Impact of fire return interval on pyrogenic carbon stocks in a tropical savanna, North Queensland, Australia
Jordahna Haig




A
B
C
Abstract
Indigenous fire management in northern Australian savannas (beginning at least 11,000 years ago) involved frequent, small, cool, early dry season fires. This fire regime changed after European arrival in the late 1700s to unmanaged fires that burn larger areas, late in the dry season, detrimental to carbon stocks and biodiversity.
Test the hypothesis that significant sequestration of pyrogenic carbon in soil accompanies the reimposition of an Indigenous fire regime.
Savanna soils under the same vegetation, but with the number of fires varying from 0 to 13 (irrespective of the season) between 2000 and 2022 were sampled. Organic and pyrogenic carbon stocks as well as carbon isotope composition of the 0–5 cm soil layer were determined along sample transects with varying fire return intervals.
An average increase of 0.25 MgC ha−1 was observed in soil pyrogenic carbon stocks in transects with ≥5 fires, compared to transects with 0–4 fires, with a small increase in soil organic carbon stocks that was not significant.
A return to more frequent fires early in the dry season has the potential to sequester significant pyrogenic carbon in northern Australian savanna soils on decadal timescales.
Keywords: biomass burning, carbon isotope, carbon sequestration, hydrogen pyrolysis, Indigenous fire management, northern Australia, pyrogenic carbon, tropical savanna.
Introduction
Pyrogenic carbon (PyC; char, black carbon) is naturally produced by the incomplete combustion of biomass during biomass burning (Bird et al. 2015). Once formed, it is largely resistant to mineralisation and will persist for centuries to millennia (Bird et al. 2015). The resistance of PyC to degradation and mineralisation has led to the suggestion that net carbon sequestration can be achieved by manufacturing PyC (biochar) and sequestering the carbon thus produced in agricultural soils, with attendant benefits to soil health and crop productivity (Woolf et al. 2010; Lehmann et al. 2021).
At the global level, Jones et al. (2019) estimated that 196–340 Tg of carbon was converted into PyC by wildfires annually over the 1996–2016 period, while Bowring et al. (2022) estimate 337 Tg of carbon converted annually to PyC. Both studies conclude that most PyC production from wildfires (49–73%) occurs in tropical mixed tree–grass savannas that are adapted to fire and burn more frequently with annual to sub-decadal return intervals, even though fuel loads in these biomes are relatively low compared to tropical or temperate forests. Tropical savannas therefore produce and sequester a quantity of PyC that is significant in the context of total terrestrial biospheric stocks and fluxes.
Savannas cover ~2 million km2 of northern Australia, around one-quarter of the total Australian land area. Savanna fires have been estimated to be responsible for 65% of carbon emitted from biomass burning in Australia (Murphy et al. 2019). The timing and frequency of fires across northern Australian savannas is highly variable. This variability is partly linked to the three major types of land tenure – pastoral lands, conservation estates and Indigenous lands – but also dependent on rainfall amount and seasonality (Perry et al. 2019; Edwards et al. 2021). Fires are ignited naturally (via lightning strike) or human induced, either by an accident, or design, or through regular bushfire abatement practices such as cultural burning practices. The average fire return interval, collated over the last 22 years by the Northern Australian Fire Information (NAFI) database (Jacklyn et al. 2015) over the entire northern savanna region, return interval is approximately biennial (Edwards et al. 2021) but with a high degree of spatial variability. In some wetter, more coastal regions, fires are an annual phenomenon often concentrated in the late dry season (after August). A substantial proportion of the drier inland area and particularly large (~100,000 ha) pastoral leases have experienced a fire return interval of 5 years or greater. Large regions have not burned in the 22-year period. When fires do occurit is mostly late in the dry season (Edwards et al. 2021).
As a result of the large area of Australian savannas and their impact on the national carbon budget, the Australian Government developed a ‘savanna burning methodology’ in 2018. This enables payment for emissions reductions generated by implementing improved fire management practices in northern Australia. The methodology recognises the traditional burning methods of Aboriginal peoples by shifting the timing of burning to earlier in the dry season, resulting in smaller and lower intensity fires. This results in avoided emissions associated with reductions in the size and number of high intensity, late dry season fires, and attendant increases in the stock of dead organic matter in the landscape. The approach was tested before implementation through initiatives such as the West Arnhem Land Fire Abatement (WALFA) project (2006–present). THis project implemented traditional burning over an area of 28,000 km² in the Northern Territory and monitored the impact of changing the timing and frequency of burning on carbon emissions in an Australian tropical savanna area (e.g. Russell-Smith et al. 2013).
While the impact of changes in fire return interval on Australian above- and belowground carbon stocks has received some attention in the recent years (Richards et al. 2011; Hunt 2014), pyrogenic carbon stocks have received only a little attention, and pyrogenic carbon sequestration was not included in the savanna burning methodology for calculating emissions offsets. There have been attempts to map the spatial distribution of pyrogenic carbon stocks both nationally (as resistant organic carbon, ROC; Viscarra Rossel et al. 2019) and globally (Reisser et al. 2016), but the impact of changes in fire regime on pyrogenic carbon stocks has received less attention. This study assesses the impact of fire return interval on both total organic carbon (TOC) and PyC stocks across an area of similar climate, covered by similar open grassy savanna on similar soil type of similarly low relief.
Methods
Study area
The area of this study in tropical north Queensland lies within the broad transcontinental arc of Australian savanna between 600 and 1000 mm annual rainfall and intense seasonality. This is within the region proposed for implementation of a savanna burning methodology for the purposes of carbon sequestration (Whitehead et al. 2014; Fig. 1), where landowners are eligible for carbon credits under the Australian Carbon Credit Unit (ACCU) Scheme, using the Savanna Fire Management – Sequestration and Emissions Avoidance Method (Department of Climate Change Energy the Environment and Water 2018a) or Savanna Fire Management – Emissions Avoidance Method (Department of Climate Change Energy the Environment and Water 2018b). Eligible land areas under both methods fall within either high (>1000 mm p.a.) and/or low (600–1000 mm p.a.) rainfall zones. See examples by Cook et al. (2016, 2020) for mathematical determination of these zones.
Map of Undara and Forty Mile Scrub National Parks (grey boundaries) showing the locations of the seven transects (blue lines) in relation to the number of years burned between 2000 and 2022 (red shading) as identified by North Australia and Rangelands Fire Information (NAFI) (2023). Transects are labelled with the number of years burned. The inset map gives the location of this study area in relation to the low rainfall zone boundary (orange region) for determining eligibility for participation in the Australian Government Carbon Credits (Carbon Farming Initiative) Act 2011.
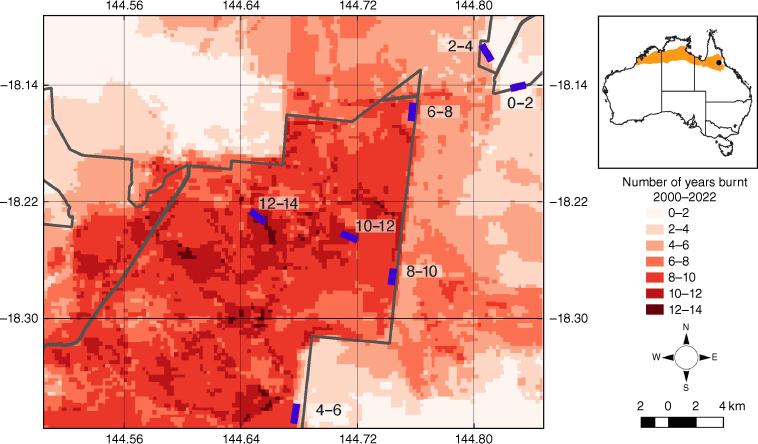
Sampling was conducted along transects with a range of fire return intervals in two small national parks in Einasleigh Uplands bioregion of North Queensland (Fig. 1). Undara Volcanic National Park was established from a former pastoral property in 1992. The park is located on a dominantly low relief landscape covered by relatively shallow stoney krasnozem soils (Grundy and Bryde 1989) developed on ~190,000-year-old Undara Basalt, part of the Late Cenozoic McBride volcanic province (Griffin and McDougall 1975), ~800 m above sea level. Climate is strongly seasonal with a mean annual temperature of 23.6°C (mean annual minimum/maximum temperatures 16.1/31.1°C) and mean annual rainfall of 795 mm, with 80% of this falling in the summer monsoon season from December to March (Bureau of Meteorology site number 030036, Mt Surprise township, 40–60 km west of Undara).
The basalt plains are covered by open, grassy Eucalypt woodland, traditionally the lands of the Ewamin Aboriginal peoples (QPWS 2023a). In an effort to combat invasive grass species (Vogler 2017) and return the park to something resembling an Indigenous fire regime with a mosaic of habitats for wildlife, the park area was aimed to be burned in rotation approximately every 2 years since at least 2002 (Abom et al. 2016). In practice, the Northern Australian Fire Information (NAFI) mapping service (Jacklyn et al. 2015) indicates that discrete areas in the Park have burned 5–13 times between 2000 and 2022. These high fire return intervals contrast strongly with the surrounding pastoral properties on similar soils and with similar vegetation, which have most commonly experienced 0–4 fires in the 2000–2022 period, equating to a return interval of ≥5 years (Fig. 1). The NAFI data is generated weekly by comparing MODIS satellite images 1–2 weeks apart and identifying areas which have been burned in the intervening period.
Forty Mile Scrub National Park is located 10–20 km northeast of Undara with similar climate and soils. While the park was established in 1970 to protect semi-evergreen vine thicket (QPWS 2023b), the park also protects areas of the same open grassy Eucalypt woodland that dominates Undara, and these areas were selected for sampling in this study (Fig. 1). Unlike Undara, this park has not had the extended history of intentional fire management. Like the surrounding pastoral properties, most areas have burned 0–4 times (Fig. 1), with unmanaged large fires having burned the open woodland and into the evergreen vine thicket on occasion in the 2000–2022 period.
Field
Seven soil sampling transects in each of the two parks, all within 40 km of each other, on similar basalt substrate and covered by open grassy savanna were chosen to provide a range of fire return intervals from ~2 to >11 years, as indicated by the NAFI fire database from 2000 to 2022 (Jacklyn et al. 2015). Woody cover (trees and shrubs) for areas within a ~500 m polygon surrounding each transect were calculated using the persistent green data layer extracted from the VegMachine landcover dataset (Beutel et al. 2019) and averaged over the last 20 years. This area has been actively managed with respect to the fire frequency by the Queensland Parks and Wildlife Service who conduct regular burns based on their fire management strategy. Under this strategy the Parks are burned in a mosaic pattern leaving some areas unburned, varying year to year.
Soil sampling followed the modified sampling protocol for mixed tree–grass ecosystems described by Wynn et al. (2006), with each sample collected by banging a soil corer (diameter of 50 mm) into the soil (after removal of litter) to a depth of 5 cm. The volume of soil in the corer was quantitatively recovered by excavating the corer and the sample placed in a ziploc bag. Along each transect, five sampling locations were selected, with minimum separation of 100 m from each other. At each of these locations two soil cores were taken, one at half-crown distance from the base of local trees (0–5 cm depth, designated 0–5T – Tree) and one core from midway between local tree stems in the grassy understorey (designated 0–5G – Grass). This approach ensures that local heterogeneity in carbon stocks and carbon isotope composition associated with modern tree–grass distribution can be captured by weighting the total stocks and isotope composition for a transect by proportion of tree canopy cover (Wynn et al. 2006).
Analytical
Weighed samples were dried at 70°C, sieved at 2 mm and re-weighed to enable calculation of dry bulk density of the <2 mm fraction. The sieved sample was then ball milled to a powder for all subsequent analyses and re-dried overnight at 70°C.
The stable polycyclic aromatic carbon (SPAC, hereafter called ‘PyC’) component was separated from labile soil carbon by hydrogen pyrolysis. This is an established method (Wurster et al. 2013) that yields the abundance and stable isotope composition of pyrogenic carbon that is resistant to degradation on centennial timescales (with >7 condensed aromatic rings), rather than all components of the pyrogenic carbon continuum, some of which are also labile (Bird et al. 2015). 250 mg of each crushed sample was loaded at 10% by weight with a molybdenum catalyst using an aqueous methanol solution of ammonium dioxydithiomolybdate, sonicated and dried at 70°C overnight. The sample/catalyst mixture was loaded into the reactor of the instrument, pressurised with H2 to 150 bar with a purge gas flow of 5 L min−1, heated at 300°C min−1 to 250°C, then by 8°C min−1 to 550°C, with the final temperature held for 5 min.
While hydrogen pyrolysis is an established methodology, there are only a few laboratories in the world with the capacity to make these measurements in part due to the special safety precautions that need to be taken when working with H2 at high temperature and pressure. As such, we also explored the use of diffuse reflectance Fourier-transform mid-infrared spectroscopy (mid-DRIFTS) as a relatively inexpensive, rapid and safe alternative to simultaneously estimate the total organic carbon (TOC) and PyC content of the soil samples (Baldock et al. 2013; Sanderman et al. 2021a, 2021b). Briefly, finely milled subsamples of each soil were scanned on a Bruker Vertex 70 FTIR (Bruker Optics, Billerica, MA, USA) with a wide-range beamsplitter/detector combination and a Pike AutoDiff (Pike Technologies, Fitchburg, WI, USA) diffuse reflectance accessory. After standard normal variate pre-processing on the 4000–628 cm−1 spectral range and normalisation of the analytical data using natural log (1+ number) transformation, partial least squares regression (PLSR, Geladi and Kowalski 1986) models were built to predict TOC% and PyC% using 20-fold cross-validation within the Unscrambler v. 10 software (Aspen Tech, Bedford, MA, USA).
The carbon abundance and carbon isotope composition of total organic carbon (TOC, δ13CTOC) and PyC (δ13CPyC) were measured by elemental analysis isotope ratio mass spectrometry using a ThermoScientific Flash EA with Smart EA option coupled via a Conflo IV to a Delta VPlus at James Cook University’s Advanced Analytical Centre. Carbon isotope measurements are reported as per mil (‰) deviations from the Vienna Pee Dee Belemnite (VPDB) reference standard scale for δ13C. International standard USGS-40 and two internal laboratory reference materials (Taipan, Chitin) were analysed within each analytical sequence for three-point calibrations of isotope delta-scale anchored by VPDB. The internal standards were calibrated using USGS40 and USGS41 international reference materials and results have an uncertainty of ±0.2‰ or better. PyC abundances have an average standard error of 2.2% of the value, which we corrected for possible in situ production of stable polycyclic aromatic carbon during the hydrogen pyrolysis reaction (Wurster et al. 2012; Meredith et al. 2017). TOC and PyC abundance measurements were converted into stocks to 5 cm depth using the dry bulk density of each sample. PyC stocks were subtracted from the TOC stocks to yield an estimate of organic carbon (OC) stocks and mass balance based on δ13CTOC and δ13CPyC values used to calculate δ13COC values.
Statistical
The data was described graphically using box plots. Prior to statistical analysis, the distribution of the measurements was tested for normality using a Shapiro–Wilk test and/or Q–Q plots. Where appropriate, comparisons were made between tree–grass pairs using a paired sample t-test. Given the unequal variances and sample sizes of the <5 and ≥5 populations, a Welch Two sample t-test (or Wilcoxon Rank Sum test in the case of non-normality) was used to compare the two groups. Lastly, a Kruskal–Wallis Test and Cuzick Trend Test were used to compare the transects. The value of the test statistic and P-value are reported in the text. All statistics are reported at the 0.05 significance level. We made efforts to avoid pseudo-replication in our sampling methodology by collecting replicates within each transect which were sufficiently separated spatially (min 100 m), and ensuring that the transects were separated by a minimum distance of 3 km. The transects themselves were not replicated as the transects were later grouped into larger temporal ranges.
Results
We discuss the data in two groups: (i) transects burned less than 5 times (transects 0–2 and 2–4 of Fig. 1, return interval >4.4 years; 18 samples), and (ii) transects that have burned 5 or more times in the 2000–2022 period (return interval <4.4 years; 48 samples). The results separated by individual transect are provided in Supplementary Tables S1, S2 and Fig. S1.
The only large-scale ecological difference between the two groups apart from fire return interval is that the <5 fire transects currently have a higher tree canopy cover (23–29% persistent green) than the transects that have burned ≥5 times (15.2–22%; Fig. 2). This difference may have developed as a result of the lower fire return interval, and/or through proximity to the vine thicket seed bank of Forty Mile Scrub itself, 2–3 km north of the <5 fire transects.
Box plots showing the percent woody cover (persistent green) within Undara and Forty Mile Scrub National Parks grouped by years burned (less than or greater than 5 fire years in the last 22 years) as identified from North Australia and Rangelands Fire Information (NAFI) (2023). Landsat seasonal persistent green estimates obtained from VegMachine (Beutel et al. 2019) have been averaged from the period of January 2000 to January 2021. The box encloses 50% of the central data with the mean (black diamonds), median (black lines), individual data points (circles), and maximum and minimum values (vertical black lines) also indicated.
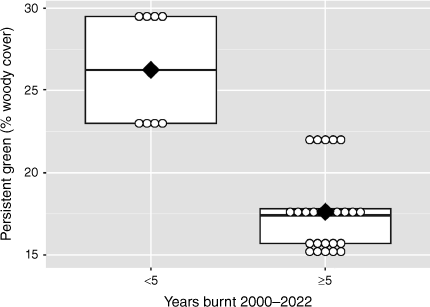
Soil dry bulk density ranged from 0.55 to 1.55 g cm−3 over the 0–5 cm depth layer (Fig. 3), with the <5 fire transects having a significantly (t = −2.96, P-value <0.05) lower bulk density (µ = 0.95 g cm−3) than the ≥5 fire transects (µ = 1.18 g cm−3). There was no significant average difference (t = −1.10, P-value >0.05) between tree (T) and grass (G) pairs with respect to dry bulk density.
Box plots comparing sites of fewer and greater than or equal to 5 fire years in a 22 year period within Undara and Forty Mile Scrub National Parks with respect to (a) dry bulk density, (b) pyrogenic/organic carbon % and differences between their respective tree and grass pairs (c, d). The box encloses 50% of the central data with the mean (black diamonds), median (black lines), individual data points (circles), and maximum and minimum values (vertical black lines) also indicated.
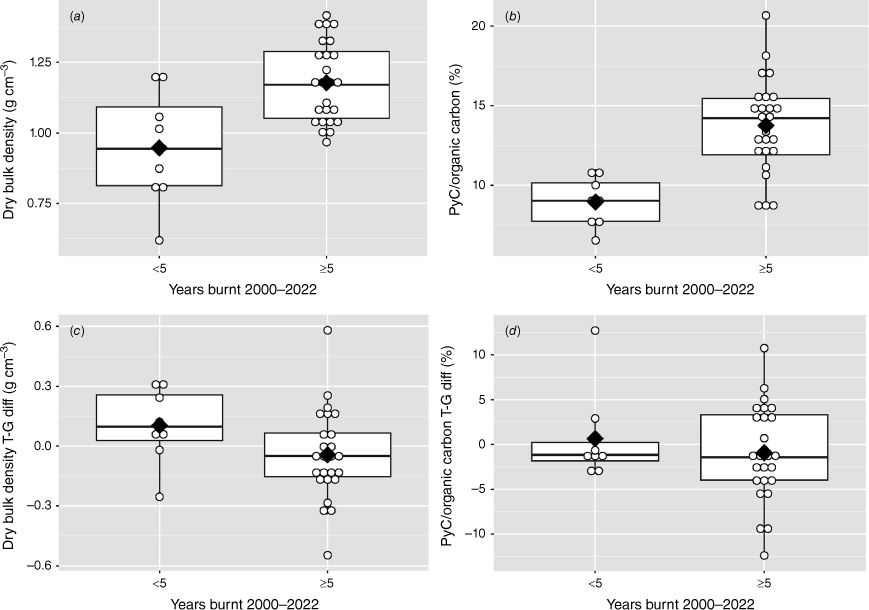
Organic carbon stocks ranged from 10.3 to 27.2 MgC ha−1 (Fig. 4), with no significant (t = −1.68, P-value >0.05) difference between <5 (µ = 15.22 MgC ha−1) and ≥5 fire (µ = 17.46 MgC ha−1), while there are differences between tree (T) and grass (G) pairs (t = −2.89, P-value <0.05) there is no significant difference between tree (T) and grass (G) samples between groups (t = −0.09, P-value >0.05). Pyrogenic carbon stocks ranged from 0.61 to 3.39 MgC ha−1, with the mean stocks for the <5 fire transects (1.39 MgC ha−1) being significantly lower (t = −5.66, P-value <0.05) than for the ≥5 fire transects (2.39 MgC ha−1), and no difference in stocks between the T and G pairs in any group (t = −0.10, P-value >0.05; Fig. 4). The similarity in OC stocks and difference in PyC stocks drove a significant increase in PyC/OC ratio (t = −5.95, P-value <0.05). This ratio ranged from 4.1 to 23.6, with a mean PyC/OC of 8.94 for the <5 fire transects, and 13.74 for the ≥5 fire transects (Fig. 3). There was no significant difference between PyC/OC for T versus G pairs in either group (t = 0.59, P-value >0.05).
Box plots comparing sites of fewer and greater than or equal to 5 fire years in a 22-year period within Undara and Forty Mile Scrub National Parks with respect to (a) Organic and (b) Pyrogenic carbon stocks, and differences between their respective tree and grass pairs (c, d). The box encloses 50% of the central data with the mean (black diamonds), median (black lines), individual data points (circles), and maximum and minimum values (vertical black lines) also indicated.
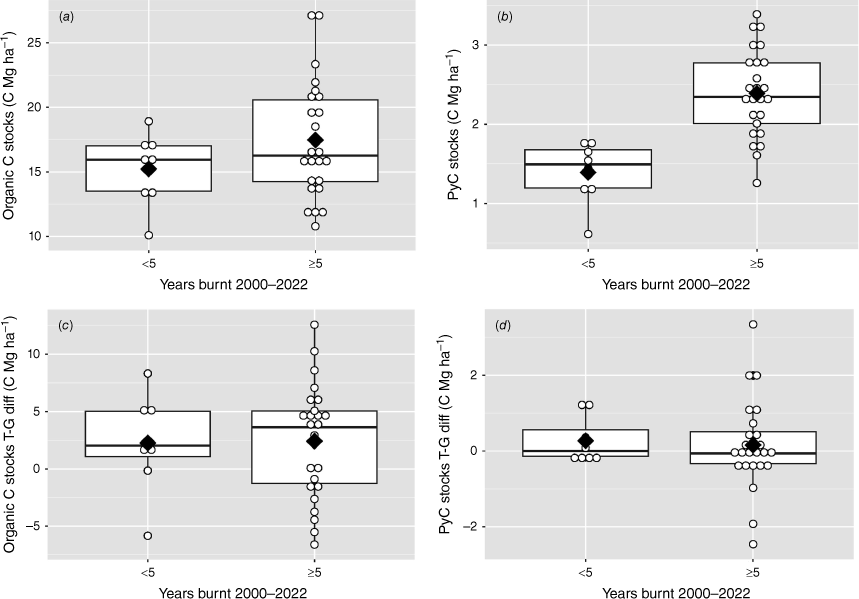
δ13COC values ranged from −26‰ to −16.6‰, a range typical of savanna environments (Fig. 5). As a result of the higher canopy cover, the average δ13COC (−24.1‰) in the <5 fire transects was significantly lower (t = −14.27, P-value <0.05) than for the ≥5 fire transects −18.3‰) with the T samples in the ≥5 fire transects having a lower δ13COC (by an average 1.1‰) than the G samples, with no difference between T and G samples in the <5 fire transects (t = 0.72, P-value >0.05; Fig. 5). δ13CPyC values ranged from −15‰ to −27.1‰, with average values 1–3‰ higher than δ13COC values and the average value for <5 fire transects (−21.1‰) being significantly lower (W = 0, P-value <0.05) than the average value for the ≥5 fire transects (−16.3‰; Fig. 5). In both groups the average difference between the T and G δ13CPyC pairs was close to 0 (µT–G<5 = 0.65, µT–G>5 = −0.67; Fig. 5).
Box plots comparing sites of fewer and greater than or equal to 5 fire years in a 22-year period within Undara and Forty Mile Scrub National Parks with respect to (a) Organic and (b) Pyrogenic carbon δ13C value, and differences between their respective tree and grass pairs (c, d). The box encloses 50% of the central data with the mean (black diamonds), median (black lines), individual data points (circles), and maximum and minimum values (vertical black lines) also indicated.
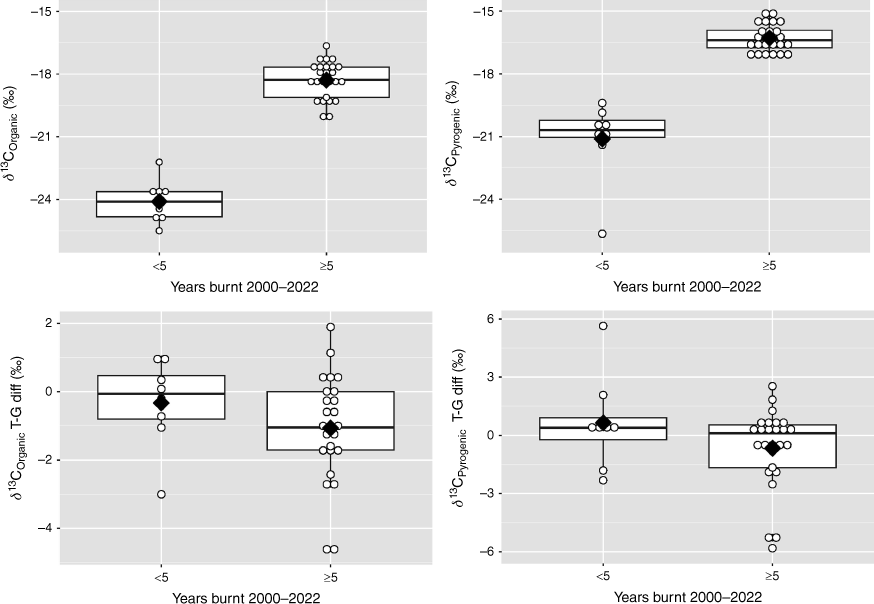
Cross validation results of PLSR models for TOC concentration were excellent with an R2 = 0.95, root mean squared error (RMSE) = 0.024, bias = 0.001 and Ratio of Performance to InterQuartile distance (RPIQ) = 4.10 (Fig. 6a). Results for PyC concentration were less certain with an R2 = 0.40, RMSE = 0.033, bias = −0.001 and RPIQ = 1.56 (Fig. 6b). Inspection of the PLSR model forms (i.e. variable importance) indicates a mix of similar and distinct features (Fig. 6c). In particular, the two models share positive features related to aromatic carbon (1650 cm−1) but only the TOC model includes aliphatic carbon (2925 and 2850 cm−1)
Discussion
Across both the <5 and ≥5 fire transects, OC stocks are on average higher in the -T (tree) than the -G (grass) samples (Fig. 4), consistent with previous research that has shown higher soil carbon stocks beneath trees in Australian tropical savannas (Wynn et al. 2006). In contrast, there is no difference in the PyC stocks associated with current tree cover, due to the long residence time of PyC in the soil (e.g. Lehmann et al. 2008, 2021; Bird et al. 2015), and hence PyC stocks are not closely related to current tree cover distribution.
The δ13COC values for all transects (Fig. 5) are within the range previously reported for Australian tropical savannas and -T samples have lower δ13COC values than the -G samples also consistent with previous research (Bird and Pousai 1997; Wynn et al. 2006). The lower δ13COC values for the <5 fire transects are consistent with the higher tree cover on those transects (Fig. 2). The δ13CPyC values tend to be 1–3‰ higher than the corresponding δ13COC values, suggesting preferential incorporation of C4-derived PyC into the soil. As with the PyC stocks, there is no significant difference in δ13CPyC values between the -T and -G samples due to the long residence time of PyC in the soil.
The results clearly indicate that PyC stocks are higher in all areas burned five or more times (5–13 times) in the 2000–2022 period, compared to unmanaged areas that burned between zero and four times (<5 times) in the same period. The difference in PyC stocks amounts to an average of ~0.25 MgC ha−1 more PyC at sites burned ≥5 times. The increase did not come at the expense of soil organic carbon stocks which average ~16–17 MgC ha−1 for the 0–5 cm layer (Fig. 4). It is not a result of the minor differences in bulk density between the <5 and ≥5 fire transects (Fig. 3) as the PyC/OC ratio, which is not influenced by soil bulk density, is also significantly higher in the more frequently burned transects. Given the recalcitrant nature of the pyrogenic carbon component measured by hydrogen pyrolysis, the pyrogenic carbon stock in the soil can be considered sequestered for centuries to millennia (Bird et al. 2015).
Saiz et al. (2015) quantified PyC production using the same analytical technique (hydrogen pyrolysis) for small experimental burns also on Undara Volcanic National Park. They found that each fire produced an average of 0.11 MgC ha−1 that remained on the ground, with some additional PyC exported in the very fine fraction (as ash and other particulates). Given likely post-fire losses of PyC through recombustion in subsequent fires (Saiz et al. 2014), via water transport and through wind erosion, particularly of fine grass-derived PyC (Saiz et al. 2018), the measured production of PyC at Undara in the experimental burns is broadly consistent with the extent of measured additional sequestration of ~0.25 MgC ha−1 in the ≥5 fire transects.
For at least the last ~11,000 years (Bird et al. 2024) but ceasing with the establishment of pastoral stations in the late 1800s, the tropical savannas of northern Australia were subjected to Indigenous fire management involving mosaic burning with a relatively high fire return interval (e.g. Russell-Smith et al. 2013). Under European management, fire was not actively managed or was suppressed for much of the 20th century. The reimposition of an Indigenous fire regime over the last two decades at Undara Volcanic National Park has enabled an assessment of the impact of the re-introduction of Indigenous fire management on pyrogenic carbon stocks, through comparison with an adjacent area that has not seen the return of Indigenous fire management.
The <5 fire transects would have been burned at similar frequency to the ≥5 fire transects before European settlement (e.g. Russell-Smith et al. 2013) and other things being equal, the PyC stocks for both groups should have simply remained as they were, that is, similar to the stocks ≥5 fire transects now. Therefore, the lower PyC stocks in the 0–5 cm soil layer of <5 fire transects indicate that there has been a relative loss of PyC from the 0–5 cm soil layer over the last century for the <5 fire transects. The PyC component quantified in this study is resistant to degradation on centennial to millennial timescales (Bird et al. 2015), implying this loss has been the result of physical translocation out of the 0–5 cm layer. Some lower temperature formed PyC may have been lost via mineralisation, and some PyC may have been lost through deflation from the soil surface. But it is likely that the majority of the decrease has resulted from the movement of PyC below the 0–5 cm layer investigated in this study. This likely occurred by a combination of bioturbation and illuviation during rain events over the last century. Downward movement of PyC has been reported in experimental studies (Nguyen et al. 2009; Major et al. 2010) and must occur given the fact that significant PyC stocks are reported well below the soil surface (Koele et al. 2017; Hobley 2019). With a lower rate of ‘replenishment’ of PyC to the soil surface as a result of a low fire return interval, the PyC stocks in the 0–5 cm layer of the <5 fire transects have decreased as the PyC has been redistributed to depth over the last century. Reimposition of a higher fire return interval has ‘replenished’ the PyC stocks in 0–5 cm soil layer of the ≥5 fire transects over the last two decades.
The mid-DRIFTS analysis was included in this study because if soil or pyrogenic carbon sequestration due to increased fire return interval, were to be traded on the carbon market, low-cost methods for quantifying the increase in TOC and PyC are needed (Department of the Prime Minister and Cabinet 2021). In this regard, mid-DRIFTS is an allowable alternative to traditional analysis for TOC in the Australian Government’s soil carbon credit methodology (Department of Climate Change Energy the Environment and Water 2021). The excellent results for TOC are consistent with other studies (Baldock et al. 2013; Sanderman et al. 2021b). The models for PyC had greater uncertainty but importantly were unbiased, (Fig. 6) suggesting that with increased sample numbers detection of even small changes in PyC over time is possible. The PyC model performance was lower than in some other studies that focused on developing spectral-prediction models for PyC (Baldock et al. 2013; Cotrufo et al. 2016; Hobley et al. 2016), but the samples from Undara had a much smaller range in PyC concentrations than those other studies, suggesting that model performance could be significantly improved with a more diverse training set.
Noting that any fire, regardless of fire regime, will lead to some PyC delivery to the soil, our results suggest a managed return to ≥5 early dry season fires per 1–2 decades will yield sequestration of PyC in the 0–5 cm soil layer amounting to ~0.25 MgC ha−1. Therefore, sequestration of 1250–2500 MgC annually is potentially achievable for an average pastoral property of 100,000 ha. With the current spot generic Australian Carbon Credit Unit (ACCU) price sitting at ~AUD$32 (Clean Energy Regulator 2023), in fiscal terms this equates to almost AUD$1 million per decade (~AUD$40,000–80,000 per year) in potential revenue. While this is obviously an illustrative figure only, it does indicate that a return to a managed, early dry season, higher return interval fire regime can provide significant long-term carbon sequestration given the large area of northern Australian savannas. Given the recalcitrant nature of the sequestered carbon compared to OC, this may be attractive to the buyers of carbon credits. We also note that an increase of ~2 MgC ha−1 of OC occurred in the more frequently burned plots. Although this increase is not significant, it is consistent with the modelling of Richards et al. (2011) who found that a statistically significant increase in soil organic carbon stocks of 4 Mg ha−1 over a century accompanied a change in fire regime to cool burns with a 5-year return interval, equivalent to ~0.8 MgC over the period considered in this study.
It is likely that the PyC sequestration measured in this study is conservative as only the 0–5 cm layer was sampled, and PyC translocation to deeper soil layers is occurring. It is also possible that PyC stocks will continue to increase to an unknown extent if the higher return interval is maintained in the future. There is a suggestion that optimal PyC sequestration in the soils of the study area occurred for transects burned 5–8 times in the 2000–2022 period (H = 8.12, P-value <0.05; Fig. S1), equating to a return interval of 3–4 years. From a fire frequency of 5 in 22 years, there is a significant (z = −2.49, P-value <0.05) downward trend in PyC stocks with increasing fire frequency. At the highest return intervals, PyC sequestration may be limited by a combination of reduced biomass available to burn along with increased potential for recombustion of PyC by subsequent fires before incorporation into the soil. It should also be noted that PyC sequestration may be augmented or offset by changes in the quantity and composition of greenhouse gas releases, and changes in dead biomass, soil carbon and nutrient cycling accompanying a change to more frequent, cooler fires (Cook et al. 2016).
The potential for PyC sequestration in savanna soils across northern Australia will also vary as a function of at least (i) climate – through control of tree–-grass distribution and biomass, (ii) livestock management – through control of above ground biomass, and (iii) soil type and landscape position, through control of tree–grass distribution, total biomass and the rate of PyC erosion from the soil surface and/or protection through illuviation to deeper soil layers or lateral movement by water erosion.
There is clearly potential for significant, ongoing soil PyC sequestration on decadal timescales through the broader reintroduction of a fire regime similar to that imposed for millennia by Aboriginal peoples in northern Australian savannas. This sequestration is supplementary to the avoided emissions that are currently allowable for carbon credits through the Australian Government's savanna burning methodology (Edwards et al. 2021) and additional biodiversity co-benefits may also accrue over time (Evans and Russell-Smith 2019; Sangha et al. 2021; Greenwood et al. 2022). Management of fire as a tool for improving carbon sequestration, avoiding emissions and improving biodiversity outcomes has the advantage of being relatively easy to implement and to monitor remotely (Jacklyn et al. 2015). However, the benefits of more frequent, cooler fires must be weighed against the opportunity costs of forgoing other land use options, broader changes in ecosystem carbon stocks and greenhouse gas emissions (Cook et al. (2016), positive (Greenwood et al. 2022) and potentially negative biodiversity impacts (Abom et al. 2016) as well as the potential health impacts associated with increased smoke pollution (Jones et al. 2022).
Acknowledgements
We acknowledge the support of the staff and traditional owners of Undara Volcanic National Park for granting and facilitating access to the Park (approval WITK18707717). This research was funded by the Australian Research Council grant DP210100881.
Author contributions
J. H.: formal analysis, investigation, methodology, writing original draft, review, editing. J. S.: conceptualisation, formal analysis, data curation, investigation, methodology, writing original draft, review, editing. C. Z.: investigation, methodology, writing – draft review, editing. C. S.: investigation, methodology, writing – draft review, editing. M. I. B.: conceptualisation, formal analysis, data curation, investigation, methodology, writing original draft, review, editing.
References
Abom R, Parsons SA, Schwarzkopf L (2016) Complex mammal species responses to fire in a native tropical savannah invaded by non-native grader grass (Themeda quadrivalvis). Biological Invasions 18, 3319-3332.
| Crossref | Google Scholar |
Baldock JA, Hawke B, Sanderman J, Macdonald LM (2013) Predicting contents of carbon and its component fractions in Australian soils from diffuse reflectance mid-infrared spectra. Soil Research 51(8), 577-595.
| Crossref | Google Scholar |
Beutel TS, Trevithick R, Scarth P, Tindall D (2019) VegMachine net online land cover analysis for the Australian rangelands. The Rangeland Journal 41(4), 355-362.
| Crossref | Google Scholar |
Bird MI, Pousai P (1997) Variations of δ13C in the surface soil organic carbon pool. Global Biogeochemical Cycles 11(3), 313-322.
| Crossref | Google Scholar |
Bird MI, Wynn JG, Saiz G, Wurster CM, McBeath A (2015) The pyrogenic carbon cycle. Annual Review of Earth and Planetary Sciences 43, 273-298.
| Crossref | Google Scholar |
Bird MI, Brand M, Comley R, Fu X, Hadeen X, Jacobs Z, Rowe C, Wurster CM, Zwart C, Bradshaw CJ (2024) Late Pleistocene emergence of an anthropogenic fire regime in Australia’s tropical savannahs. Nature Geoscience 17, 233-240.
| Crossref | Google Scholar |
Bowring SP, Jones MW, Ciais P, Guenet B, Abiven S (2022) Pyrogenic carbon decomposition critical to resolving fire’s role in the Earth system. Nature Geoscience 15(2), 135-142.
| Crossref | Google Scholar |
Clean Energy Regulator (2023) Quarterly Carbon Market Report June Quarter 2023. Available at https://wwwcleanenergyregulatorgovau/Infohub/Markets/Pages/qcmr/june-quarter-2023/Australian-carbon-credit-units-(ACCUs)aspx [accessed 20 November 2023].
Cook GD, Meyer CP, Muepu M, Liedloff A (2016) Dead organic matter and the dynamics of carbon and greenhouse gas emissions in frequently burned savannas. International Journal of Wildland Fire 25, 1252-1263.
| Crossref | Google Scholar |
Cook GD, Liedloff AC, Meyer CP, Richards AE, Bray SG (2020) Standing dead trees contribute significantly to carbon budgets in Australian savannas. International Journal of Wildland Fire 29, 215-228.
| Crossref | Google Scholar |
Cotrufo MF, Boot C, Abiven S, Foster EJ, Haddix M, Reisser M, Wurster CM, Bird MI, Schmidt MW (2016) Quantification of pyrogenic carbon in the environment: an integration of analytical approaches. Organic Geochemistry 100, 42-50.
| Crossref | Google Scholar |
Department of Climate Change Energy the Environment and Water (2018a) ‘Savanna fire management - sequestration and emissions avoidance method.’ (Australian Government) Available at https://wwwdcceewgovau/climate-change/emissions-reduction/emissions-reduction-fund/methods/savanna-fire-management-sequestration-and-emissions-avoidance [24 April 2024]
Department of Climate Change Energy the Environment and Water (2018b) ‘Savanna fire management - emissions avoidance method.’ (Australian Government) Available at https://wwwdcceewgovau/climate-change/emissions-reduction/emissions-reduction-fund/methods/savanna-fire-management-emissions-avoidance [24 April 2024]
Department of Climate Change Energy the Environment and Water (2021) Carbon Credits (Carbon Farming Initiative—Estimation of Soil Organic Carbon Sequestration Using Measurement and Models) Methodology Determination. Available at https://wwwlegislationgovau/F2021L01696/latest/text [accessed 2 January 2024]
Department of the Prime Minister and Cabinet (2021) The Plan to Deliver Net Zero – The Australian Way. Available at https://wwwdcceewgovau/sites/default/files/documents/the-plan-to-deliver-net-zero-the-australian-way.pdf [accessed 2 January 2024]
Edwards A, Archer R, De Bruyn P, Evans J, Lewis B, Vigilante T, Whyte S, Russell-Smith J (2021) Transforming fire management in northern Australia through successful implementation of savanna burning emissions reductions projects. Journal of Environmental Management 290, 112568.
| Crossref | Google Scholar | PubMed |
Evans J, Russell-Smith J (2019) Delivering effective savanna fire management for defined biodiversity conservation outcomes: an Arnhem Land case study. International Journal of Wildland Fire 29(5), 386-400.
| Crossref | Google Scholar |
Geladi P, Kowalski BR (1986) Partial least-squares regression: a tutorial. Analytica Chimica Acta 185, 1-17.
| Crossref | Google Scholar |
Greenwood L, Bliege Bird R, Nimmo D (2022) Indigenous burning shapes the structure of visible and invisible fire mosaics. Landscape Ecology 37, 811-827.
| Crossref | Google Scholar |
Griffin TJ, McDougall I (1975) Geochronology of the Cainozoic McBride volcanic province northern Queensland. Journal of the Geological Society of Australia 22(4), 387-396.
| Crossref | Google Scholar |
Hobley E (2019) Vertical distribution of soil pyrogenic matter: a review. Pedosphere 29(2), 137-149.
| Crossref | Google Scholar |
Hobley EU, Brereton AG, Wilson B (2016) Soil charcoal prediction using attenuated total reflectance mid-infrared spectroscopy. Soil Research 55(1), 86-92.
| Crossref | Google Scholar |
Hunt LP (2014) Aboveground and belowground carbon dynamics in response to fire regimes in the grazed rangelands of northern Australia: initial results from field studies and modelling. The Rangeland Journal 36, 347-358.
| Crossref | Google Scholar |
Jacklyn P, Ansell S, Crosbie A, Holmes J, Joseph A, Legge S, Lewis B, Pickworth A, Vigilante T, Weigl J (2015) The operational role of satellite-based fire data in savanna burning methodologies. In ‘Carbon Accounting and Savanna Fire Management’. (Eds B Murphy, J Russell-Smith, A Edwards, M Meyer, CP Meyer) pp. 97–113. (CSIRO Publishing: Melbourne, Australia)
Jones MW, Santín C, van der Werf GR, Doerr SH (2019) Global fire emissions buffered by the production of pyrogenic carbon. Nature Geoscience 12(9), 742-747.
| Crossref | Google Scholar |
Jones PJ, Furlaud JM, Williamson GJ, Johnston FH, Bowman D (2022) Smoke pollution must be part of the savanna fire management equation: a case study from Darwin Australia. Ambio 51(11), 2214-2226.
| Crossref | Google Scholar | PubMed |
Koele N, Bird M, Haig J, Marimon-Junior BH, Marimon BS, Phillips OL, De Oliveira EA, Quesada CA, Feldpausch TR (2017) Amazon Basin forest pyrogenic carbon stocks: first estimate of deep storage. Geoderma 306, 237-243.
| Crossref | Google Scholar |
Lehmann J, Skjemstad J, Sohi S, Carter J, Barson M, Falloon P, Coleman K, Woodbury P, Krull E (2008) Australian climate–carbon cycle feedback reduced by soil black carbon. Nature Geoscience 1, 832-835.
| Crossref | Google Scholar |
Lehmann J, Cowie A, Masiello CA, Kammann C, Woolf D, Amonette JE, Cayuela ML, Camps-Arbestain M, Whitman T (2021) Biochar in climate change mitigation. Nature Geoscience 14(12), 883-892.
| Crossref | Google Scholar |
Major J, Lehmann J, Rondon M, Goodale C (2010) Fate of soil‐applied black carbon: downward migration leaching and soil respiration. Global Change Biology 16(4), 1366-1379.
| Crossref | Google Scholar |
Murphy BP, Prior LD, Cochrane MA, Williamson GJ, Bowman D (2019) Biomass consumption by surface fires across Earth’s most fire prone continent. Global Change Biology 25(1), 254-268.
| Crossref | Google Scholar | PubMed |
Nguyen BT, Lehmann J, Kinyangi J, Smernik R, Riha SJ, Engelhard MH (2009) Long-term black carbon dynamics in cultivated soil. Biogeochemistry 92, 163-176.
| Crossref | Google Scholar |
North Australia and Rangelands Fire Information (NAFI) (2023) Long Term Fire Frequency. Available at https://firenorthorgau/nafi3/downloads/firehistory/Since_2000/250m%20pixel%202000-2022_Long%20Term%20Fire%20Frequency_Shapefilezip [accessed 21 November 2023].
Perry JJ, Cook GD, Graham E, Meyer CM, Murphy HT, VanDerWal J (2019) Regional seasonality of fire size and fire weather conditions across Australia’s northern savanna. International Journal of Wildland Fire 29(1), 1-10.
| Crossref | Google Scholar |
Reisser M, Purves RS, Schmidt MW, Abiven S (2016) Pyrogenic carbon in soils: a literature-based inventory and a global estimation of its content in soil organic carbon and stocks. Frontiers in Earth Science 4, 80.
| Crossref | Google Scholar |
Richards AE, Cook GD, Lynch BT (2011) Optimal fire regimes for soil carbon storage in tropical savannas of northern Australia. Ecosystems 14(3), 503-518.
| Crossref | Google Scholar |
Russell-Smith J, Cook GD, Cooke PM, Edwards AC, Lendrum M, Meyer CP, Whitehead PJ (2013) Managing fire regimes in north Australian savannas: applying Aboriginal approaches to contemporary global problems. Frontiers in Ecology and the Environment 11(s1), e55-e63.
| Crossref | Google Scholar |
Saiz G, Goodrick I, Wurster CM, Zimmermann M, Nelson PN, Bird MI (2014) Charcoal re-combustion efficiency in tropical savannas. Geoderma 219, 40-45.
| Crossref | Google Scholar |
Saiz G, Wynn JG, Wurster CM, Goodrick I, Nelson PN, Bird MI (2015) Pyrogenic carbon from tropical savanna burning: production and stable isotope composition. Biogeosciences 12(6), 1849-1863.
| Crossref | Google Scholar |
Saiz G, Goodrick I, Wurster C, Nelson PN, Wynn J, Bird M (2018) Preferential production and transport of grass-derived pyrogenic carbon in NE-Australian savanna ecosystems. Frontiers in Earth Science 5,.
| Crossref | Google Scholar |
Sanderman J, Baldock JA, Dangal SR, Ludwig S, Potter S, Rivard C, Savage K (2021a) Soil organic carbon fractions in the Great Plains of the United States: an application of mid-infrared spectroscopy. Biogeochemistry 156(1), 97-114.
| Crossref | Google Scholar |
Sanderman J, Savage K, Dangal SR, Duran G, Rivard C, Cavigelli MA, Gollany HT, Jin VL, Liebig MA, Omondi EC, Rui Y (2021b) Can agricultural management induced changes in soil organic carbon be detected using mid-infrared spectroscopy? Remote Sensing 13(12), 2265.
| Crossref | Google Scholar |
Sangha KK, Evans J, Edwards A, Russell-Smith J, Fisher R, Yates C, Costanza R (2021) Assessing the value of ecosystem services delivered by prescribed fire management in Australian tropical savannas. Ecosystem Services 51, 101343.
| Crossref | Google Scholar |
Viscarra Rossel RA, Lee J, Behrens T, Luo Z, Baldock J, Richards A (2019) Continental-scale soil carbon composition and vulnerability modulated by regional environmental controls. Nature Geoscience 12(7), 547-552.
| Crossref | Google Scholar |
Whitehead PJ, Russell-Smith J, Yates C (2014) Fire patterns in north Australian savannas: extending the reach of incentives for savanna fire emissions abatement. The Rangeland Journal 36(4), 371-388.
| Crossref | Google Scholar |
Woolf D, Amonette JE, Street-Perrott FA, Lehmann J, Joseph S (2010) Sustainable biochar to mitigate global climate change. Nature Communications 1(1), 56.
| Crossref | Google Scholar | PubMed |
Wurster CM, Lloyd J, Goodrick I, Saiz G, Bird MI (2012) Quantifying the abundance and stable isotope composition of pyrogenic carbon using hydrogen pyrolysis. Rapid Communications in Mass Spectrometrys 26(23), 2690-2696.
| Crossref | Google Scholar | PubMed |
Wurster CM, Saiz G, Schneider MP, Schmidt MW, Bird MI (2013) Quantifying pyrogenic carbon from thermosequences of wood and grass using hydrogen pyrolysis. Organic Geochemistry 62, 28-32.
| Crossref | Google Scholar |
Wynn JG, Bird MI, Vellen L, Grand‐Clement E, Carter J, Berry SL (2006) Continental-scale measurement of the soil organic carbon pool with climatic, edaphic, and biotic controls. Global Biogeochemical Cycles 20(1), GB1007.
| Crossref | Google Scholar |