Framework for a savanna burning emissions abatement methodology applicable to fire-prone miombo woodlands in southern Africa
Jeremy Russell-Smith A B * , Cameron Yates A B , Roland Vernooij C , Tom Eames D , Diane Lucas B , Keddy Mbindo E , Sarah Banda E , Kanembwa Mukoma E , Adrian Kaluka F , Alex Liseli F , Jomo Mafoko G , Othusitse Lekoko A B H , Robin Beatty I , Mirjam Kaestli J , Guido van der Werf C and Natasha Ribeiro KA
B
C
D
E
F
G
H
I
J
K
Abstract
To assess development of a robust emissions accounting framework for expansive miombo woodland savannas covering ~2 million km2 of southern Africa that typically are burnt under relatively severe late dry season (LDS) conditions.
A detailed site-based study of fuel accumulation, combustion and greenhouse gas (GHG) emission factor parameters under early dry season (EDS) and LDS conditions along a central rainfall-productivity and associated miombo vegetation structural and floristics gradient, from lower rainfallsites in northern Botswana to higher rainfall sites in northern Zambia.
Assembled field data inform core components of the proposed emissions reduction framework: fuel and combustion conditions sampled across the vegetation/productivity gradient can be represented by three defined Vegetation Fuel Types (VFTs); fuel accumulation, combustion and emissions parameters are presented for these. Applying this framework for an illustrative case, GHG emissions (t CO2-e) from EDS fires were one-third to half those of LDS fires per unit area in eligible miombo VFTs.
Our accounting framework supports undertaking EDS fire management to significantly reduce emissions and, realistically, burnt extent at landscape scales. We consider application of presented data to development of formal emissions abatement accounting methods, linkages with potential complementary woody biomass and soil organic carbon sequestration approaches, and necessary caveats concerning implementation issues.
Keywords: carbon markets, emission factors, fire management, fuel accumulation, fuel combustion, greenhouse gases, miombo.
Introduction
The development and application of sustainable fire management approaches in fire-prone savanna landscapes poses recognised environmental, ecologic and attendant socio-economic challenges. Savanna fires, especially from tropical biomes in northern and southern hemisphere Africa, account for the vast majority of contemporary global burnt area and fire carbon emissions, lit mostly by people under fairly severe late dry season conditions for an array of agricultural and subsistence purposes (van der Werf et al. 2017; Giglio et al. 2018; Ramo et al. 2021). Building on extensive Australian experience with the undertaking of Kyoto-compliant, commercial ‘savanna burning’ greenhouse gas (GHG) emissions abatement projects, particularly by reducing the extent and severity of late dry season (LDS) fires through prescribed early dry season (EDS) management (Russell-Smith et al. 2013a; Edwards et al. 2021), it has been suggested that a similar approach could provide tangible environmental and societal benefits in comparable savanna landscape settings elsewhere (ISFMI (International Savanna Fire Management Initiative) 2015; Lipsett-Moore et al. 2018; Moura et al. 2019; Russell-Smith et al. 2013b, 2021; Tear et al. 2021). A complementary approach accounting for fire regime effects on woody biomass sequestration in Australian savannas is under advanced development (Murphy et al. 2023; Paul and Roxburgh 2024).
In their global assessment of the potential implementation of the savanna burning emissions abatement methodology as applied in Australia, Lipsett-Moore et al. (2018) concluded that such an approach could feasibly be applied in at least 26 African countries with eligible savanna-type vegetation, mean annual rainfall (MAR) >600 mm, LDS emissions exceeding EDS emissions by at least 200,000 t CO2-e year−1, and fairly unpopulated protected areas >1000 km2 suitable for EDS fire management. In a further assessment of the applicability of the Australian methodology to a focal southern African region covering seven contiguous countries, Russell-Smith et al. (2021) observed that >80% of annual savanna large fire (>250 ha) extent (2001–2019) and associated emissions (2003–2019) occurred under LDS conditions, predominantly in sparsely inhabited areas. That assessment demonstrated that prescribed EDS fire management to reduce LDS wildfire extent and resultant emissions met key technical criteria: LDS fine fuels tended to be greater than EDS fuels given seasonal leaf litter inputs; LDS fires tended to be significantly more severe and combust more fuels; methane and nitrous oxide emission factors were essentially equivalent in EDS and LDS periods under cured fuel conditions.
The latter authors noted, however, that: (1) application of a fairly conservative, low severity savanna burning approach is not appropriate in situations at risk of exacerbating woody encroachment and associated loss of open habitat and dependent biodiversity (Parr et al. 2014; Veldman et al. 2015; Abreu et al. 2017; Bond 2019); (2) there are various significant key implementation challenges (e.g. national regulatory issues, project governance arrangements, equity and rights concerns, capacity building opportunities, market instruments) that need to be addressed to progress development of savanna burning methods for incentivising sustainable fire management and supporting community livelihoods in wildfire-dominated southern African savannas.
In this paper, we extend the preliminary technical assessment of Russell-Smith et al. (2021) to present a detailed study of fuel accumulation, combustion and GHG emission factor (EF) characteristics in extensive miombo woody savannas with grassy understoreys in southern Africa, a requisite for parametrising a robust avoided emissions method founded on current savanna burning Australian regulation and practice. We ask whether a practical methodological approach utilising prescribed fire management under EDS fuel and fire-weather conditions can substantially reduce GHG emissions in miombo woodlands as demonstrated in Australian savannas. Our study takes advantage of fieldwork conducted between 2019 and 2023 under both EDS and LDS conditions, mostly along a central rainfall-productivity and associated miombo vegetation structural and floristics gradient, from low rainfall (~600 mm MAR) sites in northern Botswana to high rainfall (~1500 mm MAR) sites in northern Zambia. In discussion, we consider the application of presented data to development of formal emissions abatement accounting Standards, and linkages to potential complementary woody biomass and soil organic carbon (SOC) sequestration, and REDD + forest degradation and deforestation accounting approaches.
Methods
Background to the ‘savanna burning’ emissions abatement methodology
The essential premise underlying Australia’s iterative savanna burning methods (CoA (Commonwealth of Australia) 2013, 2015, 2018) involves undertaking strategic prescribed burning of cured ground fuels (grass, litter, coarse woody debris – CWD, heavy woody fuels – HWF) through the EDS to reduce the risk of extensive wildfires under relatively severe LDS fire-weather conditions (high temperatures, low humidity, gusty winds), thereby significantly reducing GHG emissions and achieving a variety of other benefits (e.g. biodiversity conservation, pasture management, asset protection). The intent is not to eliminate the incidence of LDS fires but, rather, to allow safe deployment of relatively intense fires within a matrix of EDS fire-treated fuel-breaks for required purposes (e.g. habitat management, woody plant control). The method is founded on a long regional tradition of Aboriginal customary fire and land management practice (Yibarbuk et al. 2001; Russell-Smith et al. 2003; Garde et al. 2009; Vigilante et al. 2009).
Following Seiler and Crutzen (1980) and IPCC (Intergovernmental Panel on Climate Change) (1997) in their most basic form, GHG emissions from savanna fires for a defined project area or region can be calculated as the product of the area burnt, the mass of available fuel components (grass litter, CWD, HWF, shrubs) that are consumed and EFs of respective GHG species taking into account their climate warming potential relative to CO2. For emissions of carbonaceous species, EFs are defined relative to fuel carbon; nitrogenous species are expressed relative to fuel nitrogen. Fuel carbon mass is determined from fuel mass multiplied by fuel carbon content, while fuel nitrogen is derived from the fuel mass by the product of either the carbon content and the fuel nitrogen to carbon ratio, or the fuel mass and fuel nitrogen content. GHG emissions accounting from savanna fires conventionally consider only emissions of methane (CH4) and nitrous oxide (N2O), ignoring the significant impacts of other GHGs including CO2 itself, together with more reactive species (the ozone precursors comprising CO, volatile organic compounds and oxides of nitrogen).
For this assessment, we adapt, with fairly minor modifications, a currently applied version of Australia’s savanna burning methodology that accounts solely for emissions of GHGs (CoA 2015). Under IPCC guidelines, the method is Tier 2, disaggregated spatially, empirically derived with regional-specific EFs and parameters. The method takes into account contrasting emissions profiles produced under different seasonal fire-weather conditions – accounting for less fuel consumption under relatively mild EDS conditions associated with enhanced spatial unburnt patchiness and less fuel pyrolisation, versus generally more intense wildfires under LDS conditions and typically increased fuel loads given progressive dry season litterfall (Cook 2003; Yates et al. 2020). This dry seasonal contrast in fire-weather conditions also applies generally across the distributional range of miombo woodlands (e.g. Frost 1996; Russell-Smith et al. 2021), including the accession of increased LDS fuel loads associated with the dominance of seasonally deciduous miombo taxa (Malaisse et al. 1975; Frost 1996; Ribeiro et al. 2013). Studies both in Australian savanna and African miombo systems demonstrate that EFs of CH4, N2O, CO are more or less equivalent from burning cured fuels under EDS or LDS conditions (Hurst et al. 1994; Meyer et al. 2012; Russell-Smith et al. 2021; Vernooij et al. 2023). The methodological approach is only applicable where there is an extended (e.g. 5–6 months) annual dry season period with negligible rain and fully cured ground fuels.
In Australian savanna burning methods, the EDS period is defined as pre-August and the LDS thereafter. Although this temporal division is evidently somewhat arbitrary given inter-regional and -annual variability (Perry et al. 2020; Eames et al. 2023), it reflects generally the time of year in the dry season after which fires begin to burn through the night (Maier and Russell-Smith 2012), and accords well with time-tested Aboriginal seasonal calendars recognising the onset of severe fire-weather conditions (Russell-Smith et al. 2003; Garde et al. 2009; Vigilante et al. 2009). Given markedly equivalent climatic seasonality across the ranges of both Australian savanna and African miombo systems (Frost 1996; Archibald et al. 2009, 2010; Russell-Smith et al. 2021; Eames et al. 2023), for convenience the same pragmatic late July cut-off default is applied here to account for differential seasonal fire behaviour processes (see also Chidumayo 1997a, 1997b).
For the undertaking of savanna burning projects, the Australian emissions accounting method compares mean fire emissions (measured as t CO2-e) produced during a defined pre-project period (10 or 15 years, depending on MAR variability) against annual emissions reductions resulting from prescribed fire management activities. A generalised description of the application of the emissions methodology at a project scale, including associated emissions accounting and operational procedures, is given in Russell-Smith et al. (2013a). The current savanna burning emissions abatement method is due to be superseded in the near future by a Tier 3 modelled method accounting for either or both GHG emissions abatement and complementary sequestration in woody biomass stocks. Current (and proposed) savanna burning accounting methods directly link with and are accounted for in Australia’s National Greenhouse Gas Inventory.
Southern African miombo woodlands
We focus on development of a robust savanna burning emissions abatement methodology for southern African miombo woodlands covering an extensive portion of southern Africa (Fig. 1a), ranging across 11 countries bounded by the Democratic Republic of Congo and Tanzania in the north, Mozambique in the east, Angola in the west, and Zimbabwe, Botswana and Namibia in the south. Broadly defined, miombo woodlands are included within the Miombo Ecoregion (Fig. 1b; Timberlake and Chidumayo 2011) spanning 3.8 million km2, occurring mostly at 800–1250 m above sea level (asl) on typically infertile, acidic, freely draining sandy soils of the ancient African Plateau, under markedly southern hemisphere summer seasonal (November–April) rainfall conditions ranging generally between 600 and 1600 mm MAR. Recent reappraisal of the distribution of miombo vegetation suggests the current extent of miombo woodland in the strictest sense is ~1.9 million km2 (Fig. 1c; Dziba et al. 2020). Fires recur frequently throughout the range of miombo ecoregion (Fig. 1d), occurring especially under relatively severe LDS fire-weather conditions (Supplementary Fig. S1), resulting in globally significant GHG emissions (van der Werf et al. 2017; Russell-Smith et al. 2021).
Distribution of miombo woodlands: (a) African major vegetation types (Ribeiro et al. 2015 – after White 1983); (b) Miombo Ecoregion vegetation (Timberlake and Chidumayo 2011); (c) Miombo vegetation (Dziba et al. 2020); (d) fire frequency, 2001–2022, derived from MODIS (Moderate Resolution Imaging Spectroradiometer) 500 m automated mapping product (Giglio et al. 2018).
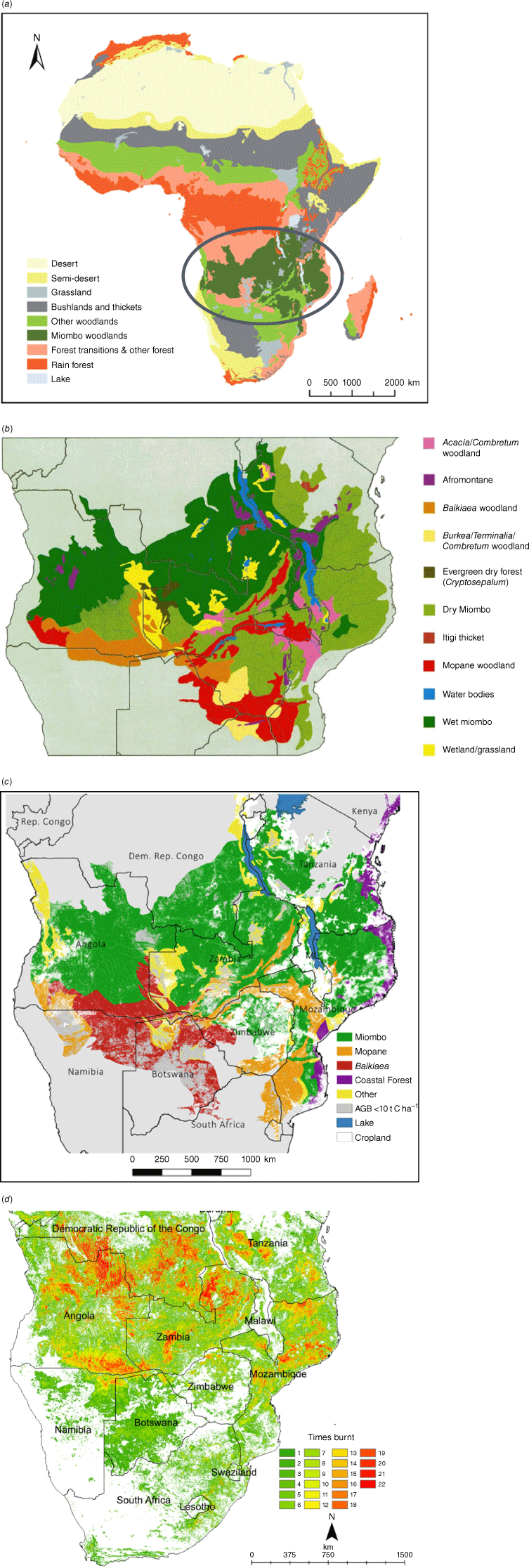
Miombo woodlands are characterised by the dominance of members of the leguminous (subfamily Deltoideae) tree genera Brachystegia (~20 spp.), Julbernardia (2 spp.) and Isoberlinia (1 sp.), typically over well-developed C4 grassy understoreys (White 1983; Frost 1996; Chidumayo 1997a; Timberlake and Chidumayo 2011; Ribeiro et al. 2020b). Although characterised as being generally floristically homogenous across their range, more floristically diverse (notably including many other legume tree species) and structurally better developed (e.g. taller, higher biomass) miombo woodlands occur under higher rainfall conditions (White 1983; Frost 1996; Godlee et al. 2021), especially in Zambia (White 1983). By convention, miombo formations occurring under >1000 mm MAR conditions are described as Wet Miombo, and those occurring under lower (<1000 mm MAR) rainfall conditions as Dry Miombo (e.g. White 1983; Frost 1996; Ribeiro et al. 2020b). Seasonal deciduousness in miombo tree species, hence leaf litter fuel input in the LDS, increases generally with declining rainfall/seasonal soil moisture availability (Frost 1996; Ribeiro et al. 2020b).
As well as the miombo woodlands defined above, for methods development, we have also included extensive legume-dominated woodlands adjoining and intergrading with Dry Miombo in southern, low rainfall regions, dominated by the slow-growing, deciduous tree Baikiaea plurijuga (Zambezi teak) (Fig. 1b, c). Baikiaea woodlands are developed typically on deep, infertile, free-draining Kalahari sands (White 1983) and, like miombo woodlands generally, are very susceptible to severe fire regimes (Frost and Robertson 1987; Holdo 2005; Russell-Smith et al. 2021; Cassidy et al. 2022). Conversely, we have excluded fairly geographically restricted woodland formations from the Miombo Ecoregion as described by White (1983), Timberlake and Chidumayo (2011), Ribeiro et al. (2020b), especially those dominated by Colophospermum mopane (mopane) and Acacia/Senegalia–Combretum (Acacia), and a variety of other Zambezian restricted regional types noted by White (1983). Legume-dominated Acacia and deciduous mopane types typically both occur on heavier-textured, nutrient-rich, less well-drained soils (in the case of mopane, often with deflocculated sodic subsoils) than Miombo and Baikiaea vegetation fuel types. Further field studies would be required to assess the fuel accumulation and emissions properties of these restricted fuel types.
Other vegetation types excluded from our methods application include Afromontane, Evergreen dry forest, Itigi thicket formations (after Timberlake and Chidumayo 2011) and more localised embedded vegetation types associated with termitaria, riparian systems, omuramba (dune swales), and seasonally flooded (dambo) and freely draining grasslands systems. Dambos, omuramba and natural grasslands occur extensively in various southern African landscape settings but are excluded from the proposed emissions abatement methodology given that prescribed more conservative EDS fire management activities would likely result in woody encroachment and thickening, and hence significant deleterious impacts on floristic and wildlife species diversity associated with loss of open grazing (O’Connor et al. 2014; Parr et al. 2014) and avian habitat (Sirami and Monadjem 2012). However, as noted in the discussion, EDS prescribed fire management in adjacent woodland savannas can allow safe application of more intense fires in embedded open grassy habitats.
Sampling rationale and study sites
Given the recognised strong relationship between miombo floristic and vegetation structure with MAR described above, our field assessment program was designed to provide a cost-efficient and -effective strategy for sampling core miombo vegetation structural and fuel load accumulation, combustion and emissions attributes focused on the central geographic miombo rainfall/productivity gradient, from low-rainfall conditions ~600 mm MAR in northern Botswana to more productive, higher rainfall sites ~1500 mm MAR in north-central Zambia (Fig. 2). The field sampling program was undertaken progressively between 2019 and 2023, with an enforced hiatus in 2020 due to COVID (Table 1). Sampling of fuel accumulation and combustion parameters was undertaken also in the Niassa Special Reserve, Mozambique, in 2019, but these unreplicated data (17 individual transects, reported in Russell-Smith et al. 2021) are not utilised here. A similar rainfall gradient-focused sampling approach was taken with developing Australia’s original savanna burning methods, on the basis that sampled fire-prone structural and floristic vegetation types were effectively representative of north Australian savanna vegetation types in general (Russell-Smith et al. 2009; CoA 2013).
Location of miombo study sites in Botswana and Zambia, with reference to MAR gradient. Refer text for details.
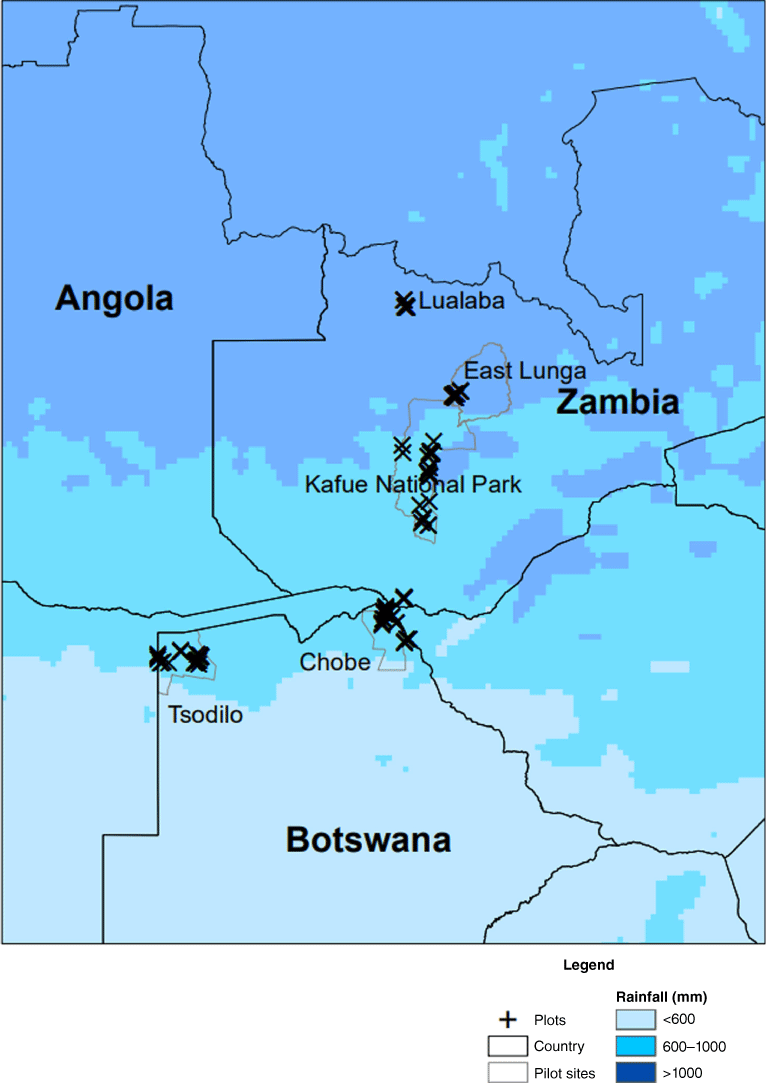
Year | Location | Sampling activity | ||||||
---|---|---|---|---|---|---|---|---|
Transect-based | Drone-based | |||||||
Pre-fire fuel accumulation | Post-fire combustion | Emission factors | ||||||
EDS | LDS | EDS | LDS | EDS | LDS | |||
2019 | Tsodilo Hills World Heritage, Botswana A | × | × | × | × | × | × | |
Niassa Special Reserve, Mozambique A, C | × | × | ||||||
2020 | No field campaign owing to COVID | |||||||
2021 | Tsodilo Hills World Heritage, Botswana A | × | × | × | × | |||
Chobe Forest Reserves, Botswana A | × | × | × | × | × | × | ||
Bovu Forest Reserve, Zambia A | × | × | × | |||||
Kafue National Park, Zambia A | × | × | × | |||||
2022 | Chobe Forest Reserves, Botswana A | × | × | |||||
Kafue National Park, Zambia A, B | × | × | × | × | × | × | ||
Loualaba Forest Reserve, Zambia B | × | × | × | |||||
East Lunga Forest Reserve, Zambia B | × | × | ||||||
2023 | East Lunga Forest Reserve, Zambia B | × |
EDS, early dry season (pre-August); LDS, late dry season (August onwards).
Field sampling was undertaken at sampling locations (sites) that were unimpacted by contemporary agricultural, pastoral and logging practices, comprising extensive structurally representative vegetation under ambient fire regime and grazing/browsing conditions in designated Forest Reserves and conservation areas (e.g. National Parks and equivalent, Tsodilo World Heritage site; Table 1). For safety reasons, site sampling was undertaken adjacent to access tracks typically within sight of vehicles, mostly under armed guard supervision. Selection of plot locations was based initially on time-since-last-burnt mapping derived from 5 years of Landsat 30 m fire history mapping and, for analysis purposes as presented here, ultimately checked with reference to 10 years prior manual Landsat (30 m pixel) fire mapping following procedures described by Evans and Russell-Smith (2019). The Collection 6 MODIS 500 m burnt area data (after Giglio et al. 2018) were used for identifying potential sites for EDS 2022 fieldwork in northern Zambia, followed by checking from the Landsat archive following plot establishment. It is well recognised that the MODIS 500 m product significantly under-represents (by at least 50%) fire extent in African contexts, especially burnt patches <100 ha (Roteta et al. 2019; Ramo et al. 2021).
Field assessment and analyses
Based on methods modified from Australian (Russell-Smith et al. 2009; Murphy et al. 2015; Yates et al. 2015), and as previously reported for southern African studies (Russell-Smith et al. 2021), transects (50 × 10 m) were established typically in groups of two or mostly three where each replicate was located in structurally similar vegetation of equivalent fuel age based on Landsat-derived time-since-fire mapping, separated by at least 100 m. Groups of transects were established in as broad a range of time-since-burnt fuel age conditions as practicable given access constraints. A total of 169 fuel accumulation plots (488 transects) were sampled under pre-fire treatment conditions over the assessment period 2019–2023 at respective sites (Table 2), noting that sampled data were utilised from different numbers of plots in respective assessments as indicated.
Sites | No. plots (transects) | ||||
---|---|---|---|---|---|
<1000 mm MAR | >1000 mm MAR | ||||
EDS | LDS | EDS | LDS | ||
(a) Pre-fire fuel accumulation | |||||
Botswana | 65 (180) | 19 (53) | |||
Zambia | 15 (45) | 17 (51) | 22 (66) | 31 (93) | |
Total | 80 (225) | 36 (104) | 22 (66) | 31 (93) | |
(b) Post-fire consumption | |||||
Botswana | 30 (75) | 12 (40) | |||
Zambia | 9 (26) | 11 (33) | 5 (8) | 26 (77) | |
Total | 39 (101) | 23 (73) | 5 (8) | 26 (77) |
EDS, early dry season (pre-August); LDS, late dry season (after August). Note lower numbers of observations sometimes used for calculation of respective accumulation and consumption parameters described in Table 4.
Sampling details for measurement of different fuel components (fine fuels – grass, litter; CWD, 6–50 mm diameter; HWF, >50 mm diameter; shrub fuels – <50 mm diameter at breast height (DBH, measured at 1.3 m) and tree stems (≥5 cm DBH)) are as given in Russell-Smith et al. (2021). Basal area (BA, m2 ha−1) was calculated subsequently for all living and dead tree stems for each transect and plot. Additionally, all tree stems were identified to species, canopy top height estimated (following clinometer or laser range-finder calibration), and canopy cover assessed by recording occurrence of any part of overlapping canopy cover (foliage, limbs) in 20 × 2.5 m sections along the central transect tape. We note that estimates strictly of foliage cover were inappropriate given progressive canopy deciduousness over the dry season. A Canopy Cover Index (CCI), calculated as the sum of 0–20 from cover observations for respective transects, was used to discriminate between Open Woodland (mean plot CCI ≤ 2), and Woodland (mean plot CCI > 2) structural types. Following Australian convention, Open woodland reflects canopy cover <20%, and Woodland >20% (CoA 2012).
Using a prepared pro forma, the volume of HWFs in a 5 × 50 m belt transect was estimated recording the length, diameter and hollowness of all materials. Assuming each piece of heavy fuel was cylindrical in shape, we estimated the total volume of HWF and total mass assuming wood density of 0.61 g cm−3 (following Handavu et al. 2021).
To estimate shrub biomass, we harvested and weighed separately the aboveground leaves and stems of three replicates of seven common taxa in four respective height classes (<0.5 m, 0–5–<1 m, 1–2 m, >2 –<5 cm DBH), taking sub-samples for oven dry weight (ODW) determinations (Supplementary Table S1), to convert field measures to dry weight. Based on these measures, sampled shrub species were allocated to one of eight representative ‘form classes’, where the eighth class represented the mean ODW biomass of the seven form-height classes and was applied to taxa not readily fitting the other seven form classes. Sampled shrub densities were then converted to aboveground dry biomass for each height class by applying the mean shrub ODW mass calculated for each form class.
Immediately following measurement of fuel and vegetation structural characteristics, fire treatments were undertaken under EDS and LDS conditions at 93 plots (259 transects) at respective sites (Table 2). EDS treatments were undertaken as perimeter spot-ignition prescribed burns (incorporating both fronting fires and backburns) ranging in extent from ~5 to 100 ha undertaken under late afternoon, fairly benign fire-climate conditions. LDS treatments were undertaken similarly but under midday high-temperature seasonally typical fire-weather conditions. LDS fire treatments especially required careful prior planning (e.g. with reference to mapped fires from current Landsat imagery) to ensure treatment fires would be effectively contained within surrounding recently burnt fuels or by other barriers (roads, streamlines) – no treatment fires escaped. As reported in Russell-Smith et al. (2021), LDS post-fire measurements included 24 of 31 transects established in anticipated advance of an extensive LDS fire-front at the Tsodilo site in 2019.
Post-fire assessments of fuel component consumption were undertaken later the same day or early the next after imposed fire treatments, following generally the same procedures as for pre-fire measurements, as described in Russell-Smith et al. (2021). Fire severity at respective burned transects was assessed following Russell-Smith et al. (2009): Low severity, leaf scorch <2 m; Moderate severity, scorch >2 m but <tree canopy top height; High severity, tree canopies scorched. Post-fire percentage unburnt patchiness was assessed in respective transects in five 1 × 10 m sections along the centre of each transect (Table 1), and additionally with drone-based assessments at some sites, as reported in Russell-Smith et al. (2021) but given lack of sampling consistency, these data are not reported here.
Assessment of pre-fire fuel accumulation for respective fuel components (fine fuels, CWD, HWF, shrubs) was undertaken with reference to data assembled from 148 plots, omitting 11 very-long-unburnt (30+ years) plots mostly from the Chobe (Botswana) site, and five high-rainfall plots in northern Zambia with uncertain recent fire histories. Relationships between accumulation of respective fuel components and time since fire were assessed with simple linear regression where both variables were natural log-transformed. Mean fuel loads were determined for respective fuel components in the absence of significant (P < 0.05) accumulation relationships with time since fire. Given recognition of seasonal deciduousness in miombo systems and the significance of litter fuels in total fuel availability (refer to Results), in the absence of seasonal litter accumulation observations from individual sites, we estimated seasonal litter contributions by adjusting for the difference in the proportions of litter:fine fuels as determined respectively from EDS and LDS samples. Similar approaches have been applied in Australian studies to account for seasonal litter contributions (Russell-Smith et al. 2009; Yates et al. 2015).
Given highly variable observations of the extent of burning and resultant severity of especially EDS fire treatments at the plot scale, assessment of mean post-fire combustion and burnt patchiness parameters was undertaken with data assembled from individual transects where treatment fires had burnt at least 20% of respective transects.
Drone-based sampling of CO2, CO, CH4 and N2O emissions from EDS and LDS fires (Table 1), including at sites in the Niassa Special Reserve (Mozambique), followed established methods in Russell-Smith et al. (2021) and Vernooij et al. (2022). We also estimated fuel C and N content in miombo woodland vegetation for EF calculations, following Russell-Smith et al. (2021). Grass, litter and CWD fuel sub-samples were collected, ground and analysed for total N and total C contents.
For gas mixing ratio analysis, cavity ring-down spectroscopy was employed. Collected samples in Tedlar bags were stored in low-light conditions and analysed within 12 h to prevent CO oxidation by OH radicals. Gas samples underwent a 20-s measurement at 1.3 L min–1 using a CO2 and CH4 analyser (LGR), followed by a 20-s measurement at 0.25 L min–1 using a CO and N2O analyser (Aeris Pico). Trace gas concentrations were averaged over 10 s after an initial 10-s flushing. Four ‘background’ samples were collected before each fire, and their average mixing ratio was subtracted from plume samples to determine the excess mixing ratio (EMR).
We express EFs for CO and CH4 as the number of moles of emitted species per mole of burned carbon using the equation:
where ERi represents the emission ratio of species i, Ci is the moles of carbon emitted in species i, and ΔCtotal is the total moles of emitted carbon. To calculate ΔCtotal, we sum the EMRs (i.e. the difference between smoke and background mixing ratios) of CO2, CO and CH4, along with the moles of carbon in non-methane hydrocarbons (NMHC) and carbonaceous particulate matter (CPM). As NMHC and CPM were not directly measured, we estimated their fractions based on prior savanna studies. CPM was approximated at 9.7% of emitted CO mass, with 48% carbon content. NMHC carbon content was estimated at 3.5 times the ER of CH4 to CO2 based on typical savanna fire ratios (Yokelson et al. 2011, 2013; Andreae 2019). We derived the EF for N2O by dividing the N2O ER by the molar-weighted average N:C ratio in the burned fuel using this equation described by Hurst et al. (1994):
Before each fire event, we collected and weighed vegetation samples from various fuel types, measured their carbon and nitrogen contents, and calculated the weighted average carbon and nitrogen contents for the burned fuel mixture.
Floristic relationships with environmental (MAR, fire frequency (FF)) and vegetation structure (BA, CCI) variables were explored with multivariate analyses conducted in Primer-E v7 (Plymouth, UK). The dataset comprised the presence–absence of 62 common tree species occurring at five or more of 160 sampled plots, excluding nine plots all from the Tsodilo Hills site lacking live common tree species. MAR and FF per plot were attributed respectively from the European Centre for Medium-Range Weather Forecasts Copernicus global data set (https://cds.climate.copernicus.eu/), 1981–2020, and FF following fire mapping procedures described in Evans and Russell-Smith (2019) with reference to the Landsat imagery archive for at least the 5 years preceding field sampling. BA and CCI were derived from plot data, with plot-scale vegetation structure attributed as Open Woodland (O) where combined CCI transect values were <10%, or Woodland (W) >10%.
A Sorensen resemblance matrix was generated based on presence–absence tree species, visualised with a principal coordinates analysis ordination (PCO). A permutational distance-based linear model was performed to assess the extent to which normalised predictor variables (MAR, FF, CCI, BA) explained floristic variation. The BEST procedure (comparing all possible combinations of predictors) was used to determine the best-fitting multiple regression model using Akaike Information Criterion-c (AICc). The model was visualised with a distance-based redundancy analysis (dbRDA) constrained ordination. Linear Pearson correlations were computed between predictor variables. No variable showed strong collinearity (Pearson’s rho > 0.9), and all variables were included in the distance-based linear model selection procedure. All tests were two-sided and P values < 0.050 considered statistically significant.
Based on above assessments, we provide a project-scale application of assembled accumulation, combustion and EF parameters to assess emissions from fire-prone eligible miombo vegetation types for baseline and realistic fire management scenarios based on available 10-year automated fire mapping derived from MODIS 500 m imagery for a representative site in northern Zambia.
In this assessment, we have adopted 100-year Global Warming Potentials (GWPs) relying on values derived from the IPCC AR5 (Fifth Assessment Report) without accounting for climate–carbon feedback mechanisms. This approach provides a standardised and widely accepted framework for evaluating the environmental impact of GHG emissions. When accounting for climate–carbon feedback mechanisms, it has been determined that CH4 has a Global Warming Potential (GWP100) of 34, whereas N2O exhibits a GWP100 of 298, as reported by the IPCC AR5 (Myhre et al. 2014).
CO is typically omitted from calculations, despite its role in extending the atmospheric lifespan of CH4 and its contribution as a precursor to O3 (Goodwin et al. 2019). Assessments of CO’s Global Warming Potential (GWP100) differ, ranging from 1.8 (as per Fry et al. 2012) to 5.4 when considering both primary and secondary aerosol effects, as outlined by Shindell et al. (2009). The IPCC AR5 lists a wide range of GWPs. The value of 2.2 is at the bottom of that range and therefore a conservative estimate. For CO and CH4, the GWP100 reported by the IPCC AR5 does not include the radiative forcing of the produced CO2 once oxidised (e.g. 1.6 g of CO2 for each gram of CO emissions). Therefore, like with CO2, these values assume all indirect CO2 emissions are compensated for in the short term through photosynthesis on regrowth.
Results
Woody vegetation structural and floristic relationships
Sampled miombo woodland (WDL) and open woodland (OWDL) woody structural attributes are summarised in Table 3 for two MAR classes, following conventional partition of Wet Miombo and Dry Miombo types under >1000 and ~600–1000 mm MAR conditions, respectively (e.g. White 1983; Frost 1996). Under higher MAR conditions, mean WDL structural attributes were observed to be taller, along with substantially larger tree stem densities (including stems >20 cm DBH), shrub densities (especially <50 cm tall), BA and number of species per plot. Although no significantly fire-impacted OWDL miombo vegetation was sampled under >1000 mm MAR conditions, mean OWDL structural attributes in <1000 mm MAR samples were typically substantially less than for equivalent WDL vegetation, notably save relatively taller tree heights and densities of shrubs >100–200 cm tall.
Attribute | 600–1000 mm MAR | >1000 mm MAR | ||
---|---|---|---|---|
OWDL | WDL | WDL | ||
(n = 38) | (n = 74) | (n = 53) | ||
Trees (≥5 cm DBH) | ||||
13 | 83 | 89 | ||
1.2 ± 0.2 | 5.9 ± 0.6 | 15 ± 0.5 | ||
Stem density (ha−1) | ||||
46 ± 6 | 267 ± 20 | 567 ± 7 | ||
7.5 ± 1.7 | 51.7 ± 4 | 117.4 ± 5.7 | ||
16.3 | 19.3 | 20.7 | ||
Height live stems | ||||
9.4 ± 0.4 | 6.8 ± 0.2 | 11.9 ± 0.1 | ||
12.6 ± 2.2 | 16.1 ± 0.6 | 25.7 ± 0.6 | ||
Basal area (m2 ha−1) | ||||
2.2 ± 0.5 | 7.0 ± 0.7 | 13.9 ± 0.5 | ||
1.9 ± 0.5 | 6.7 ± 0.7 | 13.5 ± 0.5 | ||
86.4 | 95.6 | 97.1 | ||
Shrub (<5 cm DBH) density (0.1 ha−1) | ||||
<50 cm tall | 169 ± 53 | 361 ± 32 | 2004 ± 178 | |
50–100 cm tall | 95 ± 13 | 138 ± 14 | 309 ± 37 | |
>100–200 cm tall | 154 ± 25 | 71 ± 6 | 114 ± 11 | |
>200 cm tall | 28 ± 5 | 24 ± 3 | 125 ± 22 | |
Total | 447 ± 61 | 595 ± 42 | 2538 ± 196 |
Ordination and associated analyses of a floristic dataset comprising tree species sampled at five or more of 160 plots illustrated that sampled vegetation strongly described a floristic continuum corresponding closely with the rainfall gradient, significantly positively correlated with CCI and BA structural variables, and negatively with FF (Fig. 3, Supplementary Fig. S2 and Table S2). Typical miombo tree species (Brachystegia longifolia, B. spiciformis, Dalbergiella nyasae, Julbernardia paniculata, Pterocarpus angolensis) were representative of higher MAR conditions, whereas Amblygonocarpus andongensis, Baikiaea plurijuga and the tall shrub to small tree Baphia massaiensis were representative of lower rainfall conditions (Supplementary Fig. S2a) – notably, all these representative taxa are legumes. dbRDA analysis confirmed that rainfall explained most variability (23%; P = 0.001) in tree assemblages, followed by CCI (19%; P = 0.001), live BA (12%; P = 0.001) and FF (3.6%; P = 0.001) (Fig. 3, Supplementary Fig. S2b). The sampled frequencies of commonly occurring tree species in the three structural MAR classes are given in Supplementary Table S3.
Ordination (dbRDA) based on a distance-based linear model of the relationship between tree floristics assemblages (presence/absence of 62 tree taxa occurring at five or more plots) and predictor variables, at 160 plots. The first axis of the ordination explained 88.5% of the fitted and 23.8% of the total tree floristics variability, and the second axis explained 6% of the fitted and <2% of the total variability. Key: Botswana plots (all <1000 mm MAR): C1W, Chobe site woodland; C1O, Chobe site open woodland; T1W, Tsodilo site woodland; T1O, Tsodilo site open woodland. Zambia plots: Z2W, woodland <1000 mm MAR; Z3W, woodland >1000 mm MAR.
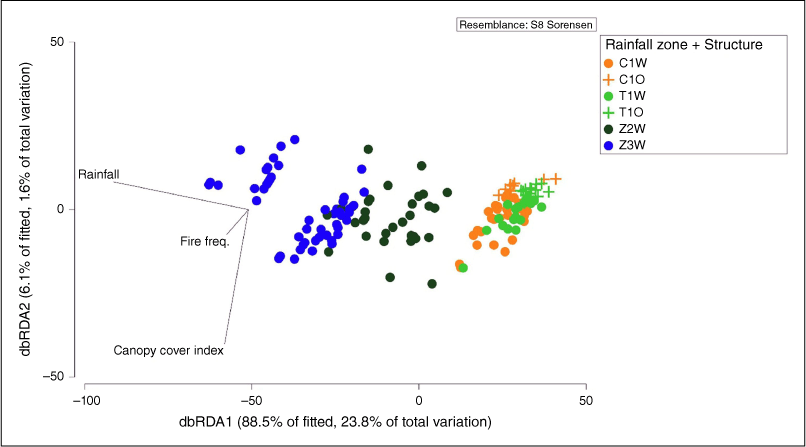
Field observations
Relationships describing accumulation of respective fuel components with time since fire for three vegetation structure–rainfall classes are presented graphically in Fig. 4. Statistically significant accumulation relationships were observed for fine fuels in all three vegetation classes and shrub fuels in WDL classes. Where fuel component accumulation relationships were not found to be statistically significant (P > 0.05), parameter values are given as means (Table 4a) following Russell-Smith et al. (2009) and Yates et al. (2015). Fuel loads in all three vegetation classes were dominated initially by fine fuels and subsequently by shrub fuels, with lesser contributions from coarse and heavy woody fuels. Fine fuels comprised mostly litter, with an increased proportion of litter in LDS samples, in all three vegetation classes (Table 5).
Relationship between accumulation of respective fuel components (a–c) and time since fire, in three vegetation structure–rainfall classes. The regression lines, P values and R2 values refer to the results of linear regressions of fuel load (natural log-transformed) vs time since fire (natural log-transformed). Refer to text for additional details.
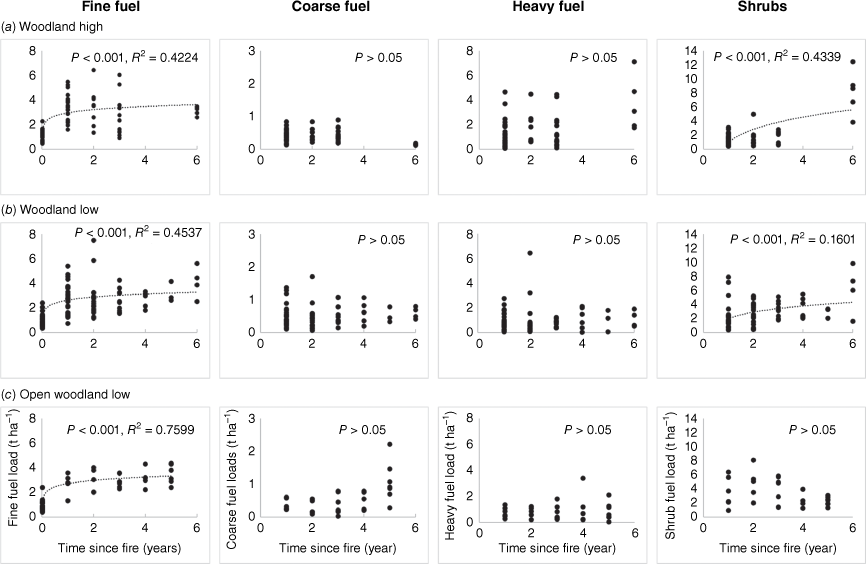
Parameters and variables | 600–1000 mm MAR | >1000 mm MAR | Notes | |||||
---|---|---|---|---|---|---|---|---|
OWDL | WDL | WDL | ||||||
(a) Fuel accumulation (t ha−1) | ||||||||
y = 0.2137x + 0.8822 | y = 0.209x + 0.8248 | y = 0.1877x + 0.9501 | Significant linear relationships of natural log-transformed data; refer to Fig. 4 | |||||
EDS | LDS | EDS | LDS | EDS | LDS | Seasonal litter contributions adjusted for by difference in the proportions of litter: fine fuels determined respectively from EDS and LDS field samples; refer to Methods | ||
1 year since last fire | 2.27 | 2.56 | 2.16 | 2.40 | 2.42 | 2.75 | ||
2 years | 2.63 | 2.97 | 2.50 | 2.78 | 2.75 | 3.14 | ||
3 years | 2.87 | 3.24 | 2.72 | 3.02 | 2.97 | 3.39 | ||
4 years | 3.05 | 3.45 | 2.88 | 3.21 | 3.14 | 3.57 | ||
5 years | 3.20 | 3.61 | 3.02 | 3.36 | 3.27 | 3.73 | ||
6+ years | 3.33 | 3.76 | 3.14 | 3.50 | 3.38 | 3.86 | ||
3.37 ± 0.10 (29) | y = 0.5108x + 0.458 | y = 0.6876x + 0.1275 | Significant linear relationships of natural log-transformed data for WDL classes; refer to Fig. 4 | |||||
1 year since last fire | 1.58 | 1.14 | ||||||
2 years | 2.25 | 1.83 | ||||||
3 years | 2.77 | 2.42 | ||||||
4 years | 3.21 | 2.95 | ||||||
5 years | 3.60 | 3.44 | ||||||
6 +years | 3.95 | 3.89 | ||||||
0.55 ± 0.08 (30) | 0.52 ± 0.04 (68) | 0.39 ± 0.03 (50) | No significant linear relationships; refer to Fig. 4 | |||||
0.91 ± 0.13 (32) | 0.99 ± 0.11 (76) | 1.6 ± 0.21 (53) | No significant linear relationships; refer to Fig. 4 | |||||
(b) Consumption (%) | ||||||||
Early Dry Season | ||||||||
65.94 ± 2.49 (44) | 63.78 ± 2.78 (53) | 33.62 ± 10.35 (8) [59.82 ± 3.03 (61)] | Combining WDL observations given apparently anomalous mean and low n | |||||
4.90 ± 2.00 (31) | 5.65 ± 1.58 (48) | 4.65 ± 0.49 (8) [5.42 ± 1.53 (56)] | Combining WDL observations given low n | |||||
10.18 ± 1.80 (44) | 6.09 ± 1.40 (57) | 3.49 ± 1.47 (8) [5.77 ± 1.23 (65)] | Combining WDL observations given apparently anomalous mean and low n | |||||
1.05 ± 0.52 (43) | 0.61 ± 0.40 (57) | 0.00 ± 0.00 (8) [0.51 ± 0.35 (65)] | Combining WDL observations given apparently anomalous mean and low n | |||||
Late Dry Season | ||||||||
76.42 ± 3.97 (13) | 70.00 ± 2.57 (51) | 73.04 ± 1.32 (73) | ||||||
9.33 ± 2.89 (13) | 18.90 ± 3.82 (40) | 13.76 ± 1.96 (78) | ||||||
19.23 ± 4.68 (14) | 21.18 ± 2.40 (50) | 8.65 ± 1.00 (77) | ||||||
23.33 ± 8.77 (14) | 8.73 ± 2.25 (50) | 10.37 ± 1.90 (78) | ||||||
(c) Burnt patchiness (%) | ||||||||
Early dry season | 67.54 ± 3.45 (44) | 68.08 ± 3.26 (57) | 39.93 ± 6.03 (8) [64.62 ± 3.16 (65)] | Combining WDL observations given apparently anomalous mean and low n | ||||
Late dry season | 81.89 ± 4.03 (14) | 94.28 ± 1.39 (50) | 96.32 ± 0.69 (78) | |||||
(d) C and N content (%) | Refer to Russell-Smith et al. (2021) | |||||||
Early Dry Season | ||||||||
49.01 ± 0.25 (12) | 43.48 ± 2.73 (10) | 45.43 ± 2.73 (13) | ||||||
0.020 ± 0.00 (12) | 0.015 ± 0.005 (10) | 0.014 ± 0.005 (13) | ||||||
Late Dry Season | ||||||||
48.59 ± 0.04 (14) | 46.44 ± 0.06 (31) | 46.28 ± 0.37 (22) | ||||||
0.020 ± 0.00 (14) | 0.020 ± 0.00 (31) | 0.012 ± 0.001 (22) | ||||||
(e) Emission factors | Refer to Vernooij et al. (2023) | |||||||
Early Dry Season | ||||||||
0.19 ± 0.04 (2) | 0.25 ± 0.02 (5) | 0.26 ± 0.02 (11) | ||||||
0.60 ± 0.05 (2) | 0.45 ± 0.08 (5) | 0.43 ± 0.08 (11) | ||||||
5.0 ± 0.8 (2) | 5.8 ± 0.2 (5) | 5.9 ± 0.4 (11) | ||||||
Late Dry Season | ||||||||
0.18 ± 0.01 (5) | 0.31 ± 0.02 (16) | 0.35 ± 0.02 (10) | ||||||
0.70 ± 0.11 (5) | 0.45 ± 0.05 (16) | 0.54 ± 0.06 (10) | ||||||
4.8 ± 0.2 (5) | 5.8 ± 0.4 (16) | 6.6 ± 0.4 (10) | ||||||
(f) Global Warming Potential | ||||||||
34 | 34 | 34 | Refer to IPCC AR5 (Myhre et al. 2014) | |||||
298 | 298 | 298 | Refer to IPCC AR5 (Myhre et al. 2014) | |||||
2.2 | 2.2 | 2.2 | Chosen by us as a conservative estimate; refer to text for details |
Errors given as s.e.m.; n given in parentheses.
Sample | Mean litter fraction in fine fuels (%) | |||
---|---|---|---|---|
OWDL (<1000 mm MAR) | WDL (<1000 mm MAR) | WDL (>1000 mm MAR) | ||
EDS | 55.3 (24) | 64 (52) | 65.7 (22) | |
LDS | 75.5 (8) | 78.1 (24) | 85 (31) | |
Annual | 59.8 (32) | 67.3 (76) | 76.4 (53) |
For WDL classes, total fuels increased from 5.5 to 9.0 and 5.9 to 9.7 t ha−1 after 6 years in Dry Miombo (<1000 mm MAR) and Wet Miombo (>1000 mm MAR) samples respectively, especially associated with developing shrub fuels. OWDL fuels exhibited limited accumulation with time since fire, from 7.4 to 8.6 t ha−1, attributable to relatively large sampled shrub fuel loads in years immediately following fire (Table 4a).
Observations of fire severity treatments at a total of 240 transects illustrated that 89% of EDS fires were of low severity (<2 m scorch height) whereas, conversely, 89% of LDS fires were either of moderate or high severity (Table 6). Overall, fire treatments were least severe in OWDL and most severe in Wet Miombo WDL.
Vegetation class | Fire severity | ||||||||||
---|---|---|---|---|---|---|---|---|---|---|---|
L | M | H | L | M | H | L | M | H | Total | ||
EDS | LDS | Total | |||||||||
(a) Frequency (n) | |||||||||||
OWD <1000 mm | 35 | 4 | 1 | 6 | 5 | 0 | 41 | 9 | 1 | 51 | |
WDL <1000 mm | 52 | 6 | 1 | 6 | 19 | 22 | 58 | 25 | 23 | 106 | |
WDL >1000 mm | 9 | 0 | 0 | 3 | 30 | 41 | 12 | 30 | 41 | 83 | |
Total | 96 | 10 | 2 | 15 | 54 | 63 | 111 | 64 | 65 | 240 | |
(b) Proportion (%) | |||||||||||
OWD <1000 mm | 79.5 | 9.1 | 2.3 | 54.5 | 45.5 | 0.0 | 80.4 | 17.6 | 2.0 | ||
WDL <1000 mm | 88.1 | 10.2 | 1.7 | 12.8 | 40.4 | 46.8 | 54.7 | 23.6 | 21.7 | ||
WDL >1000 mm | 100.0 | 0.0 | 0.0 | 4.1 | 40.5 | 55.4 | 14.5 | 36.1 | 49.4 | ||
Total | 88.9 | 9.3 | 1.9 | 11.4 | 40.9 | 47.7 | 46.3 | 26.7 | 27.1 |
Assembled observations illustrate that EDS fire treatments consistently resulted in less combustion of fuel components (Table 4b) and burnt patchiness (Table 4c) than LDS fires in respective miombo vegetation fuel types. Despite this, substantial variability is evident in comparative mean values of respective fuel components under different seasonal fire treatment conditions, given both fairly large numbers of observations (n; e.g. consumption of shrubs, heavy woody fuels in LDS fire treatments) and small n especially (e.g. EDS fire treatments in Wet Miombo).
EF measurements of CH4, N2O, CO were generally comparable between EDS and LDS samples in respective OWDL and both WDL classes, exhibiting small increases in LDS EFs of CH4 in both WDL classes, and EFs of N2O and CO in WDL >1000 mm MAR (Table 4e). Significant differences (two-tailed t-tests; P < 0.1) were observed only with seasonal differences in EFs of CH4 for WDL >1000 mm MAR, and respective EFs between OWDL and both WDL classes – likely attributable to the fairly small number of fires measured in this study impacting the ability to detect significant differences between seasonal subcategories. These EF findings corresponded with increased combustion of all fuel components in LDS fires compared with EDS fires (Table 4b). Sampled N:C ratios were generally similar in EDS and LDS fine fuel samples in all three vegetation classes, albeit increasing slightly in lower-rainfall WDL and declining slightly in higher-rainfall WDL in the LDS (Table 4d).
Fire management and emissions scenarios
We applied assembled accumulation, combustion and EF parameters to a 2716 km2 regionally characteristic case study site with a recent (last 10 years) fire history dominated by LDS fires (annual mean 35.6%) and little prescribed EDS burning (annual mean 3.2%). Based on emissions calculations for 2022 (Supplementary Fig. S3), GHG emissions from EDS fires are approximately half (56%) those of LDS fires in OWDL, and one-third (34%) those in both WDL types (Table 7). Under enhanced EDS fire management, emissions reductions of between 30 and 40% are realistically achievable in respective vegetation structural types under fire frequencies of 20% prescribed EDS fire and 20% LDS fire, and 36–50% emissions reductions with 25% EDS and 10% LDS fire frequencies (Table 7).
Criteria | Area burnt | Emissions (t CO2-e) | Reduction vs baseline (%) | ||||||
---|---|---|---|---|---|---|---|---|---|
EDS | LDS | EDS | LDS | Total | |||||
(km2) | (%) | (km2) | (%) | ||||||
Baseline emissions 2012–2022 | |||||||||
OWDL | 85.9 | 3.2 | 967.4 | 35.6 | 792.0 | 15933.1 | 16725.1 | ||
WDL <1000 mm | 85.9 | 3.2 | 967.4 | 35.6 | 575.5 | 18970.1 | 19546.2 | ||
WDL >1000 mm | 85.9 | 3.2 | 967.4 | 35.6 | 652.8 | 21544.0 | 22196.8 | ||
Emissions km−2 | |||||||||
OWDL | 9.22 | 16.47 | |||||||
WDL <1000 mm | 6.70 | 19.61 | |||||||
WDL >1000 mm | 7.6 | 22.27 | |||||||
Scenario 1 | |||||||||
OWDL | 536.9 | 20.0 | 407.6 | 15.0 | 4950.2 | 6713.2 | 11663.4 | 30.3 | |
WDL <1000 mm | 536.9 | 20.0 | 407.6 | 15.0 | 3597.2 | 7993.0 | 11590.3 | 40.7 | |
WDL >1000 mm | 536.9 | 20.0 | 407.6 | 15.0 | 4080.4 | 9077.3 | 13157.7 | 40.7 | |
Scenario 2 | |||||||||
OWDL | 671.0 | 25.0 | 271.7 | 10.0 | 6186.6 | 4474.9 | 10661.5 | 36.3 | |
WDL <1000 mm | 671.0 | 25.0 | 271.7 | 10.0 | 4495.7 | 5328.0 | 9823.7 | 49.7 | |
WDL >1000 mm | 671.0 | 25.0 | 271.7 | 10.0 | 5099.6 | 6050.8 | 11150.4 | 49.8 |
Refer to Supplementary Fig. S3 for calculation details.
Discussion
Emissions abatement framework
Building on earlier technical exploration of the feasibility of applying a savanna fire management emissions abatement methodology derived from Australian conditions to fire-prone southern African woody savanna sites at Tsodilo, northern Botswana, and Niassa, northern Mozambique (Russell-Smith et al. 2021), the present paper extends that assessment towards developing a robust emissions accounting framework pertinent to expansive woodlands covering ~2 million km2 of the southern African miombo ecoregion. Based on Australian precedent (CoA 2015), our proposed framework is Tier 2 (IPCC 2000), empirically derived, comprising spatially stratified and disaggregated parameters (e.g. different vegetation fuel types, fuel components, combustion and emissions under different seasonal conditions). One advantage of such an essentially bottom–up Tier 2 approach is that emissions calculation components and processes are readily transparent; by contrast, a Tier 3 approach typically involves complex, non-linear carbon cycle modelling such as will be applied in the next generation of Australian savanna burning methods, due from 2024. Although this proposed framework can be applied also to meet national and United Nations Framework Convention on Climate Change (UNFCCC) emissions accounting and reporting commitments as part of the Agriculture, Forestry and Other Land Use (AFOLU) sector, our primary focus here is to address project-scale accounting needs – i.e. to account for mitigated GHG emissions delivered through enhanced site-based fire management activities. Here, we address core components of the proposed miombo emissions accounting framework; limitations and ongoing methodological challenges associated with assembled plot data and derived framework parameters are summarised in Table 8.
Parameter | Project-scale assessment | Additional requirements | |
---|---|---|---|
Burnt Area (BA) mapping |
| ||
Vegetation Fuel Type (VFT) mapping |
|
| |
Fuel accumulation |
|
| |
Combustion |
| ||
Emission factors |
|
Our field program sampled miombo vegetation structural and associated fuel load accumulation, combustion and emissions attributes, focused on the recognised central geographic miombo floristics and productivity gradient, from low-rainfall conditions in northern Botswana to higher-rainfall sites in north-central Zambia (White 1983; Frost 1996; Godlee et al. 2021). An equivalent centralised rainfall gradient-focused sampling approach was undertaken with developing Australia’s original savanna burning methods (Russell-Smith et al. 2009). Ordination confirmed the strong floristic and associated vegetation structural continuum with the rainfall/productivity gradient described by our assembled common tree species dataset.
Following regional convention (e.g. White 1983; Frost 1996; Timberlake and Chidumayo 2011; Ribeiro et al. 2020b), we have recognised Wet and Dry Miombo fuel types occurring under conditions of >1000 and <1000 mm MAR, respectively. Similarly, in the Australian savanna burning method, respective vegetation fuel types in High and Low Rainfall Zones are delimited by the 1000 mm MAR isohyet. From an emissions methodology perspective, however, definitive geographic separation of Wet and Dry Miombo types presents practical difficulties given the paucity of meteorological stations and associated unreliability of rainfall surfaces (Trisos et al. 2022); here, we have attributed MAR per plot from the widely applied European Centre for Medium-Range Weather Forecasts Copernicus global data set (https://cds.climate.copernicus.eu/). Unlike with the Australian approach (Whitehead et al. 2014), here we do not define a priori the lower MAR limits applicable to the method; rather, we propose that project proponents should select prospective project areas after assessment of burnt area mapping and associated emissions estimates, and eligible miombo VFTs as described below.
Structurally, we have distinguished betweenWDL and OWDL miombo VFTs where, following Australian convention, OWDL exhibits canopy cover <20% and WDL >20% (CoA 2012; Fig. 5). Unlike for Australian savannas, we do not include an Open Forest class (OF canopy cover >50–80%) given that southern African studies report that fire occurrence is effectively truncated where percentage tree cover exceeds ~40% (Scholes 2003; Archibald et al. 2009). We note, however, that assessments reported by Archibald et al. (2009, 2010) were undertaken with burnt area mapping products derived from fairly coarse-scale MODIS 500 m imagery, which, subsequently, has been shown to significantly underestimate the extent of especially smaller fires <100 ha in African contexts (Roteta et al. 2019; Ramo et al. 2021). In eucalypt OF savannas, fires occur extensively in litter-dominated fuel loads (e.g. Russell-Smith et al. 2009).
Miombo Vegetation Fuel Types on typical sandy substrates: (a) deciduous miombo WDL, Kasane Forest Reserve, Botswana – LDS, 680 mm MAR; (b) remnant fire-affected shrubby OWDL, Tsodilo Hills World Heritage Site, Botswana – EDS, 640 mm MAR; (c) shrubby OWDL, north-west Ngamiland, Botswana – LDS, 660 mm MAR; (d) miombo WDL, Kafue National Park, Zambia – EDS, 970 mm MAR; deciduous miombo WDL, Chisamba, Zambia – LDS, 950 mm MAR; (f) miombo WDL, Lualaba Forest Reserve, Zambia – EDS, 1340 mm MAR; (g) semi-deciduous miombo WDL, East Lunga Forest Reserve, Zambia – LDS, 1100 mm MAR.
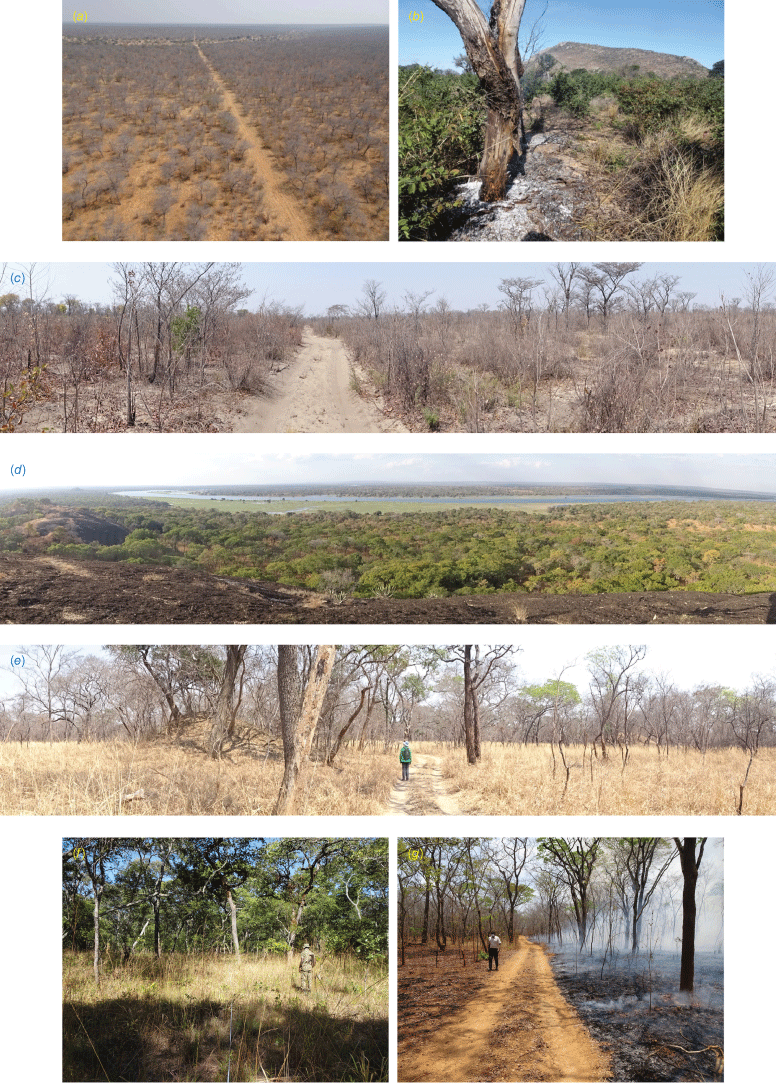
To distinguish between OWDL and ineligible grassland types, we adapted the definition of White (1983) that eligible OWDL should comprise at least 10% cover of typical miombo tree and shrub taxa. Data presented in Table 3 illustrate that OWDL plot samples typically supported (with 95% confidence) >30 tree stems ha−1, and >1000 shrubs taller than 1 m ha−1.
All OWDL plots sampled in our assessment were located under <1000 mm MAR conditions, typically comprising fire-disturbed shrubby vegetation with sparse tree stem recruitment. Although we did not sample heavily disturbed fire- and agriculturally affected (e.g. slash and burn) miombo sites under higher rainfall conditions, for example severely fire-disturbed early successional chipya vegetation types in northern Zambia (Lawton 1964, 1978), disturbed miombo vegetation is widespread and rapidly expanding (Syampungani et al. 2020; Zulu et al. 2020). For present methodological purposes, we suggest that emissions accounting parameters determined for disturbed OWDL sites under <1000 mm MAR conditions apply also to higher-rainfall OWDL sites provided that such sites meet essential eligibility criteria including the potential for functional miombo vegetation regeneration from extant root sucker and coppice resources (Stromgaard 1985; Frost 1996; Chidumayo 1997a, 2004, 2013; Williams et al. 2008; Syampungani et al. 2016). Although further assessment is evidently warranted (Table 8), such an approach follows conservative emission accounting principles given observed enhanced productivity, including of grass fuels in disturbed sites (Chidumayo and Kwibisa 2003), under higher rainfall conditions.
Sampling of fuel loads and fuel combustion was undertaken under ambient grazing, browsing, termite harvesting conditions mostly in forest and conservation reserves that included substantial herds of larger ungulates and elephants, especially in the Chobe region (Botswana) and Kafue NP (Zambia). Similarly, field sampling in Australia was conducted under ambient grazing, browsing, termite harvesting conditions (avoiding heavily impacted sites) involving macropods, cattle and Asian water buffalo. Fuel loads comprised mostly fine fuels and shrubs in all three miombo VFTs (WDL > 1000 mm MAR; WDL and OWDL < 1000 mm MAR), with the proportion of shrub fuels increasing with time since fire (TSF) in WDL types and exhibiting no relationship with TSF in OWDL. The contribution of mean shrub fuels in miombo fuel types is three to four times greater than that observed for comparable VFTs in Australian savannas, for example: 3.37 t ha−1 in OWDL vs 1.12 t ha−1 OWLMixed grasses (Yates et al. 2015); 2.23 t ha−1 in WDL > 1000 mm MAR vs 0.5 t ha−1 WLMixed grasses (Russell-Smith et al. 2009). Other than for shrubby fuels, means of other fuel load components are observed to be more or less comparable in the two savanna systems, with the exception of substantially greater (2 t ha−1) fine fuels in higher-rainfall eucalypt woodlands. Overall, mean fuel loads (unadjusted for seasonal inputs) in comparable miombo and eucalypt savanna VFTs are as follows: 7.9 t ha−1 in OWDL vs 5.86 t ha−1 OWLMixed grasses (Yates et al. 2015); 7.2 t ha−1 in WDL <1000 mm MAR vs 5.9 t ha−1 WLMixed grasses (Yates et al. 2015); 7.4 t ha−1 in WDL >1000 mm MAR vs 8.9 t ha−1 WLMixed grasses (Russell-Smith et al. 2009).
Despite grassy fuels being considered the archetypic fuel type of savannas (Scholes and Archer 1997) and recognition that grass production is inversely related to woody cover in more mesic savannas (Cook 2003; Scholes 2003; Dohn et al. 2013), fine fuel loads were dominated by leaf litter in all three miombo VFTs. By contrast, litter fuels are the dominant component of fine fuels only in more wooded eucalypt VFTs (Russell-Smith et al. 2009; Yates et al. 2015). Litter inputs in miombo systems exhibit strong seasonality, especially under more xeric conditions, including OWDL with extensive deciduous shrubby components (Malaisse et al. 1975; Frost 1996; Ribeiro et al. 2013; Russell-Smith et al. 2021). Such seasonal litter inputs have significant implications for resultant fire intensities and, as noted below, EFs. In the absence of empirical seasonal litter accession data, following Australian precedent (Russell-Smith et al. 2009; Yates et al. 2015), here, we have accounted for increased LDS litter fuels by adjusting the difference in the proportions of litter:fine fuels determined respectively from EDS and LDS field samples. This adjustment is likely very conservative and warrants further field assessment under different rainfall/productivity gradient conditions (Table 8). By contrast, grassy fuel load components, once cured, may be anticipated to be aseasonal except where extensively grazed or consumed by termites (Scholes et al. 1996; Hély et al. 2003). Given the absence of apparent accumulation relationships between CWD and HWF with TSF, respective inputs are most likely associated with stochastic events such as severe wet season storms and fire events (Yates et al. 2020) and, in African contexts, destructive impacts of large animals such as elephants (Mosugelo et al. 2002; Ribeiro et al. 2008).
Fuel combustion and associated fire patchiness characteristics were observed to be strongly related to fire severity, and thereby seasonal conditions, as widely reported in the savanna literature (e.g. Frost and Robertson 1987; Shea et al. 1996; Chidumayo 1997b; Williams et al. 1998; Russell-Smith et al. 2009; Oliveira et al. 2015). Major contributions to resultant quantities and seasonal differences in emissions are associated especially with consumption of fine fuels and burnt patchiness (Table 4). Although acknowledging that imposed experimental fire treatments were designed to emulate prescribed low-severity EDS and LDS wildfire conditions respectively, the resultant fire severity frequency distributions of both are generally similar to those observed under Australian ambient savanna fire regime conditions, for example: miombo – EDS 89% low severity, LDS 89% moderate + high severity (Table 6); eucalypt – EDS 74% low severity, LDS 84% moderate + high severity (Edwards et al. 2015). Such observations reinforce the practical feasibility of implementing prescribed strategic EDS fire management where appropriate.
In the absence of current means for reliably mapping fire severity or intensity at appropriate spatial and temporal scales to enhance combustion estimates, for convenience we have applied the end of July as a default cut-off between the EDS and the LDS. Although this suffices for our study sites given the seasonal distribution of fire severity observations, we recognise that some variation may be appropriate to cater for regional differences, e.g. for parts of northern Angola and southern Mozambique where the driest 6-month period commences 1 month earlier (Russell-Smith et al. 2021, fig. 1c).
Our methodological approach addressing combustion calculations differs in two notable, albeit probably quantitatively minor, regards to that applied in previous Australian assessments. First, here we have applied EDS and LDS combustion parameters representing the mean of respective EDS and LDS treatment observations, whereas in Australian assessments, EDS and LDS combustion parameters account for the seasonal proportions of low, moderate and severe fires as observed at long-term, ambient fire regime monitoring plots (Russell-Smith and Edwards 2006; Edwards et al. 2015). Second, for combustion calculation purposes, here we have not distinguished between unburnt and typically very small quantities of burnt residual materials (e.g. ash, char fractions) in post-fire sample quadrats but, rather, combined both on the basis that (a) experience with separating the two components in field assessments has proved practically difficult, and (b) resultant gaseous emissions estimates are therefore conservative.
The limited seasonal fluctuations of EFs observed here in seasonally dry woodlands are consistent with previous studies from northern Australian savanna (Meyer et al. 2012), miombo types (Hoffa et al. 1999; Russell-Smith et al. 2021) and Brazilian cerrado (Vernooij et al. 2021). The increase of CH4 EF in both miombo WDL types in the LDS is consistent with findings from Brazilian cerrado (Vernooij et al. 2021). This, and observed LDS increases also of N2O and CO EFs, may be related to a larger contribution of smouldering combustion-prone fuels (e.g. leaf litter) in the LDS, combined with more efficient combustion of litter and grass. Importantly, these results reinforce earlier observations (Russell-Smith et al. 2021) that prescribed EDS fire management undertaken in cured miombo fuels affords conservative emissions benefits.
To compare our EF measurements with previous literature, here we adopt the widely used format of grams emitted per kilogram of dry matter consumed, departing from the percentage-based representation given in Table 4. Our EFs were computed using the carbon mass balance methodology, following Vernooij et al. (2023). In OWDL, our CH4 and CO EFs were slightly lower than global savanna averages; EF(CH4) = 1.85 vs 1.22 (EDS), 1.45 (LDS); EF(CO) = 64 vs 57 (EDS), 55 (LDS). In both WDL types, our values exhibited a nuanced temporal pattern, with lower EFs during the EDS and higher EFs during the LDS.
Conversely, for N2O, compared with the global savanna average EF of 0.16 (Vernooij et al. 2023), EFs exhibited slightly higher values in OWDL (0.24 EDS; 0.3 LDS) albeit from low numbers of observations, and lower values in WDL types (<1000 mm MAR: 0.09 EDS, 0.1 LDS; >1000 mm MAR: 0.1 EDS, 0.11 LDS). Although our study provides fixed EFs for EDS and LDS fires, EFs are influenced by various factors including fuel characteristics like moisture content and deciduous litter accumulation, and meteorological variables such as wind speed and humidity, changing progressively throughout the fire season (Vernooij et al. 2023).
Application of the proposed fire management emissions abatement framework to a realistic project-scale case study illustrates that EDS prescribed fire management can substantially reduce LDS emissions in all three miombo VFTs (Table 7, Supplementary Fig. S3). In Australian projects, net emissions from EDS fires have been shown to be ~50% less per unit area than LDS fires under high rainfall conditions (Russell-Smith et al. 2009), and ~25% under lower rainfall conditions (Russell-Smith et al. 2015). Given current predominance of LDS fires in miombo systems, it is realistically feasible to significantly reduce both LDS and annual BA extent and realise a variety of climate change, hydrological, ecological, grazing management and socio-economic benefits (e.g. McNaughton 1985; Chidumayo 1997a; Ribeiro et al. 2020a; Trisos et al. 2022).
Complementary approaches
In addition to abatement of GHG emissions, strategic prescribed EDS fire management projects can also deliver significant sequestration in miombo woody biomass and possibly SOC. With respect to woody biomass sequestration, it is widely observed that fire regimes dominated by fairly intense fires have significant destructive impacts on miombo woodland systems, especially under more xeric conditions (e.g. Trapnell 1959; Frost 1996; Chidumayo 1997b; Holdo 2005; Ryan and Williams 2011; Ribeiro et al. 2020b, 2021; Cassidy et al. 2022). As such, there is considerable opportunity for developing complementary sequestration methods. To date, two studies have assessed the effects of fire regimes on woody tree biomass sequestration potential in more or less intact miombo systems, both based on gap phase modelling simulating photosynthesis and carbon allocation processes: one predicting that annual burning (of whatever intensity) would result in almost total loss of tree biomass (Ryan and Williams 2011); the other predicting that annual fires (based on an ambient fire regime dominated by LDS fires) would result in woody biomass stabilising at a level substantially less than that under no burning (Ribeiro et al. 2021). Further assessment of the sequestration effects (and benefits) of low-severity fire regimes is warranted, however, given contrasting results from a long-term replicated fire experiment conducted in miombo woodland at Ndola (now Zambia) where it was observed that annual EDS low-intensity fires resulted in negligible stem mortality, similar to that observed on unburnt plots (Trapnell 1959).
Significant opportunities for relatively rapid woody biomass sequestration have been identified in studies of miombo regeneration following clearing and cutting in agricultural settings. Given availability of substantial miombo root sucker and coppice recruitment stocks following initial clearing activities, and low-intensity disturbance impacts especially from infrequent burning thereafter, re-establishment of site woody biomass can be attained within as little as a few decades (e.g. Chidumayo 1997a, 1997b, 2019; Williams et al. 2008; Gumbo et al. 2018). As Syampungani et al. (2020) observe, such vigorous regeneration potential on degraded sites offers substantially enhanced carbon sequestration opportunities compared with essentially moribund old-growth stands.
Sequestration of SOC also has been shown to have possible, if variable, potential in southern African savanna systems under conditions of: (a) longer-term (multi-decadal) absence of, or infrequent, low-intensity burning; (b) commensurate maintenance of tree canopy cover to provide sustained litter inputs in addition to inputs from grasses; (c) in soils with higher clay contents (Bird et al. 2000; Williams et al. 2008; Holdo et al. 2012; Pellegrini et al. 2018, 2020; Zhou et al. 2022; Coetsee et al. 2023). SOC sequestration potential in sandy miombo soils with characteristically very low organic content and base-exchange capacity is further limited given the complementary impacts of recurrent fires and intense harvesting on leaf litter inputs (Trapnell et al. 1976; Frost 1996). In contrast to woody sequestration potential, this applies also to cultivated sites given very slow rates of identifiable SOC accumulation following agricultural abandonment, especially when associated with ongoing fire disturbance (Chidumayo and Kwibisa 2003; Walker and Desanker 2004; Williams et al. 2008). Based on evidence from a multi-decadal study of SOC sequestration potential under different experimental fire regimes, Coetsee et al. (2023) conclude that frequent low-severity fires offer a conservative management approach – at least in habitat conditions not undergoing detrimental woody encroachment.
Prescribed fire management emissions abatement projects can also complement other developing and potential market-based NBS (Nature-Based Solutions) and PES (Payments for Environmental Services) approaches applicable to miombo systems. The most established of these, albeit at early stages of development in different southern African countries, concerns REDD+ projects that aim to Reduce Emissions from Deforestation and forest Degradation through sustainable management of forests and sequester carbon for climate-change mitigation. Although Syampungani et al. (2020) note the substantial potential of REDD+ for ameliorating miombo degradation and community benefit, to date there have been mixed results and emerging governance concerns, including reliability of measurement and monitoring processes, and equitable economic benefits for participating communities (Zulu et al. 2020). Current experience with a foundational REDD+ project at Kariba, Zimbabwe, highlights both these challenges and, in the context of the present study, the importance of the integrity of certification schemes (Blake 2023).
Applying the framework
Credible carbon emissions markets are reliant on generating high-integrity, transparently accounted credits. Incorporation of our proposed miombo fire management emissions abatement framework within a formal accreditation process, or Standard, potentially can involve application of two (non-exclusive) pathways: registering a project under a voluntary carbon market standard; utilising emerging bilateral (or plurilateral) government and private sectors arrangements under especially Article 6.2 of the Paris Agreement (Minas 2022). An instructive example of the former approach is provided by the Nakau programme, which utilises a commercial certification scheme to underpin community-owned, high-integrity carbon projects in the Pacific that support nature-based, cultural and climate resilience values (https://nakau.org). The second pathway draws on experience with Australian Government and UNFCCC-sanctioned savanna burning projects as a basis for establishing ethical terms and responsibilities in contractual agreements between participating communities, governments and purchasers of carbon credits, with an annex that outlines the relevant Standard to be applied for the project.
Application of appropriate emissions accounting methods and robust Standards is reliant also on independent MRV (Monitoring, Reporting, Verification) processes and structures, ideally servicing both national (e.g. Nationally Determined Commitments to the UNFCCC under the Paris Agreement) and linked project-scale requirements. In Australia, the regulated savanna burning MRV system, SAVBAT (https://savbat.environment.gov.au), currently fulfils both roles, where mitigation achieved through emissions abatement projects links directly with and is accounted for in Australia’s National Greenhouse Gas Inventory. Development of a Tier 2 MRV system for REDD+ applications in southern African countries has been initiated through a partnership involving SADC (Southern African Development Commission) and the German development agency GIZ (Deutsche Gesellschaft für Internationale Zusammenarbeit).
As identified in the introductory remarks, there are two significant types of caveats that need to be considered when implementing incentivised savanna burning projects. First, application of a fairly conservative, low-severity EDS fire management approach is not appropriate in grassland situations at risk of exacerbated woody encroachment and associated loss of habitat and dependent biodiversity (e.g. Parr et al. 2014; Veldman et al. 2015). However, strategically undertaken savanna burning projects can provide a funded, operational framework to assist with delivering a range of objectives including, for example, safe application of intense fires in a matrix of reduced fuel loads for woody plant control and maintaining open grassy habitats (Edwards et al. 2021). Second, as cautioned in related studies (e.g. ISFMI 2015; Russell-Smith et al. 2013b, 2021; Zulu et al. 2020), there are a variety of significant economic, societal and political implementation challenges that require informed and sustained consideration if the benefits of market-based carbon offset projects are to be realised. We recognise that addressing both these conditional challenges requires considered site-based assessment.
Data availability
Datasets that support this study will be shared upon reasonable request to the corresponding author. Note that the full data will be made available online as an annex to any developed methods Standard.
Conflicts of interest
The authors declare they have no conflict of interest. Jeremy Russell-Smith and Roland Vernooij are guest editors of this special issue of the International Journal of Wildland Fire. To mitigate this potential conflict of interest, they were blinded from the review process.
Declaration of funding
Core funding for the work presented here was provided by grants from Australia’s Department of Foreign Affairs (DFAT), Corporate Carbon (CC) and the Green Climate Fund (GCF). Additionally, RV and TE were supported by grants from KNAW AMMODO and the Netherlands Organisation for Scientific Research (NWO).
Acknowledgements
Research in Botswana was undertaken under Research Permit ENT 8/36/4 XLVI (91), supported by the Botswana Savanna Burning Pilot Study Agreement between the Governments of Botswana and Australia, implemented through Botswana’s Department of Forestry & Range Resources (DFRR), and the Australian-based NGO the International Savanna Fire Management Initiative (ISFMI). Research in Zambia was supported by sequential research permits organised through the Departments of Forestry (DF) and National Parks and Wildlife (DNPW). We acknowledge the great deal of assistance provided by (1) Community Trust members in Botswana, (2) the University of Botswana’s Okavango Research Institute, especially technical expertise provided by Dr Richard Fynn and Frances Murray-Hudson; (3) Florence Banda, Thecla Masuku and many DF and DNPW field staff in Zambia, and the ongoing support of the National Designated Authority, Francis Mpampi; (4) ISFMI/Charles Darwin University colleagues Andrew Edwards, Catherine Monagle, Sam Johnston, Sean Lange, Chad Leavitt; (5) and funding support through Australia’s DFAT, GCF, CC and ISFMI. Thank you.
References
Abreu RCR, Hoffmann WA, Vasconcelos HL, Pilon NA, Rossatto DR, Durigan G (2017) The biodiversity cost of carbon sequestration in tropical savanna. Science Advances 3, e1701284.
| Crossref | Google Scholar | PubMed |
Andreae MO (2019) Emission of trace gases and aerosols from biomass burning – an updated assessment. Atmospheric Chemistry and Physics 19(13), 8523-8546.
| Crossref | Google Scholar |
Archibald S, Roy DP, van Wilgen BW, Scholes RJ (2009) What limits fire? An examination of drivers of burnt area in southern Africa. Global Change Biology 15, 613-630.
| Crossref | Google Scholar |
Archibald S, Scholes RJ, Roy DP, Roberts G, Boschetti L (2010) Southern African fire regimes as revealed by remote sensing. International Journal of Wildland Fire 19, 861-878.
| Crossref | Google Scholar |
Bird MI, Veenendaal EM, Moyo C, Lloyd J, Frost P (2000) Effect of fire and soil texture on soil carbon in a sub-humid savanna (Matopos, Zimbabwe). Geoderma 94, 71-90.
| Crossref | Google Scholar |
Blake H (2023) The great cash-for-carbon hustle. The New Yorker Magazine, 16 October 2023. Available at https://www.newyorker.com/magazine/2023/10/23/the-great-cash-for-carbon-hustle
Cassidy L, Perkins J, Bradley J (2022) Too much, too late: fires and reactive wildfire management in northern Botswana’s forests and woodland savannas. African Journal of Range & Forage Science 39, 160-174.
| Crossref | Google Scholar |
Chidumayo EN (1997b) Effects of accidental and prescribed fires on miombo woodland, Zambia. Commonwealth Forestry Review 76, 268-272.
| Google Scholar |
Chidumayo EN (2004) Development of Brachystegia–Julbernardia woodland after clear-felling in central Zambia: evidence for high resilience. Applied Vegetation Science 7, 237-242.
| Crossref | Google Scholar |
Chidumayo EN (2013) Forest degradation and recovery in a miombo woodland landscape in Zambia: 22 years of observations on permanent sample plots. Forest Ecology and Management 291, 154-161.
| Crossref | Google Scholar |
Chidumayo EN (2019) Is charcoal production in Brachystegia-Julbernardia woodlands of Zambia sustainable? Biomass and Bioenergy 125, 1-7.
| Crossref | Google Scholar |
Chidumayo EN, Kwibisa L (2003) Effects of deforestation on grass biomass and soil nutrient status in miombo woodland, Zambia. Agriculture, Ecosystems & Environment 96, 97-105.
| Crossref | Google Scholar |
CoA (Commonwealth of Australia) (2012) ‘Australia – Present Major Vegetation Groups – NVIS Version 4.1 (Albers 100 m analysis product).’ (Department of Environment, Australian Government: Canberra, ACT) Available at http://www.environment.gov.au/metadataexplorer/full_metadata.jsp
CoA (Commonwealth of Australia) (2013) Carbon Credits (Carbon Farming Initiative) Reduction of Greenhouse Gas Emissions through Early Dry Season Savanna Burning – 1.1) Methodology Determination 2013. Available at http://www.comlaw.gov.au/Series/F2013L01165
CoA (Commonwealth of Australia) (2015) ‘Carbon Credits (Carbon Farming Initiative – Emissions Abatement through Savanna Fire Management) Methodology Determination 2015.’ (ComLaw, Australian Government: Canberra, Australia) Available at http://www.comlaw.gov.au/Details/F2015L00344
CoA (Commonwealth of Australia) (2018) ‘Carbon Credits (Carbon Farming Initiative – Savanna Fire Management – Emissions Avoidance) Methodology Determination 2018.’ (Dept of Environment and Energy, Australian Government: Canberra, Australia) Available at https://www.legislation.gov.au/Details/F2018L00560
Coetsee C, February EC, Wigley BJ, Kleyn L, Strydom T, Hedin LO, Watson H, Attore F, Pellegrini A (2023) Soil organic carbon is buffered by grass inputs regardless of woody cover or fire frequency in an African savanna. Journal of Ecology 111, 2483-2495.
| Crossref | Google Scholar |
Dohn J, Dembélé F, Karembé M, Moustakas A, Amévor KA, Hanan NP (2013) Tree effects on grass growth in savannas: competition, facilitation and the stress‐gradient hypothesis. Journal of Ecology 101(1), 202-209.
| Crossref | Google Scholar |
Dziba L, Ramoelo A, Ryan C, Harrison S, Pritchard R, Tripathi H, Sitas N, Selomane O, Engelbrecht F, Pereira L, Katerere Y, Chirwa PW, Ribeiro NS, Grundy IM (2020) Scenarios for just and sustainable futures in the Miombo Woodlands. In ‘Miombo Woodlands in a changing environment: securing the resilience and sustainability of people and woodlands’. (Eds NS Ribeiro, Y Katerere, PW Chirwa, IM Grundy) pp. 191–233. (Springer Nature: Switzerland, AG)
Eames T, Vernooij R, Russell-Smith J, Yates C, Edwards A, van der Werf GR (2023) Seasonal skew of tropical savanna fire regimes. International Journal of Applied Earth Observation and Geoinformation, in press. https://www.sciencedirect.com/journal/international‐journal‐of‐applied‐earth‐observation‐and‐geoinformation/vol/125/suppl/C.
| Google Scholar |
Edwards A, Archer R, De Bruyn P, Evans J, Lewis B, Vigilante T, Whyte S, Russell-Smith J (2021) Transforming fire management in northern Australia through successful implementation of savanna burning emissions reductions projects. Journal of Environmental Management 290, 112568.
| Crossref | Google Scholar | PubMed |
Evans J, Russell-Smith J (2019) Delivering effective savanna fire management for defined biodiversity conservation outcomes: an Arnhem Land case study. International Journal of Wildland Fire 29, 386-400.
| Crossref | Google Scholar |
Fry MM, Naik V, West JJ, Schwarzkopf MD, Fiore AM, Collins WJ, Dentener FJ, Shindell DT, Atherton C, Bergmann D, Duncan BN, Hess P, MacKenzie IA, Marmer E, Schultz MG, Szopa S, Wild O, Zeng G (2012) The influence of ozone precursor emissions from four world regions on tropospheric composition and radiative climate forcing. Journal of Geophysical Research: Atmospheres 117(7), 1-16.
| Crossref | Google Scholar |
Garde M, Nadjamerrek LB, Kolkiwarra M, Kalarriya J, Djandjomerr J, Birriyabirriya B, Bilindja R, Kubarkku M, Biless P (2009) The language of fire: seasonality, resources and landscape burning on the Arnhem Land Plateau. In ‘Culture, ecology and economy of savanna fire management in northern Australia: rekindling the Wurrk tradition’. (Eds J Russell-Smith, PJ Whitehead, PM Cooke) pp. 85–164. (CSIRO Publishing: Melbourne, Vic., Australia)
Giglio L, Boschetti L, Roy DP, Humber ML, Justice CO (2018) The Collection 6 MODIS burned area mapping algorithm and product. Remote Sensing of Environment 217, 72-85.
| Crossref | Google Scholar | PubMed |
Godlee JL, Ryan CM, Bauman D, Bowers SJ, Carreiras JMB, Chisingu AV, Cromsigt JPMG, Druce DJ, Finckh M, Goncalves FM, Holdo RM, Makungwa S, McNicol IM, Mitchard ETA, Muchawona A, Revermann R, Ribeiro NS, Siampale A, Syampungani S, Tchamba JJ, Tripathi HG, Wallenfang J, te Beest M, Williams M, Dexter KG (2021) Structural diversity and tree density drives variation in the biodiversity–ecosystem function relationship of woodlands and savannas. New Phytologist 232, 579-594.
| Crossref | Google Scholar |
Goodwin J, Gillenwater M, Romano D, Radunsky K (2019) Precursors and indirect emissions. In ‘2019 Refinement to the 2006 IPCC Guidelines for National Greenhouse Gas Inventories – General Guidance and Reporting. Vol. 1’. (Eds D Gómez, W Irving) (Institute for Global Environmental Strategies (IGES) for the IPCC) Available at https://www.ipcc.ch/report/2019-refinement-to-the-2006-ipcc-guidelines-for-national-greenhouse-gas-inventories/
Gumbo D, Clendenning J, Martius C, Moombe K, Grundy I, Nasi R, Mumba KY, Ribeiro N, Kabwe G, Petrokofsky G (2018) How have carbon stocks in central and southern Africa’s Miombo woodlands changed over the last 50 years? A systematic map of the evidence. Environmental Evidence 7, 16.
| Crossref | Google Scholar |
Handavu F, Syampungani S, Sileshi GW, Chirwa PWC (2021) Aboveground and belowground tree biomass and carbon stocks in the miombo woodlands of the Copperbelt in Zambia. Carbon Management 12, 307-321.
| Crossref | Google Scholar |
Hély C, Dowty PR, Alleaume S, Caylor KK, Korontzi S, Swap RJ, Shugart HH, Justice CO (2003) Regional fuel load for two climatically contrasting years in southern Africa. Journal of Geophysical Research: Atmospheres 108(D13), 8475.
| Crossref | Google Scholar |
Hoffa EA, Ward DE, Hao WM, Susott RA, Wakimoto RH (1999) Seasonality of carbon emissions from biomass burning in a Zambian savanna. Journal of Geophysical Research: Atmospheres 104(13), 13841-13853.
| Crossref | Google Scholar |
Holdo RM (2005) Stem mortality following fire in Kalahari sand vegetation: effects of frost, prior damage, and tree neighbourhoods. Plant Ecology 108, 77-86.
| Crossref | Google Scholar |
Holdo RM, Mack MC, Arnold SG (2012) Tree canopies explain fire effects on soil nitrogen, phosphorus and carbon in a savanna ecosystem. Journal of Vegetation Science 23, 352-360.
| Crossref | Google Scholar |
Hurst DF, Griffith DWT, Cook GD (1994) Trace gas emissions from biomass burning in tropical Australian savannas. Journal of Geophysical Research: Atmospheres 99(D8), 16441-16456.
| Crossref | Google Scholar |
IPCC (Intergovernmental Panel on Climate Change) (2000) Good practice guidance and uncertainty management in national greenhouse gas inventories. Available at https://www.ipcc.ch/publication/good-practice-guidance-and-uncertainty-management-in-national-greenhouse-gas-inventories/
ISFMI (International Savanna Fire Management Initiative) (2015) ‘The global potential of Indigenous Fire Management.’ (Institute of Advanced Studies, United Nations University: Tokyo) Available at http://i.unu.edu/media/tfm.unu.edu/news/2151/Final-Report-Findings-Regional-Feasibility-Assessments-ISFMI.pdf
Lawton RM (1964) The ecology of the Marquesia Acuminata (Gilg) R.E.Fr. evergreen forest and the related chipya vegetation types of north-eastern Rhodesia. Journal of Ecology 52, 467-479.
| Crossref | Google Scholar |
Lawton RM (1978) A study of the dynamic ecology of Zambian vegetation. Journal of Ecology 66, 175-198.
| Crossref | Google Scholar |
Lipsett-Moore GJ, Wolff NH, Game ET (2018) Emissions mitigation opportunities for savanna countries from early dry season fire management. Nature Communications 9, 2247.
| Crossref | Google Scholar | PubMed |
Maier SW, Russell-Smith J (2012) Measuring and monitoring of contemporary fire regimes in Australia using satellite remote sensing. In ‘Flammable Australia: fire regimes, biodiversity and ecosystems in a changing world’. (Eds RA Bradstock, AM Gill, RW Williams) pp. 79–95. (CSIRO Publishing: Melbourne, Vic., Australia)
McNaughton SJ (1985) Ecology of a grazing ecosystem: the Serengeti. Ecological Monographs 55, 259-294.
| Crossref | Google Scholar |
Meyer CP, Cook GD, Reisen F, Smith TEL, Tattaris M, Russell‐Smith J, Maier SW, Yates CP, Wooster MJ (2012) Direct measurements of the seasonality of emission factors from savanna fires in northern Australia. Journal of Geophysical Research: Atmospheres 117, D20305.
| Crossref | Google Scholar |
Minas S (2022) Market making for the planet: the Paris Agreement Article 6 decisions and transnational carbon markets. Transnational Legal Theory 13, 287-320.
| Crossref | Google Scholar |
Mosugelo DK, Moe SR, Ringrose S, Nellemann C (2002) Vegetation changes during a 36-year period in northern Chobe National Park, Botswana. African Journal of Ecology 40, 232-240.
| Crossref | Google Scholar |
Moura LC, Scariot AO, Schmidt IB, Beatty R, Russell-Smith J (2019) The legacy of colonial fire management policies on traditional livelihoods and ecological sustainability in savannas: impacts, consequences, new directions. Journal of Environmental Management 232, 600-606.
| Crossref | Google Scholar | PubMed |
Murphy BP, Whitehead PJ, Evans J, Yates CP, Edwards AC, MacDermott HJ, Lynch DC, Russell-Smith J (2023) Using a demographic model to project the long-term effects of fire management on tree biomass in Australian savannas. Ecological Monographs 93, e1564.
| Crossref | Google Scholar |
Myhre G, Shindell DT, Breon F-M, Collins WJ, Fluglestvedt J, Huang J, Koch D, Lamarque J-, Lee D, Mendoza B, Nakajima, Robock A, Stephens G, Takemura T, Zhang H (2014) Anthropogenic and Natural Radiative Forcing. In ‘Climate Change 2013 – The Physical Science Basis. Vol. 6’. (Ed D Jacob, AR Ravishankara, K Shine) pp. 659–740. (Cambridge University Press: Cambridge, UK)
Ngoma J, Moors E, Kruijt B, Speer JH, Vinya R, Chidumayo EN, Leemans R (2018) Data for developing allometric models and evaluating carbon stocks of the Zambezi teak forests in Zambia. Data in Brief 17, 1361-1373.
| Crossref | Google Scholar | PubMed |
O’Connor TG, Puttick JR, Hoffmann MT (2014) Bush encroachment in southern Africa: changes and causes. African Journal of Range & Forage Science 31, 67-88.
| Google Scholar |
Oliveira SLJ, Campagnolo ML, Price OF, Edwards AC, Russell-Smith J, Pereira JMC (2015) Ecological implications of fine-scale fire patchiness and severity in tropical savannas of northern Australia. Fire Ecology 11, 10-31.
| Crossref | Google Scholar |
Parr CL, Lehmann CER, Bond WJ, Hoffmann WA, Andersen AN (2014) Tropical grassy biomes: misunderstood, neglected, and under threat. Trends in Ecology & Evolution 29, 205-213.
| Crossref | Google Scholar | PubMed |
Paul KI, Roxburgh SH (2024) A national accounting framework for fire and carbon dynamics in Australian savannas. International Journal of Wildland Fire 33(4), WF23104.
| Crossref | Google Scholar |
Pellegrini AFA, Ahlström A, Hobbie SE, Reich PB, Nieradzik LP, Staver AC, Scharenbroch BC, Jumpponen A, Anderegg WRL, Randerson JT, Jackson RB (2018) Fire frequency drives decadal changes in soil carbon and nitrogen and ecosystem productivity. Nature 553, 194-198.
| Crossref | Google Scholar | PubMed |
Pellegrini AFA, Hobbie SE, Reich PB, Jumpponen A, Brookshire ENJ, Caprio AC, Coetsee C, Jackson RB (2020) Repeated fire shifts carbon and nitrogen cycling by changing plant inputs and soil decomposition across ecosystems. Ecological Monographs 90, e01409.
| Crossref | Google Scholar |
Perry JJ, Cook GD, Graham E, Meyer CP, Murphy HT, VanDerWal J (2020) Regional seasonality of fire size and fire weather conditions across Australia’s northern savanna. International Journal of Wildland Fire 29, 1-10.
| Crossref | Google Scholar |
Ramo R, Roteta E, Bistinas I, van Wees D, Bastarrika A, Chuvieco E, van der Werf GR (2021) African burned area and fire carbon emissions are strongly impacted by small fires undetected by coarse resolution satellite data. Proceedings of the National Academy of Sciences 118(9), e2011160118.
| Crossref | Google Scholar | PubMed |
Ribeiro NS, Shugart HH, Washington-Allen R (2008) The effects of fire and elephants on species composition and structure of the Niassa Reserve, northern Mozambique. Forest Ecology and Management 255, 1626-1636.
| Crossref | Google Scholar |
Ribeiro NS, Matos CN, Moura IR, Washington-Allen RA, Ribeiro AI (2013) Monitoring vegetation dynamics and carbon stock density in miombo woodlands. Carbon Balance and Management 8, 11.
| Crossref | Google Scholar | PubMed |
Ribeiro NS, Syampungani S, Matakala NM, Nangoma D, Ribeiro‐Barros AS (2015) Miombo woodlands research towards the sustainable use of ecosystem services in southern Africa. In ‘Biodiversity in ecosystems: living structure and function’. (Eds J Blanco, Y‐H Lo, S Roy) pp. 475–491. (IntechOpen) 10.5772/59288
Ribeiro NS, Silva de Miranda PL, Timberlake J (2020b) Biogeography and ecology of Miombo Woodlands. In ‘Miombo Woodlands in a changing environment: securing the resilience and sustainability of people and woodlands’. (Eds NS Ribeiro, Y Katerere, PW Chirwa, IM Grundy) pp. 9–54. (Springer Nature: Switzerland, AG)
Ribeiro NS, Armstrong A, Fischer R, Kim Y-S, Shugart HH, Ribeiro-Barros AI, Chauque A, Tear T, Washington-Allen R, Bandeira RR (2021) Prediction of forest parameters and carbon accounting under different fire regimes in Miombo woodlands, Niassa Special Reserve, Northern Mozambique. Forest Policy & Economics 133, 102625.
| Crossref | Google Scholar |
Roteta E, Bastarrika A, Padilla M, Storm T, Chuvieco E (2019) Development of a Sentinel-2 burned area algorithm: generation of a small fire database for sub-Saharan Africa. Remote Sensing of Environment 222, 1-17.
| Crossref | Google Scholar |
Russell-Smith J, Edwards AC (2006) Seasonality and fire severity in savanna landscapes of monsoonal northern Australia. International Journal of Wildland Fire 15, 541-550.
| Crossref | Google Scholar |
Russell-Smith J, Cook GD, Cooke PM, Edwards AC, Lendrum M, Meyer CP, Whitehead PJ (2013a) Managing fire regimes in north Australian savannas: applying customary Aboriginal approaches to contemporary global problems. Frontiers in Ecology and the Environment 11, e55-e63.
| Crossref | Google Scholar |
Russell-Smith J, Yates C, Edwards A, Allan GE, Cook GD, Cooke P, Craig R, Heath B, Smith R (2003) Contemporary fire regimes of northern Australia, 1997–2001: change since Aboriginal occupancy, challenges for sustainable management. International Journal of Wildland Fire 12, 283-297.
| Crossref | Google Scholar |
Russell-Smith J, Murphy BP, Meyer CP, Cook GD, Maier S, Edwards AC, Schatz J, Brocklehurst P (2009) Improving estimates of savanna burning emissions for greenhouse accounting in northern Australia: limitations, challenges, applications. International Journal of Wildland Fire 18, 1-18.
| Crossref | Google Scholar |
Russell-Smith J, Monagle CM, Jacobsohn M, Beatty RL, Bilbao B, Vessuri H, Sánchez I, Millán A (2013b) Can savanna burning projects deliver measurable greenhouse emissions reductions, and sustainable livelihood opportunities for indigenous and local communities, in fire-prone settings? Climatic Change 140, 47-61.
| Crossref | Google Scholar |
Russell-Smith J, Yates CP, Evans J, Meyer CP, Edwards AC (2015) Application of a ‘lower rainfall’ savanna burning emissions abatement methodology. In ‘Carbon accounting and management in fire-prone Australian savannas’. (Eds BP Murphy, AC Edwards, CP Meyer, J Russell-Smith) pp. 219–234. (CSIRO Publishing: Melbourne, Vic., Australia)
Russell-Smith J, Yates C, Vernooij R, Eames T, van der Werf G, Ribeiro N, Edwards A, Beatty R, Lekoko O, Mafoko J, Monagle C, Johnston S (2021) Opportunities and challenges for savanna burning emissions abatement in southern Africa. Journal of Environmental Management 288, 112414.
| Crossref | Google Scholar | PubMed |
Ryan CM, Williams M (2011) How does fire intensity and frequency affect miombo woodland tree populations and biomass? Ecological Applications 21, 48-60.
| Crossref | Google Scholar | PubMed |
Scholes RJ (2003) Convex relationships in ecosystems containing mixtures of trees and grass. Environmental and Resource Economics 26, 559-574.
| Crossref | Google Scholar |
Scholes RJ, Archer SR (1997) Tree–grass interactions in savannas. Annual Review of Ecology and Systematics 28, 517-544.
| Crossref | Google Scholar |
Scholes RJ, Kendall J, Justice CO (1996) The quantity of biomass burned in southern Africa. Journal of Geophysical Research: Atmospheres 101(D19), 23667-23676.
| Crossref | Google Scholar |
Seiler W, Crutzen PJ (1980) Estimates of gross and net fluxes of carbon between the biosphere and the atmosphere from biomass burning. Climatic Change 2, 207-47.
| Crossref | Google Scholar |
Shea RW, Shea BW, Kauffman JB, Ward DE, Haskins CI, Scholes MC (1996) Fuel biomass and combustion factors associated with fires in savanna ecosystems of South Africa and Zambia. Journal of Geophysical Research: Atmospheres 101(D19), 23551-23568.
| Crossref | Google Scholar |
Shindell DT, Faluvegi G, Koch DM, Schmidt GA, Unger N, Bauer SE (2009) Improved attribution of climate forcing to emissions. Science 326, 716-718.
| Crossref | Google Scholar | PubMed |
Sirami C, Monadjem A (2012) Changes in bird communities in Swaziland savannas between 1998 and 2008 owing to shrub encroachment. Diversity and Distributions 18, 390-400.
| Crossref | Google Scholar |
Stromgaard P (1985) Biomass, growth, and burning of woodland in a shifting cultivation area of south central Africa. Forest Ecology & Management 12, 163-178.
| Crossref | Google Scholar |
Syampungani S, Tigabu M, Matakala N, Handavu F, Oden PC (2016) Coppicing ability of dry miombo woodland species harvested for traditional charcoal production in Zambia: a win–win strategy for sustaining rural livelihoods and recovering a woodland ecosystem. Journal of Forestry Research 28, 549-556.
| Crossref | Google Scholar |
Syampungani S, Chirwa PW, Geldenhuys CJ, Handavu F, Chishaleshale M, Rija AA, Mbanze AA, Ribeiro NS (2020) Managing miombo: ecological and silvicultural options for sustainable socio-economic benefits. In ‘Miombo Woodlands in a changing environment: securing the resilience and sustainability of people and woodlands’. (Eds Ribeiro NS, Katerere Y, Chirwa PW, Grundy IM) pp. 101–134. (Springer Nature: Switzerland, AG)
Tear TH, Wolff NH, Lipsett-Moore GJ, Ritchie ME, Ribeiro NS, Petracca LS, Lindsey PA, Hunter L, Loveridge AJ, Steinbruch F (2021) Savanna fire management can generate enough carbon revenue to help restore Africa’s rangelands and fill protected area funding gaps. One Earth 4, 1776-1791.
| Crossref | Google Scholar |
Trapnell CG (1959) Ecological results of woodland and burning experiments in northern Rhodisia. The Journal of Ecology 47, 129-168.
| Crossref | Google Scholar |
Trapnell CG, Friend MT, Chamberlain GT, Birch HF (1976) The effects of fire and termites on a Zambian woodland soil. Journal of Ecology 64, 577-588.
| Crossref | Google Scholar |
Trisos CH, Adelekan LO, Totin E, Ayanlade A, Efitre J, Gemeda A, Kalaba K, Lennard C, Masao C, Mgaya Y, Ngaruiya G, Olago D, Simpson NP, Zakieldeen S (2022) In Climate Change 2022: Impacts, Adaptation and Vulnerability. In ‘Contribution of Working Group II to the Sixth Assessment Report of the Intergovernmental Panel on Climate Change’. (Eds H-O Pörtner, DC Roberts, M Tignor, ES Poloczanska, K Mintenbeck, A Alegri’a, M Craig, S Langsdorf, S Löschke, V Möller, A Okem, B Rama) pp. 1285–1455. (Cambridge University Press: Cambridge, UK and New York, NY, USA) doi:10.1017/9781009325844.011
van der Werf GR, Randerson JT, Giglio L, van Leeuwen TT, Chen Y, Rogers BM, Mu M, van Marle MJE, Morton DC, Collatz GJ, Yokelson RJ, Kasibhatla PS (2017) Global fire emissions estimates during 1997–2016. Earth System Science Data 9, 697-720.
| Crossref | Google Scholar |
Veldman JW, Overbeck GE, Negreiros D, Mahy G, Le Stradic S, Fernandes GW, Durigan G, Buisson E, Putz FE, Bond WJ (2015) Where tree planting and forest expansion are bad for biodiversity and ecosystem services. Bioscience 65, 1011-1018.
| Crossref | Google Scholar |
Vernooij R, Giongo M, Borges MA, Costa MM, Barradas ACS, van der Werf GR (2021) Intraseasonal variability of greenhouse gas emission factors from biomass burning in the Brazilian Cerrado. Biogeosciences 18, 1375-1393.
| Crossref | Google Scholar |
Vernooij R, Winiger P, Wooster M, Strydom T, Poulain L, Dusek U, Grosvenor M, Roberts GJ, Schutgens N, van der Werf GR (2022) A quadcopter unmanned aerial system (UAS)-based methodology for measuring biomass burning emission factors. Atmospheric Measurement Techniques 15, 4271-4294.
| Crossref | Google Scholar |
Vernooij R, Eames T, Russell-Smith J, Yates C, Beatty R, Evans J, Edwards A, Ribeiro N, Wooster M, Strydom T, Giongo MV, Borges MA, Menezes Costa M, Barradas ACS, van Wees D, van der Werf GR (2023) Dynamic savanna burning emission factors based on satellite data using a machine learning approach. Earth System Dynamics 14, 1039-1064.
| Crossref | Google Scholar |
Walker SM, Desanker PV (2004) The impact of land use on soil carbon in miombo woodlands of Malawi. Forest Ecology and Management 203, 345-360.
| Crossref | Google Scholar |
Whitehead PJ, Russell-Smith J, Yates C (2014) Fire patterns in north Australian savannas: extending the reach of incentives for savanna fire emissions abatement. The Rangeland Journal 36, 371-388.
| Crossref | Google Scholar |
Williams M, Ryan CM, Rees RM, Sambane E, Fernando J, Grace J (2008) Carbon sequestration and biodiversity of re-growing miombo woodlands in Mozambique. Forest Ecology & Management 254, 145-155.
| Crossref | Google Scholar |
Williams RJ, Gill AM, Moore PHR (1998) Seasonal changes in fire behaviour in a tropical savanna in northern Australia. International Journal of Wildland Fire 8, 227-239.
| Crossref | Google Scholar |
Yates C, MacDermott H, Evans J, Murphy BP, Russell-Smith J (2020) Seasonal fine fuel and coarse woody debris dynamics in north Australian savannas. International Journal of Wildland Fire 29, 1109-1119.
| Crossref | Google Scholar |
Yates CP, Russell-Smith J, Murphy BP, Desailly M, Evans J, Legge S, Lewis F, Lynch D, Edwards AC (2015) Fuel accumulation, consumption and fire patchiness in the lower rainfall savanna region. In ‘Carbon accounting and savanna fire management’. (Eds BP Murphy, AC Edwards, CP Meyer, J Russell-Smith) pp. 115–132. (CSIRO Publishing: Melbourne, Vic., Australia)
Yibarbuk D, Whitehead PJ, Russell‐Smith J, Jackson D, Godjuwa C, Fisher A, Cooke P, Choquenot D, Bowman DMJS (2001) Fire ecology and Aboriginal land management in central Arnhem Land, northern Australia: a tradition of ecosystem management. Journal of Biogeography 28, 325-343.
| Crossref | Google Scholar |
Yokelson RJ, Burling IR, Urbanski SP, Atlas EL, Adachi K, Buseck PR, Wiedinmyer C, Akagi SK, Toohey DW, Wold CE (2011) Trace gas and particle emissions from open biomass burning in Mexico. Atmospheric Chemistry and Physics 11, 6787-808.
| Crossref | Google Scholar |
Yokelson RJ, Burling IR, Gilman JB, Warneke C, Stockwell CE, de Gouw J, Akagi SK, Urbanski SP, Veres P, Roberts JM, Kuster WC, Reardon J, Griffith DWT, Johnson TJ, Hosseini S, Miller JW, Cocker III DR, Jung H, Weise DR (2013) Coupling field and laboratory measurements to estimate the emission factors of identified and unidentified trace gases for prescribed fires. Atmospheric Chemistry and Physics 13, 89-116.
| Crossref | Google Scholar |
Zhou Y, Singh J, Butnor JR, Coetsee C, Boucher PB, Case MF, Hockridge EG, Davies AB, Staver AC (2022) Limited increases in savanna carbon stocks over decades of fire suppression. Nature 603(7901), 445-449.
| Crossref | Google Scholar |
Zulu LC, Kamoto JFM, Djenontin INS, Mbanze AA, Kambanje, Katerere Y (2020) Governance and Institutional arrangements for sustainable management of Miombo Woodlands. In ‘Miombo Woodlands in a changing environment: securing the resilience and sustainability of people and woodlands’. (Eds Ribeiro NS, Katerere Y, Chirwa PW, Grundy IM) pp. 138–189. (Springer Nature: Switzerland, AG)