A systematic approach to soil carbon inventory on rangelands
Shawn W. Salley
A
B
Abstract
Significant and lasting soil carbon change in rangeland ecosystems requires ecological state change. Although within-ecological state, soil carbon dynamics can occur, they are driven primarily by short-term fluctuations in weather, specifically precipitation, and are insufficient to provide reliable estimates of change to support policy and management decisions. Changes in grazing management typically do not result in ecological state change, apart from the vegetation structural change associated with long-term overgrazing. Dominant vegetation attributes such as shrub-to-grass ratios, cool season versus warm season plant production, and annual versus perennial growth habit define ecological state and are detectable accurately and cost-effectively using existing remote-sensing technology. These vegetation attributes, along with stationary soil properties, allow for mapping at scales consistent with a variety of policy and management decisions and provide a logical basis for developing a credible sampling framework for verification. Furthermore, state-transition models of ecological state dynamics are designed to provide information that can be used to support inventories and management decisions for soil carbon and other ecosystem services.
Keywords: ecological restoration, ecological site, ecological state, ecosystem services, greenhouse gas management, livestock grazing management, nature accounting, rangeland soil carbon, resource inventories, shrub invasion, state transition model.
Introduction
Rangeland terrestrial ecosystems comprise grasses, forbs, and shrubs, make up more than 25% (~470 Mha) of the earth’s land surface (Henderson et al. 2015) and provide many valuable ecosystem services, both for residents and non-residents (Briske et al. 2023). The link between land management and the provision of ecosystem services is comparatively straightforward for commodities and services consumed by resident societies, but non-residents are increasingly important consumers of rangeland ecosystem services. When those commodities are distributed and consumed off-site (for instance, food and fibre), systems for establishing value and regulating transactions are established and accepted. However, many ecosystem services provided by rangelands (such as soil carbon sequestration) can benefit society while remaining onsite, and rangeland owners, managers and policy makers are increasingly interested in establishing systems for valuing and exchanging ecosystem services that remain onsite (Society for Range Management 2023).
To address the negative effects of high concentrations of greenhouse gases (GHG) in the atmosphere resulting from human activities, most major economic sectors have responded to public concerns and government incentives by identifying opportunities for emission reductions or carbon sequestration (NCBA 2021; AMLB 2023). Whereas these industry strategies encompass the entire livestock supply chain, rangeland management has the following two major opportunities specific to grazing systems and operations on rangelands to contribute to lowering atmospheric GHG concentrations: (1) reducing methane emissions from enteric fermentation (Hristov et al. 2014) and (2) sequestering atmospheric carbon in rangeland soils and vegetation (Sanderson et al. 2020). In this paper, we focus on the management options for reducing carbon losses from rangeland soils and on increasing sequestration of atmospheric carbon in depleted rangeland soils, as a basis for designing a soil carbon inventory and monitoring system.
A large proportion of the total terrestrial soil carbon stock is contained in rangeland ecosystems. Soil organic carbon (SOC) in all of world’s ecosystems is estimated to be 1500 Pg (Batjes 1996), whereas SOC contained in rangeland soils is estimated to be about a third of that total (Schuman et al. 2002), with some estimates being as high as 50% of global soil carbon stocks (Mokany et al. 2006; Li et al. 2018). Although often overlooked (Raza et al. 2024), soil inorganic carbon (SIC) also comprises a significant component of global soil carbon, with estimates of 695–1738 Pg within the top 1 m and 2255 Pg within the top 2 m (Eswaran et al. 1995; Batjes 1996). When SIC is combined with estimates of bicarbonate in groundwater (~1400 Pg C), these two components make up the largest terrestrial carbon pool (Monger et al. 2015). SIC is generally considered to change slowly over geologic times and depends highly on soil age (Gile et al. 1966; Monger and Martinez-Rios 2000); however, SIC stocks are sensitive to land-use changes, exhumation, and soil acidification (Mikhailova and Post 2006; Yang et al. 2012), with some estimates of SIC stocks deeper than 7 m shown to be vulnerable to loss (Kim et al. 2020). Because rangelands contain a significant amount of SIC (in addition to SOC), they are especially crucial ecosystems with respect to carbon management.
Because rangelands are not tilled or logged, most soil profiles have remained largely intact and SOC stocks are likely to be near their potential natural climatic equilibrium or, at a minimum, have maintained the capacity for recovering carbon stocks. Even in areas where inappropriate management has reduced existing carbon stocks, the soil-geomorphic template (or the materials and processes of the soil, topography and parent materials, Monger and Bestelmeyer 2006) necessary to recover previous carbon concentrations still exists, with an obvious exception at locations where the soil function has been severely degraded. Thus, a clear understanding of ecological processes that drive soil carbon dynamics and elucidation of the detectable attributes that reflect those processes are critical to designing a credible inventory system if the goal is to optimise soil carbon storage in rangelands.
Soil carbon losses in rangeland ecosystems are generally attributed to inappropriate livestock grazing in the form of overstocking, change in the fire dynamics, and/or improper season of use (Follett 2001). Excessive harvest by grazing animals, native or domestic, can result in reduced plant production, limiting organic matter inputs, and alterations of nutrient-cycling relationships. Indirectly, inappropriate season of use can change plant species composition to a mix less suited to the soil and climate conditions, leading to reduce primary productivity (McSherry and Ritchie 2013). Not all rangeland ecosystems are losing soil carbon. Many rangelands are characterised by a dynamic mix of grasses and woody plants, typically shrubs, that can complicate soil carbon dynamics. Shrub increase is a global challenge that can reduce forage production, alter hydrology, affect wildlife habitat, and change in the fire dynamics negatively affect a host of rangeland-based ecosystem services, both on-site and off-site (Archer and Predick 2014; Sala et al. 2017). However, expansion of shrub cover in rangelands has generally been associated with increased concentrations of both above- and below-ground carbon (Throop et al. 2022). However, in other systems, the invasion by exotic annual grasses, confounded with changes in fire regime and loss of native shrubs, has dramatically decreased soil carbon (TM Maxwell, HE Quicke, SJ Price, MJ Germino, unpubl. data) within a short period of time.
Although the dynamics of soil carbon change can vary greatly depending on the particular rangeland ecosystem, one common theme consistently emerge, namely, the extreme variability in both space and time (Reeder 2003; Wang et al. 2021). Nearly a century of experimental and observational research has strengthened our understanding of these fine-scaled attributes and their interactions as they affect multiple ecological functions at the management scale. But this enhanced understanding has yet to produce a universally accepted methodology to reliably predict soil carbon changes in response to minor changes in management (Wang et al. 2021), even though they may affect enterprise-scale economic performance.
Changes in land use (i.e. conversion of rangeland to cropland or cropland to rangeland) result in predictable soil carbon concentration changes within a climatic region over decadal time scales (Gebhart et al. 1994). But within the broad set of commonly accepted sustainable grazing-management practices, such as those defined by appropriate stocking rate, season of use, grazing distribution and animal grazing kind, predicting the effects on soil carbon stock change year-to-year is unreliable, with limited ability of detection.
Measuring soil carbon to support land management decisions
The global extent of the large rangeland soil carbon pool (~500 Pg) demands accurate inventories that can be used as a basis for policy and program implementation via management decisions. With the exception of oceans, the amount of carbon stored in rangeland soils constitutes the second-largest organic carbon pool, exceeding all other terrestrial sources (Batjes 1996; Conant et al. 2017). The sheer size of this pool and the dynamics compared with other carbon repositories (oceans, atmosphere, cropland soils, forest soils) and annual human emissions (~10 Pg/year; GML 2024) demands accounting systems with sufficient accuracy to inspire confidence and a level of precision that can distinguish among policy and management alternatives. The public sector investments in GHG accounting should be scientifically credible enough, so as to guide private sector actions, both buying and selling, towards achieving public benefit.
Likewise, soil carbon information at an individual management scale (ha to km2) can be important for feedback to decision-making. Although time lags preclude using soil carbon laboratory data in near real time, the link between strategic management and soil carbon has important implications for advisors and managers. Increased organic carbon concentrations are often associated with enhanced ecosystem function such as soil and site stability, hydrologic function, and biotic integrity (Lal 2004), and generally reflect commonly accepted sustainable management regimes (Follett 2001). Proven grazing management strategies and practices to reliably affect soil carbon would certainly be helpful in guiding site-specific management decisions.
Empirical observations that purport to support grazing management strategies to increase soil carbon are inconsistent, and frequently fail to specify operational aspects of grazing management that can lead to soil carbon changes. In an eastern Texas USA grassland (Teague et al. 2011), an observational study of ranches that had been grazed differently for at least 9 years failed to find differences in soil carbon attributable to grazing management beyond ‘heavy continuous’ versus all other treatments (including grazing exclusion). That study area ranged from 800 to 1000 mm average annual precipitation across different soil types, representing the mesic end of rangeland ecosystems. Similarly, Moore et al. (2023), in an area of central Texas USA (average precipitation 780 mm), could not distinguish the effects of a variety of grazing management treatments in place over 12 years on soil carbon concentrations. These observational reconstructions have not contributed to specific recommendations of new management practices or generated testable hypotheses to advance research and development. Derner et al. (2019), on the basis of a more than 70 year-long controlled study of soil and vegetation properties in a short-grass prairie (north-eastern Colorado, USA, 340 mm average annual precipitation), concluded that grazing management had no discernable effect on soil carbon stocks within the range of practices that are economically sustainable, including grazing exclusion.
In Australia, several long-term studies have specifically examined the interactions of common grazing management practices and soil carbon change. Orgill et al. (2017) found that the effects of grazing management were more likely to be expressed in vegetation change (cover and species composition) than directly as soil carbon, with ecosystem variability often conditioned based on the landscape position and proximity to woody plants. Similarly, in a tropical woodland ecosystem, the overriding influence of soil carbon variability (>80%) was attributable to climatic attributes (e.g. temperature and vapour pressure deficient) across several soil types and livestock grazing management regimes (Allen et al. 2013). Within the context of climate attributes, the ecosystem vegetation attributes reflecting the long-term influences of grazing management (such as standing dry material and grass species) were found to be more reliable indicators of soil carbon. Previously, Pringle et al. (2011), in the same region, suggested via modelling that soil type, followed by tree-to-grass ratios, would be a major influence on soil carbon and a critical factor in constructing an accurate inventory system.
In a recent review of experimental results across Australia, McDonald et al. (2023) used a meta-analysis approach to conclude that grazing management (both stocking intensity and method) can have positive effects on a host of environmental and production benefits, yet were poorly connected to soil carbon change. In a recent global literature synthesis, Conant et al. (2017) examined several common management practices that could influence soil carbon concentrations. Grazing management was found as the least effective, with less than 0.3 Mg C/ha.year, and with a high degree of uncertainty. Results of these and other syntheses (see Hawkins 2017; Hawkins et al. 2017; Venter et al. 2019) cast doubt on the importance of grazing management as a direct influence on factors that govern soil carbon. Certainly, poor management (of any kind) can degrade land; however, within the broad context of proper land-use practices, it does not appear that manipulating livestock has a predictable effect on soil carbon.
Even with highly specific, experimentally based recommendations for grazing management practices, a typology of grazing management has not yet emerged that could guide implementation or analysis. A recent review of the multidecade adoption patterns of grazing management strategies across the US based on land manager surveys (O’Hara et al. 2023) failed to clearly detect practice adoption, and was severely limited by a lack of information at the most basic level about what practitioners are actually doing with livestock grazing management. Although several authors have proposed a more systematic approach to classifying grazing management regimes (see Stanley et al. 2024), there remains a concerning lack of evidence, within the overall framework of appropriate stocking rate and season of use, that subtle alterations to frequency and duration of grazing periods has a significant impact on important soil carbon-related ecological functions such as primary production (see reviews by Hawkins 2017; McDonald et al. 2023; Ren et al. 2024). Even with a more precise definition of grazing practice, the confounding array of management objectives, temporal and spatial variability, and management history are likely to preclude a more quantitative understanding that could support ranch-level decision and implementation, and importantly, monitoring at regional and national scales (Roche et al. 2015). Grazing management is a critical element of livestock production systems and ultimately financial performance, but it is not a sufficient driver of soil carbon dynamics to serve as a basis for either management guidance or a transparent, analytical monitoring system.
Conversely, the spatial template within which land-management decisions are implemented has been demonstrated to have a consistent predictive capacity in framing soil carbon dynamics, regardless of scale (Bird et al. 2002; Booker et al. 2013; Hunt et al. 2016; Miller et al. 2019; Sarkar et al. 2020; Aoyama et al. 2022; McKenna et al. 2022). Soil properties, even within a landscape or catena, can vary significantly in texture (clay vs sand), hydrologic context (shallow uplands vs run-on lowlands), depth, aspect and moisture holding capacity, all of which have been shown to dramatically affect soil carbon-storage potential (Lal et al. 2018). Fortunately, there are several land classification schemes that can be used to group these complex biotic and abiotic factors that affect soil carbon dynamics into more manageable units (Aoyama et al. 2022).
Ecological sites are management-scale groupings that combine soil properties and landscape position, within a climate zone, to delineate and define units that exhibit similar ecological behaviours (Brown and Havstad 2016). These units, while conceptual in nature, are sufficiently well defined by quantifiable soil properties to provide a framework for describing how management history, weather patterns, and management actions can interact to influence ecological outcomes. Each ecological site has a unique description of vegetation dynamics, generally in the form of a state-and-transition model, that is relevant to management decisions influencing ecological function (Brown and MacLeod 2012; Bestelmeyer et al. 2017). Those ecological functions (hydrology, life-form level productivity) typically are drivers of attributes such as soil organic carbon and can be integrated into interpretive, predictive models (Williams et al. 2016). Such site-based frameworks can support unique, property-scale inventory and management decisions for a variety of ecosystem services, including soil carbon, and have been applied in a variety of ecosystems around the world (Bestelmeyer et al. 2017). Integrating soil carbon dynamics into such a framework has been demonstrated as a proof-of-concept but requires widespread adoption and refinement to improve reliability and further development (Aoyama et al. 2022). These spatially distinct units can be used to support property-scale decision-making, but require a more integrative approach to credibly scale site-level attributes to catchment- and regional-scale outcomes, especially for larger-scale processes with public benefits (i.e. water quality, wildlife habitat).
Even when spatial variability in the drivers of soil carbon dynamics is accounted for (see Pringle et al. 2011), substantial temporal variation can occur, generally in response to precipitation patterns (Wang et al. 2018; Kaveh et al. 2022; Wang et al. 2022). Year-to-year, soil carbon fluxes can vary from losses to gains in response to precipitation patterns, independent of changes in management (Svejcar et al. 2008). In a multi-site, multi-year North American network using eddy-covariance methods to estimate carbon fluxes, highly productive tallgrass prairie sites demonstrated carbon fluxes between −1.5 and 4.3 Mg C/ha.year; mixed-grass prairie ranged from −1.3 Mg to 2.3 Mg C/ha.year; shrub steppe sites ranged from −0.6 to 2.6 T C.ha; and desert grassland sites ranged from −2.5 to 0.9 Mg C/ha.year (see Svejcar et al. 2008). Soil carbon fluxes can occur at within-season timescales that preclude management responses, even those intended to maximise soil carbon gain, increasing the emphasis on flexible, moderate stocking to minimise overgrazing and subsequent carbon losses during drought (Morgan et al. 2016).
In a similar eddy covariance network study using 25 sites over 10 years, Biederman et al. (2017) documented ecosystem fluxes between −3.5 and 3.3 Mg C/ha.year in a range of dryland ecosystems. More than half of the sites switched between carbon source and sink during the course of the study, primarily driven by changes in precipitation and temperature, across a wide range of vegetation types, with Mediterranean and Monsoon systems exhibiting the greatest yearly variability. Multi-year network studies deploying highly precise measurement technologies, although limited in extent, have clearly demonstrated the overwhelming influence of year-to-year and within-season precipitation on the direction of carbon fluxes. Fluxes in the order of −3.0 to 3.0 Mg C/ha.year were common, and even in years when precipitation was close to long-term averages, substantial fluxes were the rule.
Effectively tracking soil carbon changes to inform policy and management requires focusing on the most influential factors, namely, multi-decadal climate, soil-geomorphology, vegetation structure, and annual precipitation (in order of decreasing importance). Although proper grazing management is essential for sustainable rangelands, its impact on short-term carbon fluxes appears minimal. Instead, mid- to long-term changes in vegetation structure (ecological state) serve as a more reliable and readily detectable indicator of carbon dynamics.
An inventory system to improve policy and management
These persistent challenges complicate the development of credible methodologies for quantification of soil carbon’s temporal change that are both accurate enough for large-scale inventories (increasing or declining), and precise enough to guide management (how practice affects carbon). Generally, the following three approaches (and combinations) have been deployed for SOC inventory: direct measurements, remote-sensing, and simulation modelling (Paul et al. 2023). Direct measurements are the most reliable method, yet are costly, time consuming, and require a sufficiently large sample number to both quantify and detect SOC change, with results requiring interpretation within the context of the high spatial and temporal variability. Remote sensing is potentially cost-effective but requires precise calibration and validation, with limited success detecting field-specific trends. Many SOC simulation models are readily available (Parton et al. 1987; Coleman and Jenkinson 1996; Campbell and Paustian 2015) and considered cheaper than direct sampling, yet simulation models can result in large uncertainty (often encompassing both losses and gains), depending on their complexity, calibration, and input data. Finally, even if the above challenges for accurate SOC detection and quantification could reasonably be overcome, ascertaining the drivers of SOC change as a basis for policy development and management response remains a challenge because of the lack of readily available knowledge regarding historical land use and management.
Soil carbon inventory sampling should follow a systematic sampling strategy based on climate, geomorphology, and soil potential. Although mostly known (and often predictable), multiple confounding physical, chemical, and biological properties condition soil carbon. Capturing this variability requires a strategic, hierarchical sampling strategy across multiple scales to accurately measure soil carbon dynamics. Regionalisation products such as hierarchical land resource classifications organise major trends, cycles, and patterns of soil occurrence (Salley et al. 2016) and provide a logical framework to estimate mean soil carbon trends. This framework can generate smaller variances for the estimated mean if coupled with existing soil survey data that can provide estimates of within stratum variances (Cochran 1977). However, the overall magnitude of potential carbon variability among strata necessitates stratification of the variance of carbon change versus only a total baseline of soil carbon. Baseline carbon stocks and rates of carbon stock change are different variables with differing variability and interpretations. Because change is considered an additive effect, potentially influenced by factors independent of or related to the starting amount of carbon, resampling of well-defined monitoring sites is crucial for both baseline and follow-up measurements (Lark 2009).
Once strata and sites are identified, sampling strategies must balance the frequently competing objectives of using field data to parametrise carbon simulation models versus sampling the variability of similar soils for experimental tests of management treatments. Sampling approaches intended to parameterise and validate SOC simulation models focus on maximising change detection, while minimising the spatial variability at an individual location. In practice, this has guided methods that collect multiple sets of composited samples within a small area to minimise the variance of soil carbon (Spencer et al. 2011). Although necessary to support carbon simulation model development and validation, focused composited sampling intrinsically fails to attain soil carbon variability of the soil landscape or management unit (e.g. field or paddock). Model parameterisation primarily focuses on exploring and quantifying the variability, while sampling as part of a designed experiment is directed toward minimising variability around means.
Capturing the within-field variability (experimental comparisons) requires a priori knowledge of both the soil landscape (ecological site), land use and conservation practices (ecological state). Ecological site is determined by static landscape and soil properties, found at varying levels of detail in common soil maps. Ecological state, defined as the dominant vegetation characteristics (shrub-to-grass ratios, cool season vs warm season plants, and annual vs perennial growth habit), is detectable accurately and cost-effectively using field identification or existing remote sensing technology. Once an experimental or management boundary is determined, soil survey map- derived data or remotely sensed data (such as topography or normalised difference vegetation index) can be used to further stratify the within-site variability, although additional in-field assessments will likely be necessary to verify ecological state and identify other information relevant to interpretations (i.e. soil erosion).
Although there is currently not a national or regional application using site-specific state-and-transition models to predict changes in rangeland soil carbon, several examples are available. Even in arid environments, change in herbaceous dominance owing to extended heavy grazing use can result in significant soil carbon change (Fig. 1). Effects of state change extend throughout the soil profile and usually occur because of excessive grazing pressure over multiple years concurrent with multi-year drought. In this case, soil carbon concentrations (% SOC) measured in the top 5 cm differ by a factor of two. A similar approach has been extended to multiple ecosystem services (Fig. 2) in a Mediterranean savanna across three distinct ecological states on a loamy site that is often treated mechanically or chemically to reduce (savanna state) or eliminate (grassland state) oak trees. In this case, grassland-dominated sites have higher agricultural value, expressed as forage productivity and monetary value (USD per hectare), whereas savannas and woodlands have higher watershed and biodiversity values. A more comprehensive approach is underway in Australia via the Land Accounts in the National Ecosystem Accounts (see environment.gov.au).
Example of soil organic carbon change by depth in a Chihuahuan Desert (250 mm average annual precipitation) Clayey ecological site expressed as ecological state change. State 1. Tabosa (Hilaria mutica); and State 2. Burrograss (Scleropogon brevifolius)-dominated ecological states are only two of five possible ecological states defined for this site. Figure courtesy of Dr. Jeffrey Herrick (unpubl. data).
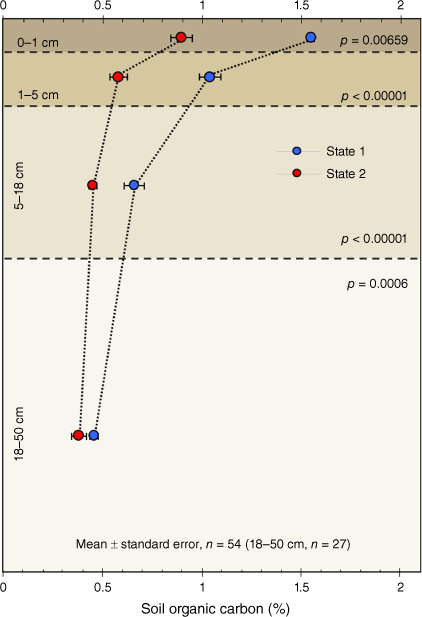
Example of ecosystem service changes by ecological state changes in a California oak savanna ecological site. Soil organic carbon (SOC, yellow line) pools for the A horizon of each state were calculated as 27.5 ± 0.18 Mg C/ha for savanna, 32.4 ± 0.13 Mg C/ha for woodland, and 20.98 ± 0.08 Mg C/ha for grassland. Figure is redrawn from Eastburn et al. (2017), displaying the provisioning of multiple ecosystem services across agricultural production (forage and cost per hectare), soil health (carbon, nitrogen, and infiltration), and habitat biodiversity (plant diversity, richness, and resistance to invasives).
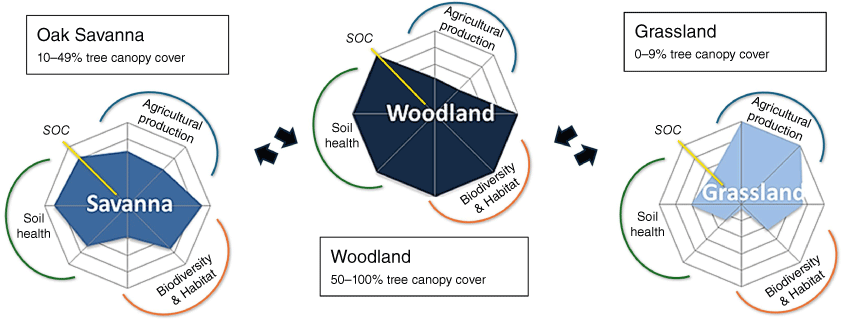
Repeat soil sampling is one strategy used to monitor soil carbon concentration, soil carbon change, and to estimate soil carbon stocks (FAO 2019); however, as previously discussed, the magnitude, direction, and uncertainty of rangeland SOC changes can differ greatly on decadal and centennial timescales (Piñeiro et al. 2006; Dean et al. 2012). This makes attribution of any observed changes in soil carbon to specific drives (management, climate change) very difficult (Smith 2004). Space-for-time sampling is often used to account for temporal changes because it allows for derivation of SOC dynamics beyond the time period during which observations are made (Dane and Topp 2020; Sharma et al. 2024). Although space-for-time approaches minimise the temporal variability, they lacks directional soil carbon change. Where temporal drivers and dynamics between ecological states are reasonably understood, ecological states serve as a logical stratification for sampling strategies. However, spatial heterogeneity and improperly pairing soils (i.e. where soil properties by depth are not as predictable) may limit overall space-for-time sampling inference, reinforcing the need for integrated research through long-term experiments to understand decadal to centurial soil change (Tugel et al. 2005).
On the basis of the preceding synthesis of the relevant literature, we suggest that agglomerative, opportunistic, ad hoc inventories of rangeland management practices, long-term climate, and general soil properties will not provide direct actionable information for either policymakers or land managers. Existing inventories of land condition and/or practice implementation are not sufficiently sensitive to the changes in ecological state and short-term climate variability to reliably track changes in rangeland soil carbon. Attaching soil carbon estimates to existing inventory frameworks lacks the accuracy needed to set long-term (decade-scale) goals and implement programs to improve carbon storage. Additionally, these data would not be detailed enough to provide practical guidance for individual land managers or show how these actions might affect other rangeland ecosystem services.
Capturing dynamics relevant to on-the-ground management decisions is an important component of developing credible inventories. In turn, those inventories can be indispensable in developing national and regional policies that encourage land managers to manage for a wide variety of ecosystem services, including GHG emission reduction and carbon storage. Although methods outlined in the previous section incorporate somewhat quantifiable landscape properties, our goal should be to have the most reliable information at the landscape scale (field or paddock), where policy can be implemented by specific practices. The basis of this system is the assumption that sampling strata will account for individual climate, geomorphic, topo-edaphic, and ecological-state combinations; however, scaling management concepts to the landscape scale will require mapping at the ecological-state level.
Ecological sites, and specific, unique state-and-transition models (STMs), are mapped using landscape position and edaphic properties (Duniway et al. 2010). In the USA, more than 90% of soils on rangelands are mapped at a scale sufficient to confidently assign and verify ecological sites; where standardised soil maps are not available, landscape position or soil properties are easily discernable by practitioners after a minimum of training. Each ecological site has a unique STM that describes temporal dynamics in terms of vegetation relationships that are common for the site. Ecological states can be mapped either based on field work or remote-sensing (Steele et al. 2012; Maynard and Levi 2017; Nauman et al. 2022) at a highly accurate level. Although distributing soil carbon values from field measurements or estimates to the landscape scale by using ecological states does not require complex mathematics, it does require lots of data to understand the range of values associated with ecological state.
More importantly, STMs can be used to identify priority areas for targeting efforts to avoid carbon loss or increase sequestration by changing vegetation. For example, Maxwell and Germino (2022) and Maxwell et al. (TM Maxwell, HE Quicke, SJ Price, MJ Germino, unpubl. data) have demonstrated the effects of specific combinations of invasive annual grasses and changes in fire regime on the loss of shrubs, and subsequent effects on soil carbon concentrations in the North American Great Basin. The plant communities associated with those processes and easily discernable indicators are easily definable with existing remote-sensing technology and are common components in STMs. STMs also include specific recommendations about how land managers can avoid undesirable or accelerate desirable ecological-state change by implementing well-defined management practices. The descriptions of those practices are the bases for widely used land conservation programs in the US Department of Agriculture and US Department of Interior (Natural Resources Conservation Service 2024).
Soil carbon is most unlikely to be the major factor in determining land management objectives or management decisions, at either the policy or operational levels (Brown et al. 2010), but will be an increasingly important factor within the context of the current land use of livestock grazing. Unrealistic expectations of land managers on potential change in soil carbon, driven by promotion of selected results, means that this is not always the case. Fortunately, optimum soil carbon dynamics are usually associated with a host of other rangeland management objectives and ecosystem services (Booker et al. 2013; Sanderson et al. 2020; Briske et al. 2023), also known as ‘good rangeland management’. However, the need for a credible system to assess and value the range of ecosystem services (including soil carbon) that can be delivered to a market, whether private or public sector, is a necessary part of policy development and implementation via land management.
There is a substantial effort underway within Australia to use State-and-Transition Models as a basis for inventory, management and policy-making for a variety of ecosystem services (Richards et al. 2020; Prober et al. 2023). This comprehensive accounting approach reflects the temporal dynamics of a variety of ecosystem services (including soil carbon) associated with changes in ecological state. However, we agree with the authors that credibly tracking soil carbon will require more attention to the inherent edaphic spatial variability in soil carbon stocks, which can be exacerbated by ecological-state change at small scales (Richards et al. 2024).
Conclusions
Our approach in this paper has been to focus on the reliably detectable indicators of rangeland soil carbon as a framework for building a credible inventory system. Ultimately, a system that can support policy, program and management decision-making has to deliver accurate information at the smallest scale (points within paddocks) and be logically scalable through other levels. We believe that an inventory system based on site-specific climatic and edaphic attributes, with accurate State and Transition Models, as the basis for estimating soil carbon concentrations can meet these goals. An added benefit is that ecological state level soil and vegetation attributes are generally used to parameterise models to estimate ecosystem service outcomes of land management actions (Webb et al. 2024). The STM framework can also be used as a basis for downscaling regional climate drivers to farm and paddock level to assess priorities for management intervention (Nauman et al. 2015). These fine-scaled soil and vegetation dynamics that we have described are the most accurate means of reflecting the interactions of ecology and land management available today.
Conflicts of interest
JRB is a member of The Rangeland Journal Advisory Committee. To mitigate this potential conflicts of interest he had no editor-level access to this manuscript during peer review. The author(s) have no further conflicts of interest to declare.
Acknowledgements
We thank Paul Novelly, Katherine Dynarski, and Jonathan Maynard for constructive comments and suggestions that greatly improved the final manuscript.
References
Allen DE, Pringle MJ, Bray S, Hall TJ, O’Reagain PO, Phelps D, Cobon DH, Bloesch PM, Dalal RC (2013) What determines soil organic carbon stocks in the grazing lands of north-eastern Australia? Soil Research 51, 695-706.
| Crossref | Google Scholar |
AMLB (2023) ‘Carbon neutral 2030 R&D | Meat & Livestock Australia.’ (MLA Corporate) Available at https://www.mla.com.au/research-and-development/Environment-sustainability/carbon-neutral-2030-rd/ [verified 12 March 2024]
Aoyama L, Bartolome J, Silva L, Silver WL (2022) Using ecological site descriptions to make ranch-level decisions about where to manage for soil organic carbon. California Agriculture 76, 85-92.
| Crossref | Google Scholar |
Archer SR, Predick KI (2014) An ecosystem services perspective on brush management: research priorities for competing land-use objectives. Journal of Ecology 102, 1394-1407.
| Crossref | Google Scholar |
Batjes NH (1996) Total carbon and nitrogen in the soils of the world. European Journal of Soil Science 47, 151-163.
| Crossref | Google Scholar |
Bestelmeyer BT, Ash A, Brown JR, Densambuu B, Fernández-Giménez M, Johanson J, Levi M, Lopez D, Peinetti R, Rumpff L, Shaver P (2017) State and Transition Models: theory, applications, and challenges. In ‘Rangeland systems’. Springer Series on Environmental Management. (Ed. DD Briske) pp. 303–345. (Springer International Publishing: Cham, Switzerland) 10.1007/978-3-319-46709-2_9
Biederman JA, Scott RL, Bell TW, Bowling DR, Dore S, Garatuza‐Payan J, Kolb TE (2017) CO2 exchange and evapotranspiration across dryland ecosystems of southwestern North America. Global Change Biology 23(10), 4204-4221.
| Crossref | Google Scholar | PubMed |
Bird SB, Herrick JE, Wander MM, Wright SF (2002) Spatial heterogeneity of aggregate stability and soil carbon in semi-arid rangeland. Environmental Pollution 116, 445-455.
| Crossref | Google Scholar | PubMed |
Booker K, Huntsinger L, Bartolome JW, Sayre NF, Stewart W (2013) What can ecological science tell us about opportunities for carbon sequestration on arid rangelands in the United States? Global Environmental Change 23, 240-251.
| Crossref | Google Scholar |
Briske DD, Archer SR, Burchfield E, Burnidge W, Derner JD, Gosnell H, Hatfield J, Kazanski CE, Khalil M, Lark TJ, Nagler P, Sala O, Sayre NF, Stackhouse-Lawson KR (2023) Supplying ecosystem services on US rangelands. Nature Sustainability 6, 1524-1532.
| Crossref | Google Scholar |
Brown JR, Havstad KM (2016) Using ecological site information to improve landscape management for ecosystem services. Rangelands 38, 318-321.
| Crossref | Google Scholar |
Brown JR, MacLeod N (2012) A site-based approach to delivering rangeland ecosystem services. The Rangeland Journal 33, 99-108.
| Crossref | Google Scholar |
Brown J, Angerer J, Salley SW, Blaisdell R, Stuth JW (2010) Improving estimates of rangeland carbon sequestration potential in the US Southwest. Rangeland Ecology & Management 63(1), 147-154.
| Crossref | Google Scholar |
Campbell EE, Paustian K (2015) Current developments in soil organic matter modeling and the expansion of model applications: a review. Environmental Research Letters 10, 123004.
| Crossref | Google Scholar |
Coleman K, Jenkinson DS (1996) RothC-26.3 - A Model for the turnover of carbon in soil. In ‘Evaluation of soil organic matter models’. (Eds DS Powlson, P Smith, JU Smith) pp. 237–246. (Springer: Berlin, Heidelberg, Germany) 10.1007/978-3-642-61094-3_17
Conant RT, Cerri CEP, Osborne BB, Paustian K (2017) Grassland management impacts on soil carbon stocks: a new synthesis. Ecological Applications 27, 662-668.
| Crossref | Google Scholar | PubMed |
Dean C, Roxburgh SH, Harper RJ, Eldridge DJ, Watson IW, Wardell-Johnson GW (2012) Accounting for space and time in soil carbon dynamics in timbered rangelands. Ecological Engineering 38(1), 51-64.
| Crossref | Google Scholar |
Derner JD, Augustine DJ, Frank DA (2019) Does grazing matter for soil organic carbon sequestration in the western North American Great Plains? Ecosystems 22, 1088-1094.
| Crossref | Google Scholar |
Duniway MC, Bestelmeyer BT, Tugel A (2010) Soil processes and properties that distinguish ecological sites and states. Rangelands 32, 9-15.
| Crossref | Google Scholar |
Eastburn DJ, O’Geen AT, Tate KW, Roche LM (2017) Multiple ecosystem services in a working landscape. PLoS One 12(3), e0166595.
| Crossref | Google Scholar | PubMed |
Follett RF (2001) Soil management concepts and carbon sequestration in cropland soils. Soil and Tillage Research 61, 77-92.
| Crossref | Google Scholar |
Gebhart DL, Johnson HB, Mayeux HS, Polley HW (1994) The CRP increases soil organic carbon. Journal of Soil and Water Conservation 49, 488-492.
| Google Scholar |
Gile LH, Peterson FF, Grossman RB (1966) Morphological and genetic sequences of carbonate accumulation in desert soils. Soil Science 101, 347-360.
| Google Scholar |
GML (2024) Global monitoring laboratory – carbon cycle greenhouse gases. Available at https://gml.noaa.gov/ccgg/carbontracker/ [accessed 18 March 2024]
Hawkins H-J (2017) A global assessment of Holistic Planned Grazing™ compared with season-long, continuous grazing: meta-analysis findings. African Journal of Range & Forage Science 34, 65-75.
| Crossref | Google Scholar |
Hawkins H-J, Short A, Kirkman KP (2017) Does holistic planned grazing work on native rangelands? African Journal of Range & Forage Science 34(2), 59-63.
| Crossref | Google Scholar |
Henderson G, Cox F, Ganesh S, Jonker A, Young W, Janssen PH (2015) Rumen microbial community composition varies with diet and host, but a core microbiome is found across a wide geographical range. Scientific Reports 5, 14567.
| Crossref | Google Scholar | PubMed |
Hristov AN, Johnson KA, Kebreab E (2014) Livestock methane emissions in the United States. Proceedings of the National Academy of Sciences 111, E1320.
| Crossref | Google Scholar | PubMed |
Hunt JE, Laubach J, Barthel M, Fraser A, Phillips RL (2016) Carbon budgets for an irrigated intensively grazed dairy pasture and an unirrigated winter-grazed pasture. Biogeosciences 13, 2927-2944.
| Crossref | Google Scholar |
Kaveh N, Ebrahimi A, Asadi E (2022) Environmental drivers of above-ground biomass in semi-arid rangelands. The Rangeland Journal 44, 165-175.
| Crossref | Google Scholar |
Kim JH, Jobbágy EG, Richter DD, Trumbore SE, Jackson RB (2020) Agricultural acceleration of soil carbonate weathering. Global Change Biology 26, 5988-6002.
| Crossref | Google Scholar | PubMed |
Lal R (2004) Soil carbon sequestration impacts on global climate change and food security. Science 304, 1623-1627.
| Crossref | Google Scholar | PubMed |
Lal R, Smith P, Jungkunst HF, Mitsch WJ, Lehmann J, Nair PKR, McBratney AB, Sá JC, de M, Schneider J, Zinn YL, Skorupa ALA, Zhang H-L, Minasny B, Srinivasrao C, Ravindranath NH (2018) The carbon sequestration potential of terrestrial ecosystems. Journal of Soil and Water Conservation 73, 145A-152A.
| Crossref | Google Scholar |
Lark RM (2009) Estimating the regional mean status and change of soil properties: two distinct objectives for soil survey. European Journal of Soil Science 60(5), 748-56.
| Crossref | Google Scholar |
Li W, Ciais P, Guenet B, Peng S, Chang J, Chaplot V, Khudyaev S, Peregon A, Piao S, Wang Y, Yue C (2018) Temporal response of soil organic carbon after grassland‐related land‐use change. Global Change Biology 24(10), 4731-4746.
| Crossref | Google Scholar | PubMed |
Maxwell TM, Germino MJ (2022) The effects of cheatgrass invasion on US Great Basin carbon storage depend on interactions between plant community composition, precipitation seasonality, and soil climate regime. Journal of Applied Ecology 59, 2863-2873.
| Crossref | Google Scholar |
Maynard JJ, Levi MR (2017) Hyper-temporal remote sensing for digital soil mapping: characterizing soil-vegetation response to climatic variability. Geoderma 285, 94-109.
| Crossref | Google Scholar |
McDonald SE, Badgery W, Clarendon S, Orgill S, Sinclair K, Meyer R, Butchart DB, Eckard R, Rowlings D, Grace P, Doran-Browne N, Harden S, Macdonald A, Wellington M, Pachas ANA, Eisner R, Amidy M, Harrison MT (2023) Grazing management for soil carbon in Australia: a review. Journal of Environmental Management 347, 119146.
| Crossref | Google Scholar | PubMed |
McKenna DM, Grams SE, Barasha M, Antoninka AJ, Johnson NC (2022) Organic and inorganic soil carbon in a semi-arid rangeland is primarily related to abiotic factors and not livestock grazing. Geoderma 419, 115844.
| Crossref | Google Scholar |
McSherry ME, Ritchie ME (2013) Effects of grazing on grassland soil carbon: a global review. Global Change Biology 19, 1347-1357.
| Crossref | Google Scholar | PubMed |
Mikhailova EA, Post CJ (2006) Effects of land use on soil inorganic carbon stocks in the Russian Chernozem. Journal of Environmental Quality 35, 1384-1388.
| Crossref | Google Scholar | PubMed |
Miller GA, Rees RM, Griffiths BS, Ball BC, Cloy JM (2019) The sensitivity of soil organic carbon pools to land management varies depending on former tillage practices. Soil and Tillage Research 194, 104299.
| Crossref | Google Scholar |
Mokany K, Raison RJ, Prokushkin AS (2006) Critical analysis of root : shoot ratios in terrestrial biomes. Global Change Biology 12, 84-96.
| Crossref | Google Scholar |
Monger HC, Bestelmeyer BT (2006) The soil-geomorphic template and biotic change in arid and semi-arid ecosystems. Journal of Arid Environments 65, 207-218.
| Crossref | Google Scholar |
Monger HC, Martinez-Rios JJ (2000) Inorganic carbon sequestration in grazing lands, The potential of US grazing lands to sequester carbon and mitigate the greenhouse effect. In ‘The potential of US grazing lands to sequester carbon and mitigate the greenhouse effect’. (Eds RF Follett, JM Kimble) pp. 87–118. (CRC Press: Boca Raton, FL, USA)
Monger HC, Kraimer RA, Khresat S, Cole DR, Wang X, Wang J (2015) Sequestration of inorganic carbon in soil and groundwater. Geology 43, 375-378.
| Crossref | Google Scholar |
Moore JM, Manter DK, Maczko KA (2023) Rotational grazing strategies minimally impact soil microbial communities and carbon vynamics – a Texas case study. Land 12, 1517.
| Crossref | Google Scholar |
Morgan JA, Parton W, Derner JD, Gilmanov TG, Smith DP (2016) Importance of early season conditions and grazing on carbon dioxide fluxes in Colorado shortgrass steppe. Rangeland Ecology & Management 69, 342-350.
| Crossref | Google Scholar |
Natural Resources Conservation Service (2024) Ecological site descriptions | Natural Resources Conservation Service (usda.gov) [accessed 18 April 2024]
Nauman TW, Thompson JA, Teets J, Dilliplane T, Bell JW, Connolly SJ, Liebermann HJ, Yoast K (2015) Pedoecological modeling to guide forest restoration using ecological site descriptions. Soil Science Society of America Journal 79, 1406-1419.
| Crossref | Google Scholar |
Nauman TW, Burch SS, Humphries JT, Knight AC, Duniway MC (2022) A quantitative soil-geomorphic framework for developing and mapping ecological site groups. Rangeland Ecology & Management 81, 9-33.
| Crossref | Google Scholar |
NCBA (2021) ‘Cattle Industry Commits to Climate Neutrality by 2040.’ (National Cattlemen’s Beef Association) Available at https://www.ncba.org/ncba-news/news-releases/news/details/27404/cattle-industry-commits-to-climate-neutrality-by-2040 [accessed 12 March 2024]
O’Hara JK, Reyes J, Knight LG, Brown J (2023) Why has the adoption of rotational grazing declined in parts of the United States? Rangelands 45, 92-101.
| Crossref | Google Scholar |
Orgill SE, Waters CM, Melville G, Toole I, Alemseged Y, Smith W (2017) Sensitivity of soil organic carbon to grazing management in the semi-arid rangelands of south-eastern Australia. The Rangeland Journal 39, 153-167.
| Crossref | Google Scholar |
Parton WJ, Schimel DS, Cole CV, Ojima DS (1987) Analysis of factors controlling soil organic matter levels in Great Plains grasslands. Soil Science Society of America Journal 51, 1173-1179.
| Crossref | Google Scholar |
Paul C, Bartkowski B, Dönmez C, Don A, Mayer S, Steffens M, Weigl S, Wiesmeier M, Wolf A, Helming K (2023) Carbon farming: are soil carbon certificates a suitable tool for climate change mitigation? Journal of Environmental Management 330, 117142.
| Crossref | Google Scholar | PubMed |
Piñeiro G, Paruelo JM, Oesterhald M (2006) Potential long-term impacts of livestock introduction on carbon and nitrogen cycling in grasslands of southern South America. Global Change Biology 12, 1267-1284.
| Crossref | Google Scholar |
Pringle MJ, Allen DE, Dalal RC, Payne JE, Mayer DG, O’Reagain P, Marchant BP (2011) Soil carbon stock in the tropical rangelands of Australia: effects of soil type and grazing pressure, and determination of sampling requirement. Geoderma 167, 261-273.
| Crossref | Google Scholar |
Prober SM, Cook G, Gosper CR, Hodgson JR, Langridge JM, Rumpff L, Williams RJ, Yates CJ, Richards AE (2023) ‘The Australian ecosystems model framework: eucalypt woodlands, Version 1.0.’ (CSIRO: Australia) Available at https://publications.csiro.au/publications/publication/PIcsiro:EP2023-2878 [accessed 19 June 2024]
Raza S, Irshad A, Margenot A, Zamanian K, Li N, Ullah S, Mehmood K, Ajmal Khan M, Siddique N, Zhou J, Mooney SJ, Kurganova I, Zhao X, Kuzyakov Y (2024) Inorganic carbon is overlooked in global soil carbon research: a bibliometric analysis. Geoderma 443, 116831.
| Crossref | Google Scholar | PubMed |
Reeder J (2003) Overcoming spatial variation in measuring carbon stocks and sequestration potential of native rangelands in the western US. (pp. 193–200) In ‘Soil Organic Carbon and Agriculture: Developing Indicators for Policy Analyses. Proceedings of an OECD Expert meeting’, Ottawa, Canada, October 2002. 329 pp. (Agriculture and Agri-Food Canada and Organisation for Economic Co-operation and Development: Ottawa)
Ren S, Terrer C, Li J, Cao Y, Yang S, Liu D (2024) Historical impacts of grazing on carbon stocks and climate mitigation opportunities. Nature Climate Change 14, 380-386.
| Crossref | Google Scholar |
Richards AE, Dickson F, Williams KJ, Cook GD, Roxburgh S, Murphy H, Doherty M, Warnick A, Metcalfe D, Prober SM (2020) ‘The Australian ecosystem models framework project: a conceptual framework.’ (CSIRO: Australia) 10.25919/f61q-1386
Ewamian Ltd, Ewamian People Aboriginal Corporation RNTBC, Richards AE, Brandon C Liu N, Mokany K, Schmidt RK, Smith GS, Williams KJ, Evans D, Ferrier S, Giljohann KM, Grainger D, Harwood TD, Hayward J, Jarvis D, Johnson S, Khan S, Lehmann EA, Liedloff AC, Murphy HT, Newnham G, Pascoe S, Roxburgh SH, Scheufele G, Stewart SB, Ewamian Ltd, Ewamian People Aboriginal Corporation RNTBC, Tagalaka Aboriginal Corporation RNTBC, Tetreault-Campbell S, Ware C (2024) ‘Experimental ecosystem accounts for the Flinders, Norman and Gilbert river catchments. A synthesis report from the Regional Ecosystem Accounting Pilot projects.’ (CSIRO) 10.25919/xeas-3102 [accessed 19 June 2024]
Roche LM, Cutts BB, Derner JD, Lubell MN, Tate KW (2015) On-ranch grazing xtrategies: context for the rotational grazing dilemma. Rangeland Ecology & Management 68, 248-256.
| Crossref | Google Scholar |
Sala OE, Yahdjian L, Havstad K, Aguiar MR (2017) Rangeland ecosystem eervices: nature’s supply and humans’ demand. In ‘Rangeland systems: processes, management and challenges’. (Ed. DD Briske) pp. 467–489. (Springer International Publishing: Cham, Switzerland) 10.1007/978-3-319-46709-2_14
Salley SW, Talbot CJ, Brown JR (2016) The Natural Resources Conservation Service land resource hierarchy and ecological sites. Soil Science Society of America Journal 80(1), 1-9.
| Crossref | Google Scholar |
Sanderson JS, Beutler C, Brown JR, Burke I, Chapman T, Conant RT, Derner JD, Easter M, Fuhlendorf SD, Grissom G, Herrick J (2020) Cattle, conservation, and carbon in the western Great Plains. Journal of Soil and Water Conservation 75, 5A-12A.
| Crossref | Google Scholar |
Sarkar R, Corriher-Olson V, Long C, Somenahally A (2020) Challenges and potentials for soil organic carbon sequestration in forage and grazing systems. Rangeland Ecology & Management 73, 786-795.
| Crossref | Google Scholar |
Schuman GE, Janzen HH, Herrick JE (2002) Soil carbon dynamics and potential carbon sequestration by rangelands. Environmental Pollution 116, 391-396.
| Crossref | Google Scholar | PubMed |
Sharma R, Levi MR, Ricker MC, Thompson A, King EG, Robertson K (2024) Scaling of soil organic carbon in space and time in the southern Coastal Plain, USA. Science of the Total Environment 933, 173060.
| Crossref | Google Scholar | PubMed |
Smith P (2004) How long before a change in soil organic carbon can be detected? Global Change Biology 10(11), 1878-1883.
| Crossref | Google Scholar |
Society for Range Management (2023) Rangeland Ecosystem Services: connecting nature and people. Available at https://rangelands.org/rangelands-provide-five-ecosystem-services/ [accessed 18 April 2024]
Spencer S, Ogle SM, Breidt FJ, Goebel JJ, Paustian K (2011) Designing a national soil carbon monitoring network to support climate change policy: a case example for US agricultural lands. Greenhouse Gas Measurement and Management 1, 167-178.
| Crossref | Google Scholar |
Stanley PL, Wilson C, Patterson E, Machmuller MB, Cotrufo MF (2024) Ruminating on soil carbon: applying current understanding to inform grazing management. Global Change Biology 30, e17223.
| Crossref | Google Scholar | PubMed |
Steele CM, Bestelmeyer BT, Burkett LM, Smith PL, Yanoff S (2012) Spatially explicit representation of State-and-Transition Models. Rangeland Ecology & Management 65, 213-222.
| Crossref | Google Scholar |
Svejcar T, Angell R, Bradford JA, Dugas W, Emmerich W, Frank AB, Gilmanov T, Haferkamp M, Johnson DA, Mayeux H, Mielnick P, Morgan J, Saliendra NZ, Schuman GE, Sims PL, Snyder K (2008) Carbon fluxes on North American rangelands. Rangeland Ecology & Management 61, 465-474.
| Crossref | Google Scholar |
Teague WR, Dowhower SL, Baker SA, Haile N, DeLaune PB, Conover DM (2011) Grazing management impacts on vegetation, soil biota and soil chemical, physical and hydrological properties in tall grass prairie. Agriculture, Ecosystems & Environment 141, 310-322.
| Crossref | Google Scholar |
Throop HL, Munson S, Hornslein N, McClaran MP (2022) Shrub influence on soil carbon and nitrogen in a semi-arid grassland is mediated by precipitation and largely insensitive to livestock grazing. Arid Land Research and Management 36, 27-46.
| Crossref | Google Scholar |
Tugel AJ, Herrick JE, Brown JR, Mausbach MJ, Puckett W, Hipple K (2005) Soil change, soil survey, and natural resources decision making: a blueprint for action. Soil Science Society of America Journal 69(3), 738-747.
| Crossref | Google Scholar |
Venter ZS, Cramer MD, Hawkins H-J (2019) Rotational grazing management has little effect on remotely-sensed vegetation characteristics across farm fence-line contrasts. Agriculutre, Ecosystems and Environment 282, 40-48.
| Crossref | Google Scholar |
Wang C, Houlton BZ, Liu D, Hou J, Cheng W, Bai E (2018) Stable isotopic constraints on global soil organic carbon turnover. Biogeosciences 15, 987-995.
| Crossref | Google Scholar |
Wang S, Xu L, Zhuang Q, He N (2021) Investigating the spatio-temporal variability of soil organic carbon stocks in different ecosystems of China. Science of The Total Environment 758, 143644.
| Crossref | Google Scholar | PubMed |
Wang M, Guo X, Zhang S, Xiao L, Mishra U, Yang Y, Zhu B, Wang G, Mao X, Qian T, Jiang T, Shi Z, Luo Z (2022) Global soil profiles indicate depth-dependent soil carbon losses under a warmer climate. Nature Communications 13, 5514.
| Crossref | Google Scholar | PubMed |
Webb NP, Edwards BL, Heller A, McCord SE, Schallner JW, Treminio RS, Wheeler BE, Stauffer NG, Spiegal S, Duniway MC, Traynor ACE, Kachergis E, Houdeshell C-A (2024) Establishing quantitative benchmarks for soil erosion and ecological monitoring, assessment, and management. Ecological Indicators 159, 111661.
| Crossref | Google Scholar |
Williams JC, Pierson FB, Spaeth KE, Brown JR, Al-Hamdan OZ, Weltz MA, Nearing MA, Herrick JE, Boll J, Robichaud PR, Goodrich DC, Heilman P, Guertin DP, Hernandez M, Wei H, Hardegree SP, Strand EK, Bates JD, Metz LJ, Nichols MH (2016) Incorporating hydrologic data and ecohydrologic relationships into ecological site descriptions. Rangeland Ecology & Management 69, 4-19.
| Crossref | Google Scholar |
Yang Y, Fang J, Ji C, Ma W, Mohammat A, Wang S, Wang S, Datta A, Robinson D, Smith P (2012) Widespread decreases in topsoil inorganic carbon stocks across China’s grasslands during 1980s–2000s. Global Change Biology 18, 3672-3680.
| Crossref | Google Scholar |