, Pauligk Y, Marneweck C, Ritchie EG (2021) Moon phase and nocturnal activity of native Australian mammals. Australian Mammalogy 43, 190-195.
| Crossref | Google Scholar |
Lishmund O (2023) Behavioural responses of Australian lizards towards visual cues of feral cats. FigShare 2023, 6968802 [Data files, uploaded 7 December 2023].
| Crossref | Google Scholar |
Lloyd R, Alford RA, Schwarzkopf L (2009) Chemical discrimination among predators by lizards: responses of three skink species to the odours of high- and low-threat varanid predators. Austral Ecology 34, 50-54.
| Crossref | Google Scholar |
McGregor H, Legge S, Jones ME, Johnson CN (2015) Feral cats are better killers in open habitats, revealed by animal-borne video. PLoS ONE 10, e0133915.
| Crossref | Google Scholar |
Monks JM, Nelson NJ, Daugherty CH, Brunton DH, Shine R (2019) Does evolution in isolation from mammalian predators have behavioural and chemosensory consequences for New Zealand lizards? New Zealand Journal of Ecology 43, 3359.
| Crossref | Google Scholar |
Moseby KE, McGregor HM (2022) Feral cats use fine scale prey cues and microhabitat patches of dense vegetation when hunting prey in arid Australia. Global Ecology and Conservation 35, e02093.
| Crossref | Google Scholar |
Moseby KE, Hill BM, Read JL (2009) Arid recovery – a comparison of reptile and small mammal populations inside and outside a large rabbit, cat and fox-proof exclosure in arid South Australia. Austral Ecology 34, 156-169.
| Crossref | Google Scholar |
Moseby KE, Blumstein DT, Letnic M (2016) Harnessing natural selection to tackle the problem of prey naïveté. Evolutionary Applications 9, 334-343.
| Crossref | Google Scholar | PubMed |
Nunes AL, Orizaola G, Laurila A, Rebelo R (2014) Rapid evolution of constitutive and inducible defenses against an invasive predator. Ecology 95, 1520-1530.
| Crossref | Google Scholar | PubMed |
Peacock D, Abbott I (2014) When the ‘native cat’ would ‘plague’: historical hyperabundance in the quoll (Marsupialia: Dasyuridae) and an assessment of the role of disease, cats and foxes in its curtailment. Australian Journal of Zoology 62, 294-344.
| Crossref | Google Scholar |
Pellitteri-Rosa D, Bellati A, Cocca W, Gazzola A, Martín J, Fasola M (2017) Urbanization affects refuge use and habituation to predators in a polymorphic lizard. Animal Behaviour 123, 359-367.
| Crossref | Google Scholar |
Polo V, López P, Martín J (2011) Uncertainty about future predation risk modulates monitoring behavior from refuges in lizards. Behavioral Ecology 22, 218-223.
| Crossref | Google Scholar |
Read J, Bowen Z (2001) Population dynamics, diet and aspects of the biology of feral cats and foxes in arid South Australia. Wildlife Research 28, 195-203.
| Crossref | Google Scholar |
Roshier DA, Hotellier FL, Carter A, Kemp L, Potts J, Hayward MW, Legge SM (2020) Long-term benefits and short-term costs: small vertebrate responses to predator exclusion and native mammal reintroductions in south-western New South Wales, Australia. Wildlife Research 47, 570-579.
| Crossref | Google Scholar |
Schoener TW, Spiller DA, Losos JB (2001) Predators increase the risk of catastrophic extinction of prey populations. Nature 412, 183-186.
| Crossref | Google Scholar | PubMed |
Schwarzkopf L, Shine R (1992) Costs of reproduction in lizards: escape tactics and susceptibility to predation. Behavioral Ecology and Sociobiology 31, 17-25.
| Crossref | Google Scholar |
Sih A, Bolnick DI, Luttbeg B, Orrock JL, Peacor SD, Pintor LM, Preisser E, Rehage JS, Vonesh JR (2010) Predator–prey naïveté, antipredator behavior, and the ecology of predator invasions. Oikos 119, 610-621.
| Crossref | Google Scholar |
Spence-Bailey LM, Nimmo DG, Kelly LT, Bennett AF, Clarke MF (2010) Maximising trapping efficiency in reptile surveys: the role of seasonality, weather conditions and moon phase on capture success. Wildlife Research 37, 104-115.
| Crossref | Google Scholar |
Spencer RJ (2002) Experimentally testing nest site selection: fitness trade-offs and predation risk in turtles. Ecology 83, 2136-2144.
| Crossref | Google Scholar |
Stobo-Wilson AM, Murphy BP, Legge SM, Chapple DG, Crawford HM, Dawson SJ, Dickman CR, Doherty TS, Fleming PA, Gentle M, Newsome TM, Palmer R, Rees MW, Ritchie EG, Speed J, Stuart JM, Thompson E, Turpin J, Woinarski JCZ (2021) Reptiles as food: predation of Australian reptiles by introduced red foxes compounds and complements predation by cats. Wildlife Research 48, 470-480.
| Crossref | Google Scholar |
Szabo B, Ringler E (2023) Fear of the new? Geckos hesitate to attack novel prey, feed near objects and enter a novel space. Animal Cognition 26, 537-549.
| Crossref | Google Scholar | PubMed |
van Damme R, Bauwens D, Vanderstighelen D, Verheyen RF (1990) Responses of the lizard Lacerta vivipara to predator chemical cues: the effects of temperature. Animal Behaviour 40, 298-305.
| Crossref | Google Scholar |
Vucetich JA, Peterson RO, Schaefer CL (2002) The effect of prey and predator densities on wolf predation. Ecology 83, 3003-3013.
| Crossref | Google Scholar |
Wallis RL (2021) Historical reports of quolls in Victoria’s south-west. The Victorian Naturalis 138, 78-85.
| Crossref | Google Scholar |
Wang X, Zanette L, Zou X (2016) Modelling the fear effect in predator–prey interactions. Journal of Mathematical Biology 73, 1179-1204.
| Crossref | Google Scholar | PubMed |
Webb JK, Du W, Pike D, Shine R (2010) Generalization of predator recognition: velvet geckos display anti-predator behaviours in response to chemicals from non-dangerous elapid snakes. Current Zoology 56, 337-342.
| Crossref | Google Scholar |
Webster C, Massaro M, Michael DR, Bambrick D, Riley JL, Nimmo DG (2018) Native reptiles alter their foraging in the presence of the olfactory cues of invasive mammalian predators. Royal Society Open Science 5(10), 180136.
| Crossref | Google Scholar | PubMed |
West R, Letnic M, Blumstein DT, Moseby KE (2018) Predator exposure improves anti-predator responses in a threatened mammal. Journal of Applied Ecology 55, 147-156.
| Crossref | Google Scholar |
Woinarski JCZ, Burbidge AA, Harrison PL (2015) Ongoing unraveling of a continental fauna: decline and extinction of Australian mammals since European settlement. Proceedings of the National Academy of Sciences 112, 4531-4540.
| Crossref | Google Scholar |
Woinarski JCZ, Murphy BP, Legge SM, Garnett ST, Lawes MJ, Comer S, Dickman CR, Doherty TS, Edwards G, Nankivell A, Paton D, Palmer R, Woolley LA (2017) How many birds are killed by cats in Australia? Biological Conservation 214, 76-87.
| Crossref | Google Scholar |
Woinarski JCZ, Murphy BP, Palmer R, Legge SM, Dickman CR, Doherty TS, Edwards G, Nankivell A, Read JL, Stokeld D (2018) How many reptiles are killed by cats in Australia? Wildlife Research 45, 247-266.
| Crossref | Google Scholar |
Zenger KR, Richardson BJ, Vachot-Griffin AM (2003) A rapid population expansion retains genetic diversity within European rabbits in Australia. Molecular Ecology 12, 789-794.
| Crossref | Google Scholar | PubMed |