The role of gene editing, organoids, and in vitro phenotyping in sustainable animal production
Bethany K. Redel
A
B
C
Abstract
There is a critical need for improving animal resilience, welfare, and productivity to meet the nutritional needs of the growing global population. While selective breeding has brought about tremendous improvement in livestock genetics and improving traits, it is a relatively lengthy process to integrate beneficial alleles into the herd and it is not possible to introduce variants identified in other species. Therefore, gene editing provides researchers with a tool to rapidly overcome many of these challenges. This review highlights the advances in gene editing technology, the methods used to generate gene edited livestock, and approaches that can be used to accelerate the discovery of novel alleles linked to specific traits in vitro. Additionally, the application of organoid technology is discussed, and how that linked with gene editing technology can mimic the in vivo physiology and biological functions in vitro, providing answers to important biological questions and decreasing the number of large animals needed for research. Together, these tools will enable production agriculture to be more productive and thus better able to meet the growing worldwide demand for food.
Keywords: CRISPR-Cas, direct embryo delivery, gene editing, high-throughput phenotyping, host-pathogen interactions, livestock, organoids, somatic cell nuclear transfer.
Introduction
It is estimated that the global population will reach 10 billion people by 2050. Increasing animal productivity and resilience is vital to meet the nutritional needs of the growing global population. However, there are many challenges facing livestock production that can create stress for both producers and animals, causing production to fall behind, and ultimately resulting in high prices for consumers with the potential to cause food insecurity. Globally, 309 million people are facing acute levels of food insecurity (World Food Programme 2024), and animal disease is one of the largest contributors to the loss of livestock. The World Organisation for Animal Health estimates that 20% of livestock production is lost due to disease every year (World Organisation for Animal Health 2024).
Our ability to produce genetically engineered cells and animals has dramatically improved with the recent advances in gene editing technology. This technology has the ability to provide a solution to many issues facing production agriculture today. Genetic engineering can influence livestock production by generating novel alleles linked to beneficial traits and decreasing the time needed to introduce the novel allele(s) into the herd, with the overall goal of improving animal health and resilience. The possibilities of this technology and its impact on livestock are endless. Recently, gene-edited pigs were generated that were resistant to the porcine reproductive and respiratory syndrome virus (PRRSV), which is the most economically devastating disease in the swine industry (Whitworth et al. 2016). In addition to these pigs, the first gene-edited calf was recently produced with resistance to the bovine viral diarrhea virus (BVDV), one of the most significant viruses affecting cattle world-wide (Workman et al. 2023). These examples illustrate gene editing technology’s profound impact on generating more resilient and healthier livestock.
In this review, we discuss the recent advances in gene editing systems and their impact on genetic engineering in livestock species. We also detail the importance of in vitro phenotyping to increase the possibility of identifying gene(s) or causative mutations that may influence specific livestock traits.
Genetic improvements using gene editing technologies
Traditionally, improvement in livestock genetics has been accomplished using a selective breeding approach. This method has been incredibly successful in improving traits in livestock species; however, it is limited in the ability to introduce novel alleles from other species and takes considerable time to breed specific traits into a herd. With the development and application of new gene editing tools, the amount of time it takes to produce genetically modified livestock has decreased significantly. Over the past 20 years, gene editing nucleases have been developed and have been reviewed in detail by many (Sander and Joung 2014; Redel and Prather 2016; Ryu et al. 2018; Yang and Wu 2018; Lee et al. 2019, 2020; Perisse et al. 2021; Whitworth et al. 2022). The foundation of gene editing technology is the use of site-directed endonucleases that cleave specific DNA sequences and create double-strand breaks (DSBs) in the cell genome. The cell’s own repair mechanisms can then repair these DSBs until errors are made using the error prone non-homologous end joining (NHEJ) repair process. The errors produced by NHEJ are often small insertions or deletions (indels), creating a frameshift mutation and premature stop codon for gene disruption experiments where a knockout of the gene is desired. In the presence of a donor template, homology directed repair (HDR) can also be used to create a knock-in to incorporate a specific DNA sequence into the genome.
The first endonucleases developed to introduce site-directed modifications were zinc finger nucleases (ZFNs) and transcription activator-like effector nucleases (TALENs). These endonucleases improved the efficiency of producing genetically engineered cells and livestock compared to traditional methods (Whyte et al. 2011; Carlson et al. 2012). While these endonucleases advanced the efficiency of genetically engineered (GE) livestock production, it was the repurposing of the clustered regularly interspaced short palindromic repeats (CRISPR)–CRISPR associated protein 9 (Cas9) system that transformed the GE animal production process. The CRISPR–Cas9 system was adapted from Streptococcus pyogenes, where it is used as the adaptive immune system in prokaryotes (Wiedenheft et al. 2012; Rodríguez-Rodríguez et al. 2019). This system cleaves viral or plasmid invader DNA and stops the infection (Garneau et al. 2010; Marraffini 2016). Because of its efficient mechanism to cleave target regions of DNA, this system was adapted to be used to generate genetic modifications in mammalian cells (Cong et al. 2013). Three specific components are needed for the CRISPR system to work: a transactivating CRISPR RNA (tracrRNA), Cas9, and a precursor CRISPR RNA (crRNA) (Brazelton et al. 2015). Researchers combined the tracrRNA and crRNA to simplify this gene editing system and together they create a single guide RNA (sgRNA) (Jinek et al. 2012). The sgRNA, homologous to the target region in the genome, guides the Cas9 endonuclease which will then cleave the DNA if a protospacer adjacent motif (PAM) sequence is present (Cong et al. 2013).
Once cleaved, DSBs in the DNA can then be repaired as mentioned above. The most efficient mechanism for repair is NHEJ. It is thought that the cell will correctly repair the DSB each time the CRISPR–Cas9 cuts the repaired DNA until a mistake is made in the nucleotide sequence and the guide no longer recognizes the targeted region. The most frequent mutations are indels or large deletions depending on sgRNA design and number of guides used. This is a very effective method for producing knockout alleles with little issue. However, creating a knock-in of a specific DNA template into the genome using HDR often results in very low efficiency of insertions and high rate of indels (Mao et al. 2008). More recently, DNA base editors and prime editors have been developed to circumvent this limitation. Base and prime editors rely on the use of modified enzymes to directly create point mutations into DNA or RNA without creating DSBs (Komor et al. 2016, 2017; Gaudelli et al. 2017). Base editors do have constraints on what nucleotide mutations can be made, but with the use of prime editors insertions, deletions, and all 12 possible base-to-base conversions, without requiring DSBs or donor DNA templates, are possible (Anzalone et al. 2019). Prime editing has been successful in producing edited human cells, mice, and zebrafish (Anzalone et al. 2019; Liu et al. 2020a; Petri et al. 2022). More recently, researchers used prime editors to generate pig gene-edited blastocysts and sheep (Zhou et al. 2023).
Methods for GE livestock production
The production of GE livestock (pigs, cows, sheep, and goats) can be accomplished using multiple approaches. Prior to the use of endonucleases for genetic modifications, genetic modification of somatic cells followed by somatic cell nuclear transfer, the cloning procedure, was the primary method used to create GE livestock beyond random integration of transgenes. However, with the development of gene editing systems, direct injection into oocytes or zygotes is possible, providing high editing efficiencies. Here we will summarize the most popular methods for GE livestock production.
Direct delivery into oocytes or zygotes
The first reported transgenic animal was produced by injection of genes into the pronucleus of mouse zygotes followed by transfer into recipient mice (Gordon and Ruddle 1981). Soon after, more transgenic mice, along with rabbits, cattle, pigs, and sheep were produced using zygote microinjections (Palmiter et al. 1982; Hammer et al. 1985; Hill et al. 1992; Wall 1996). This process is technically challenging, very costly, results in very low integration efficiencies, and researchers are limited to the addition of DNA into the genome by random integration (Wall 1996). There is no way to control the number of copies or locations in which the foreign DNA is integrated into the genome, causing an inability to control expression or mosaicism within founder individuals. Due to these limitations, many animals are required to be generated to be able to screen for the optimal integration number and expression of the transgene.
With developments in gene editing technologies (ZFNs, TALENs, and CRISPR), the efficiency of producing targeted modifications using the microinjection approach has been rapidly improved. Mature oocytes or freshly fertilized zygotes can be injected with the CRISPR–Cas9 system to create either indels to produce frameshift mutations or with a donor template to create DNA knock-in modifications. This microinjection approach has produced animals that contain high editing efficiencies (Bevacqua et al. 2016; Sheets et al. 2016; Li et al. 2017; Whitworth et al. 2017; Carey et al. 2019; Lamas-Toranzo et al. 2019; Owen et al. 2021).
Direct injection into the cytoplasm of oocytes or embryos requires specialized training and expensive equipment. An alternative to the direct zygote delivery method is electroporation. Electroporation is commonly used to deliver gene editing reagents into somatic cells. This method has been optimized to be used for delivery of reagents into zygotes for many livestock species (Tanihara et al. 2016; Hirata et al. 2019; Camargo et al. 2020).
Mosaicism and unknown off-targeting events are limitations often encountered with the direct delivery of gene editing reagents into oocytes or zygotes. Many groups are working on identifying methods to identify and mitigate both challenges as direct delivery is often advantageous over more technical methods discussed below (Lamas-Toranzo et al. 2019; Redel et al. 2024).
Somatic cell nuclear transfer
The somatic cell nuclear transfer (SCNT) procedure, also referred to as cloning, is a method in which a donor cell is used to transfer specific genomic material into an enucleated oocyte (Campbell et al. 1996). This SCNT procedure has traditionally been the most widely used procedure to produce genetically engineered animals as somatic cells are modified and intensely screened to identify the exact genetic modification prior to making an animal (Polejaeva 2021). Knowing the exact genetic modification of the future offspring is an advantage if these animals will be used as founder animals. However, the SCNT procedure involves many technically challenging steps that require highly specialized training and much time. Another disadvantage to this technology is that the resulting animals may contain abnormalities due to aberrant DNA remodeling/reprogramming, causing them to have issues after birth or even loss of life (Whitworth and Prather 2010). Some of the aberrant remodeling is a result of changes in DNA methylation and imprinting. In general, it is thought that since the imprint pattern is reset during gametogenesis, if the cloned animal can reach sexual maturity and reproduce, then the offspring will be normal and not have DNA methylation mistakes. While SCNT is not the easiest method to produce gene-edited animals, it is still being used today, with continued research on improving efficiency of animal production (Lee and Prather 2013; Whitworth et al. 2016; Polejaeva 2021; Workman et al. 2023).
Challenges accompanying the genome editing systems
Producing GE livestock is much more costly and time consuming than producing GE mice. Large livestock species have long gestational intervals and have few offspring, making the production of GE offspring more challenging. Because of the long gestation, small offspring number, and time to reach puberty in founder animals, it is imperative that the founder animals generated have the genotype that is needed for phenotyping. However, direct injection of the CRISPR system into the oocyte or zygote can generate offspring that may not be useful for phenotyping. As mentioned above, these founder animals generated from direct injection can contain unedited alleles, carry edits that are not useful (in-frame deletions), or possess mosaicism within an individual animal, making phenotyping complicated (Hennig et al. 2020). Single guide RNAs that do not cut DNA prior to the first cleavage event have the ability to produce a mosaic animal containing more than one genotype across tissues within an individual animal (Vilarino et al. 2018). This can cause some animals to not be used for phenotyping or the need to obtain offspring from these animals to be able to study the expected phenotype, thus prolonging research findings >2–3 years. Unlike methods used to produce cloned embryos where the exact genetic modification is screened and selected for, direct injection results in edits that are difficult to predict. While edits can be screened by embryo biopsy (although even this may not be accurate as it will be dependent on the random sample of cells removed from a potentially mosaic embryo), this process is not feasible for litter-bearing animals, like pigs, where many embryos are transferred into one surrogate. Test injections to identify the highest cutting guides are essential in producing animals that will be useful for phenotyping founder animals. If selecting high efficiency guides is not possible due to the need to target a very particular region of the genome, producing F1 animals for phenotyping is warranted to correctly phenotype.
The use of gene editing technology allows for production of GE livestock at a high efficiency; however, assessing genome integrity after editing has not been carefully examined. Because these technologies often rely on inducing a DSB in the genome, there is also a chance unintended DSBs may be introduced elsewhere in the genome. These unintended mutations, often referred to as ‘off-site cleavage events’ have been considered a concern of utilizing genome editing systems. While off-target events are most likely silent, there are concerns that these events may lead to unexpected phenotypes, thus negatively impacting animal production or the phenotype. A second potential unintended event is the incorporation of a portion of the gene editing material into the genome. Researchers produced hornless dairy cattle by editing using TALENs to introduce the POLLED allele (Carlson et al. 2016). The resulting gene edited cattle do not need to be dehorned, a procedure that protects the animals and producers from injury, but horn removal causes the animal pain. Incorporating this allele into the dairy industry could improve the welfare of cattle by eliminating the need for them to undergo this painful procedure. Propagation of the POLLED allele has great potential for improving dairy cattle welfare. The resulting GE animals were analyzed for off-targeting events, and none were found initially. However, later it became clear that the edited calves contained the full length plasmid backbone integrated into the target cut site (Norris et al. 2020). Currently, many gene-edited livestock have been produced and off-target analysis is routine in animals that may be incorporated into the food chain or used as models for human diseases (Burger et al. 2024; Redel et al. 2024). Identifying ways to mitigate the creation of unwanted modifications using the gene editing system will likely be important for the regulatory process and consumer acceptance.
Use of high-throughput in vitro phenotyping technology
Due to the challenges associated with producing animals with gene editing technologies, i.e. length of gestation, production of unusable alleles, mosaicism, and potential off-targeting, new methods need to be developed to accelerate findings that link the genome and the phenome. Research needs to move towards making only the animals that will have the desired phenotype. Researchers are utilizing in vitro systems paired with genome editing technology to enhance the ability to make these scientific discoveries. To move to in vitro screening, a full understanding of what is needed to recapitulate the in vivo system in an in vitro setting is critical. Identifying the appropriate biological cell type that expresses the gene(s) and is the correct cell type for phenotyping the trait is an essential starting point. For example, porcine epidemic diarrhea virus does not replicate well in most porcine immortalized cell lines and in vitro challenges are most often performed in Vero cells, an African green monkey kidney line that is not capable of producing a type I interferon response (Li et al. 2019).
Selecting the optimal cell type for genetic engineering and for downstream phenotyping is important. Primary cell cultures contain the in vivo tissue characteristics initially; however, they are limited in their ability to self-renew and as they age, they have the potential for changes in their function and morphology. At low passage, they contain the morphological and functional characteristics of the tissue from which they are derived, but as passage number or time in culture increases, there is a decrease in cellular integrity and an increase in senesce at early passages. This makes gene editing a challenge in these cells when passaging and extended time in culture is needed. Livestock immortalized cell lines are available and have been used to phenotype and study many different cellular mechanisms and biological aspects in vitro (Kasloff and Weingartl 2016; Fay et al. 2020; Guo et al. 2022). Immortalized cell lines have been manipulated so that they may maintain the ability to proliferate indefinitely (Carter and Shieh 2010). This allows for increased passaging and culture for long periods of time without senescing. However, there are often issues with aneuploidy of these cell lines which can have a direct impact on measuring gene expression and phenotype of gene edited cells (Sheltzer et al. 2012; Johnsson et al. 2024). Genome wide loss-of-function CRISPR library screens can target thousands of genes within a species’ genome and examine whether they are linked to a specific biological event. These screens can also be used to examine which genes play a role in pathogen (parasite, bacterial, or viral) infection. Cells that overcome these infections can then be analyzed to identify potential receptors or genes that play a role in pathogen resilience (Sidik et al. 2016; Miles et al. 2017). CRISPR screens are most often completed in immortalized cell lines due to their ability to continuously replicate and support pathogen infectivity. However, if these cells contain a diploid or triploid genome, this can drastically reduce the gene editing efficiency and complicate the downstream phenotyping.
A major advancement in cell culture methods is the development of three-dimensional (3D) systems that may closely resemble the in vivo organ from which it originated. Classical two-dimensional (2D) or monolayer culture systems may not be the optimal culture system if the 3D systems can maintain the organ-like characteristics over extended periods of time and passage number. Identifying methods to isolate, culture, and differentiate stem cells has allowed researchers the ability to generate self-renewing structures that replicate the in vivo tissue to better model whole animal phenotypes and answer biological questions.
Organoids
The use of organoids to understand in vivo mechanisms for disease and biology has increased in recent years due to their unique ability to mimic whole organ structures during in vitro culture. Organoids are 3D ‘mini-organs’ that can self-organize, self-renew, and mimic the structure and function of the organ from which they were derived. In mice and humans, they can be differentiated from embryonic stem cells (ESCs) or induced pluripotent stem cells (iPSCs), propagated from tumor cells, or grown from tissue biopsy. However, in livestock, there are limited reproducible methods for establishment of ESC and iPSCs. Therefore, in livestock species, organoids are primarily derived from organ biopsies. The type of cell population used to start the culture is vital for the success of the development of organoids as they can affect the structure and ability to mimic the physiology of the organ. Isolating cells for culture is similar across origin types with dissociation being the main method. Tissue-derived stem cells are collected through optimized tissue collection and dissociation of cells. Cells are then embedded into a 3D matrix to mimic their original environment (Zhao et al. 2022).
Matrigel has been a key component in the establishment of many organoid cultures. Developed in 1977 from mouse sarcoma tumors, Matrigel contains laminin, collagen, entactin, and growth factors that are similar in composition to the basement membrane and support the in vitro culture of cells (Orkin et al. 1977). When stem cells are embedded in Matrigel and exposed to tissue-specific growth factors they can organize into organoids. However, the variability in Matrigel, as a biologically derived matrix, is not desirable. Other matrixes can support organoid growth like natural ECM or synthetic hydrogels (Zhao et al. 2022).
Researchers first recorded the successful culture and maintenance of organoids when small clumps of cells were able to self-organize from mouse ESCs into neural cells (Eiraku et al. 2008). Then in 2009, researchers identified ways to improve the culture system for intestinal epithelium (Sato et al. 2009). In vivo, the intestinal epithelium is consistently renewed every 3–5 days; however, the intestinal epithelium culture systems did not allow long-term survival of these cells. Using previously described insights into the growth requirements of the intestinal epithelium, researchers were able to design a culture system to promote proliferation, expansion of the intestinal crypts, and to mimic the normal tissue (Korinek et al. 1998; Pinto et al. 2003; Kuhnert et al. 2004). The addition of a Wnt agonist (R-spondin 1), epidermal growth factors, and Noggin to the growth medium and culture in Matrigel allows for the maintenance of stem cells in the isolated intestinal crypts. Their attempts and passages eventually resulted in the formation of organoids with more than 40 crypt domains, which surrounded a lumen and were lined by villus-like epithelium. These organoids were cultured and passaged for more than 8 months without losing their intestinal characteristics (Sato et al. 2009).
While there are many studies involving mice and humans, the use of farm species as a comparative model is rising in popularity. Different types of organoids, ranging from digestive to reproductive, have been developed across many livestock species. These can be used in species-specific and cross-species studies (Table 1). Intestinal organoids have been used to investigate the interactions between various nutrients on pig and chicken intestinal epithelium development and stability (Ferrandis Vila et al. 2018; Zhu et al. 2019; Kawasaki et al. 2022; Mitchell et al. 2024). Similarly, sheep pancreatic duct organoids have been used to explore the impacts of copper on organ growth (Liu et al. 2020b). Organoids provide a tool to ask many basic biological questions. High-throughput studies can be accomplished using organoids paired with gene editing technology to provide phenotyping data, thus reducing the costs and need for large animal numbers (Giuffra et al. 2019) (Fig. 1).
Species | Tissue | References | |
---|---|---|---|
Pig | Esophageal submucosal gland | von Furstenberg et al. (2017) | |
Airway | Jiang et al. (2022) | ||
Intestine | Sharbati et al. (2015), Gonzalez et al. (2013), van der Hee et al. (2018), Khalil et al. (2016) | ||
Rectum | Adegbola et al. (2017) | ||
Gallbladder | Zarei et al. (2020) | ||
Testis | Sakib et al. (2019) | ||
Lung | Yang et al. (2024) | ||
Kidney | Li et al. (2024) | ||
Neuro | Hwang et al. (2021) | ||
Cattle | Airway | Jiang et al. (2022) | |
Intestine | Powell and Behnke (2017) | ||
Mammary gland | Martignani et al. (2018) | ||
Airway | Quah et al. (2023) | ||
Oviduct | Bourdon et al. (2021) | ||
Sheep | Intestine | Powell and Behnke (2017) | |
Pancreatic duct | Liu et al. (2020b) | ||
Chicken | Intestine | Li et al. (2018) | |
Fish | Rectum | Schwarz et al. (2015) | |
Retinal | Zilova et al. (2021) |
Summary of methods for identifying genes influencing traits in livestock species. Organoids play a role in gene editing and phenotyping important traits in vitro to validate whether the genetic modification has an impact on the trait before making a gene-edited animal. This system can decrease the need for large numbers of animals in research and provide a means to accelerate the time needed for discovering gene(s) important for improving production traits. Once modified genes are validated to have the expected outcome in vitro, multiple methods are available to produce the gene-edited animal.
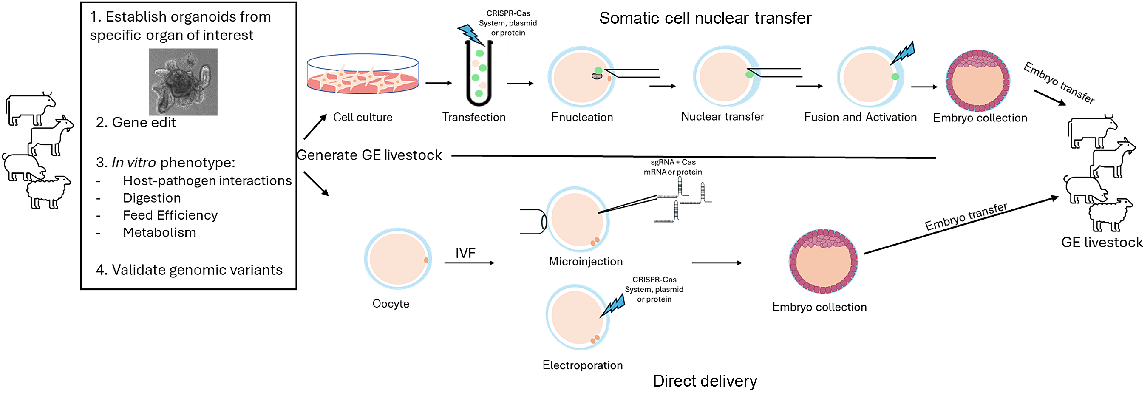
Intestinal organoids: investigation of host–pathogen interactions
Intestinal organoids have been used to study feed efficiency, nutrient uptake and metabolism, and host–pathogen interactions in livestock species (Koltes and Gabler 2016; Pierzchalska et al. 2017; Derricott et al. 2019; Madsen et al. 2024) (Fig. 2). However, modeling host–pathogen interactions using intestinal organoids is becoming a critical research topic to understand pathogenesis and strategies for mitigating many diseases facing animals today. Traditional cell lines used to model infectivity in vitro do not mimic the normal in vivo environment or do not always support viral infection (Zhang et al. 2018).
Images of intestinal organoids derived from the small intestine of a newborn piglet over passages 1 and 2. Porcine isolated crypts were embedded in Matrigel and cultured with IntestiCult Organoid Growth Medium (Stem Cell Technologies). By day 4, organoids containing lumen and crypts formed from all three segments of the porcine small intestine and were able to be maintained beyond passage number 6.
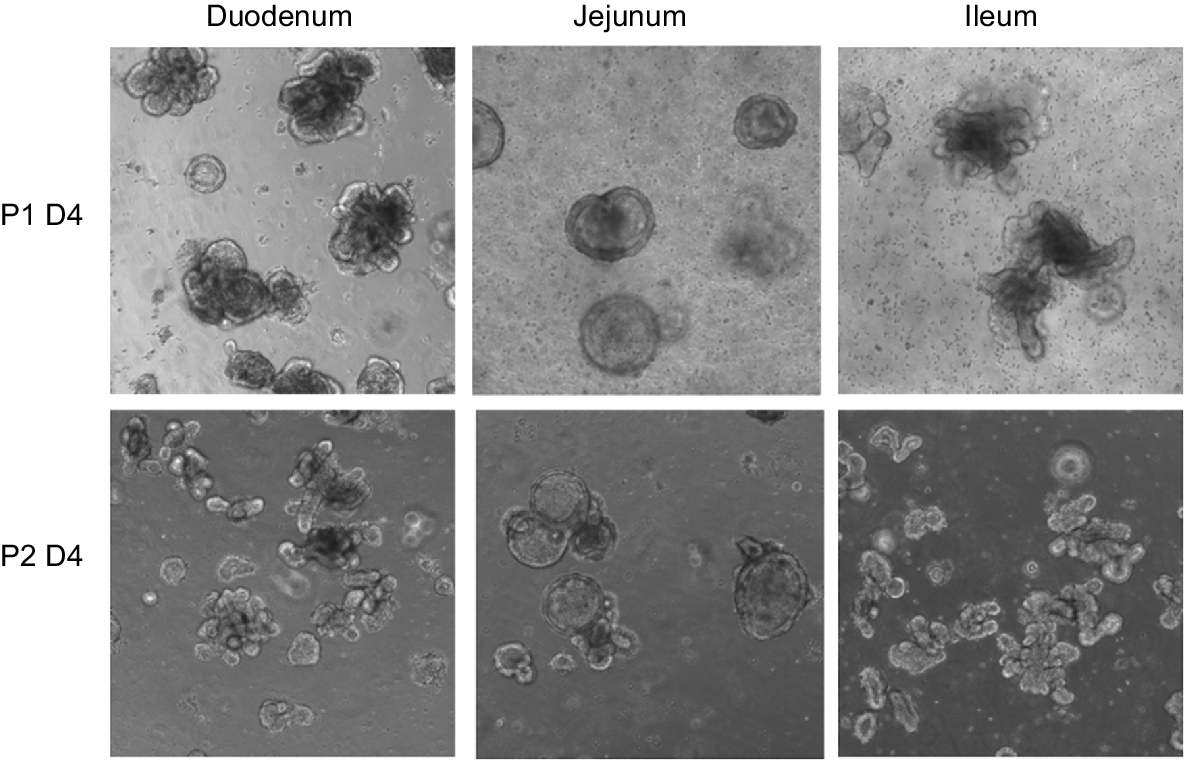
There are many coronaviruses that infect livestock and create significant losses. One, the porcine epidemic diarrhea virus (PEDV), is a devastating virus to the swine industry. This virus causes high mortality rates in piglets less than 2 weeks of age, with symptoms of vomiting, anorexia, diarrhea, dehydration, and weight loss (Fu et al. 2017). In 2013, this deadly virus made its way to North America and caused the death of more than 7 million piglets, roughly 10% of the country’s pig population in 1 year (Diel et al. 2016). Vaccines are not effective against current strains; therefore, identifying alternative strategies to mitigate this virus is important. Porcine epidemic diarrhea virus infects the villous epithelium of the small intestine but identifying an appropriate biological system in vitro has remained a challenge. Recently, researchers illustrated that swine intestinal organoids are susceptible to PEDV infection and were able to recapitulate the events seen with infectivity in vivo (Li et al. 2019). However, the delivery of the virus to the apical side of the lumen, where the virus would naturally infect, was accomplished by creating a 2D monolayer from these organoids to demonstrate infectivity. This identified a challenge with modeling host–pathogen interactions in organoids: the epithelium has polarity, with the basal epithelium formed toward the outside of the organoid, making the apical membrane hard to access. Researchers recently identified an ‘apical-out’ organoid system that was maintained in a suspension culture (Li et al. 2020). These apical-out organoids maintained the characteristics of traditional basal-out organoids and were able to be infected by transmissible gastroenteritis virus (TGEV), another enteric swine coronavirus.
The use of livestock models is not limited to improving production. They can also be used to investigate pathogen interactions and ultimately impact both animal and human health. With the recent pandemic, the public is as aware as ever of the spread of pathogens between animals and humans. Organoids can be used to evaluate how different species contract the same pathogens. Using animal organoids can also be beneficial as they might be a more reproducible system for high-value projects like clinical studies for drug development compared to monolayer systems. This, paired with the organoid’s ability to culture for a long period of time, can reduce the need for many live animal research models and the care and challenges that are associated with them.
Conclusions and future prospectives
Gene editing has transformed our ability to investigate whether genome variants are causative for many different livestock traits. The identification of novel alleles linked to specific traits using high-throughput gene editing and phenotyping in vitro is becoming increasingly important. Future research priorities should focus on bridging the gap between genomic variation and traits of interest to allow for enhanced discovery of gene influencing traits, especially in less characterized species. Recent advancements in cell culture systems and the development of organoids will have a positive impact on determining genes that play a critical role in pathogen infectivity, metabolism, feed efficiency, and digestion, along with many others. These in vitro systems will decrease the requirement for live animals to be tested and, at the same time, increase the throughput of discovery. With technology continuously improving and advancing, livestock production will be able to overcome many of the challenges it is currently facing and ensure that we are able to maintain a sustainable food supply with healthy and resilient animals.
Data availability
The establishment of porcine organoids in this paper will be shared upon reasonable request to the corresponding author. Otherwise no new data were generated or analyzed in this review.
Declaration of funding
The funding for the establishment of porcine organoids presented here was provided by USDA-ARS project number 5070-31320-001-00D.
References
Adegbola S, Moore J, Sahnan K, Tozer P, Phillips R, Warusavitarne J, Faiz O, Hart A (2017) P005 Establishing a porcine model to translate anorectal stem cell organoid models to elucidate the aetiology of perianal Crohn’s fistulae. Journal of Crohn’s and Colitis 11, S81-S82.
| Crossref | Google Scholar |
Anzalone AV, Randolph PB, Davis JR, Sousa AA, Koblan LW, Levy JM, Chen PJ, Wilson C, Newby GA, Raguram A, Liu DR (2019) Search-and-replace genome editing without double-strand breaks or donor DNA. Nature 576, 149-157.
| Crossref | Google Scholar | PubMed |
Bevacqua RJ, Fernandez-Martín R, Savy V, Canel NG, Gismondi MI, Kues WA, Carlson DF, Fahrenkrug SC, Niemann H, Taboga OA, Ferraris S, Salamone DF (2016) Efficient edition of the bovine PRNP prion gene in somatic cells and IVF embryos using the CRISPR/Cas9 system. Theriogenology 86, 1886-1896.e1.
| Crossref | Google Scholar |
Bourdon G, Cadoret V, Charpigny G, Couturier-Tarrade A, Dalbies-Tran R, Flores M-J, Froment P, Raliou M, Reynaud K, Saint-Dizier M, Jouneau A (2021) Progress and challenges in developing organoids in farm animal species for the study of reproduction and their applications to reproductive biotechnologies. Veterinary Research 52, 42.
| Crossref | Google Scholar | PubMed |
Brazelton VA, Jr, Zarecor S, Wright DA, Wang Y, Liu J, Chen K, Yang B, Lawrence-Dill CJ (2015) A quick guide to CRISPR sgRNA design tools. GM Crops & Food 6, 266-276.
| Crossref | Google Scholar | PubMed |
Burger BT, Beaton BP, Campbell MA, Brett BT, Rohrer MS, Plummer S, Barnes D, Jiang K, Naswa S, Lange J, Ott A, Alger E, Rincon G, Rounsley S, Betthauser J, Mtango NR, Benne JA, Hammerand J, Durfee CJ, Rotolo ML, Cameron P, Lied AM, Irby MJ, Nyer DB, Fuller CK, Gradia S, Kanner SB, Park K-E, Waters J, Simpson S, Telugu BP, Salgado BC, Brandariz-Nuñez A, Rowland RRR, Culbertson M, Rice E, Cigan AM (2024) Generation of a commercial-scale founder population of porcine reproductive and respiratory syndrome virus resistant pigs using CRISPR-Cas. The CRISPR Journal 7, 12-28.
| Crossref | Google Scholar | PubMed |
Camargo LSA, Owen JR, Van Eenennaam AL, Ross PJ (2020) Efficient one-step knockout by electroporation of ribonucleoproteins into zona-intact bovine embryos. Frontiers in Genetics 11, 570069.
| Crossref | Google Scholar |
Campbell KHS, McWhir J, Ritchie WA, Wilmut I (1996) Sheep cloned by nuclear transfer from a cultured cell line. Nature 380, 64-66.
| Crossref | Google Scholar | PubMed |
Carey K, Ryu J, Uh K, Lengi AJ, Clark-Deener S, Corl BA, Lee K (2019) Frequency of off-targeting in genome edited pigs produced via direct injection of the CRISPR/Cas9 system into developing embryos. BMC Biotechnology 19, 25.
| Crossref | Google Scholar | PubMed |
Carlson DF, Tan W, Lillico SG, Stverakova D, Proudfoot C, Christian M, Voytas DF, Long CR, Whitelaw CBA, Fahrenkrug SC (2012) Efficient TALEN-mediated gene knockout in livestock. Proceedings of the National Academy of Sciences of the United States of America 109, 17382-17387.
| Crossref | Google Scholar | PubMed |
Carlson DF, Lancto CA, Zang B, Kim E-S, Walton M, Oldeschulte D, Seabury C, Sonstegard TS, Fahrenkrug SC (2016) Production of hornless dairy cattle from genome-edited cell lines. Nature Biotechnology 34, 479-481.
| Crossref | Google Scholar | PubMed |
Cong L, Ran FA, Cox D, Lin S, Barretto R, Habib N, Hsu PD, Wu X, Jiang W, Marraffini LA, Zhang F (2013) Multiplex genome engineering using CRISPR/Cas systems. Science 339, 819-823.
| Crossref | Google Scholar | PubMed |
Derricott H, Luu L, Fong WY, Hartley CS, Johnston LJ, Armstrong SD, Randle N, Duckworth CA, Campbell BJ, Wastling JM, Coombes JL (2019) Developing a 3D intestinal epithelium model for livestock species. Cell and Tissue Research 375, 409-424.
| Crossref | Google Scholar | PubMed |
Diel DG, Lawson S, Okda F, Singrey A, Clement T, Fernandes MHV, Christopher-Hennings J, Nelson EA (2016) Porcine epidemic diarrhea virus: an overview of current virological and serological diagnostic methods. Virus Research 226, 60-70.
| Crossref | Google Scholar | PubMed |
Eiraku M, Watanabe K, Matsuo-Takasaki M, Kawada M, Yonemura S, Matsumura M, Wataya T, Nishiyama A, Muguruma K, Sasai Y (2008) Self-organized formation of polarized cortical tissues from ESCs and its active manipulation by extrinsic signals. Cell Stem Cell 3, 519-532.
| Crossref | Google Scholar | PubMed |
Fay PC, Cook CG, Wijesiriwardana N, Tore G, Comtet L, Carpentier A, Shih B, Freimanis G, Haga IR, Beard PM (2020) Madin-Darby bovine kidney (MDBK) cells are a suitable cell line for the propagation and study of the bovine poxvirus lumpy skin disease virus. Journal of Virological Methods 285, 113943.
| Crossref | Google Scholar | PubMed |
Ferrandis Vila M, Trudeau MP, Hung Y-T, Zeng Z, Urriola PE, Shurson GC, Saqui-Salces M (2018) Dietary fiber sources and non-starch polysaccharide-degrading enzymes modify mucin expression and the immune profile of the swine ileum. PLoS ONE 13, e0207196.
| Crossref | Google Scholar | PubMed |
Fu F, Li L, Shan L, Yang B, Shi H, Zhang J, Wang H, Feng L, Liu P (2017) A spike-specific whole-porcine antibody isolated from a porcine B cell that neutralizes both genogroup 1 and 2 PEDV strains. Veterinary Microbiology 205, 99-105.
| Crossref | Google Scholar | PubMed |
Garneau JE, Dupuis M-E, Villion M, Romero DA, Barrangou R, Boyaval P, Fremaux C, Horvath P, Magadán AH, Moineau S (2010) The CRISPR/Cas bacterial immune system cleaves bacteriophage and plasmid DNA. Nature 468, 67-71.
| Crossref | Google Scholar | PubMed |
Gaudelli NM, Komor AC, Rees HA, Packer MS, Badran AH, Bryson DI, Liu DR (2017) Programmable base editing of A•T to G•C in genomic DNA without DNA cleavage. Nature 551, 464-471.
| Crossref | Google Scholar | PubMed |
Giuffra E, Tuggle CK, FAANG Consortium (2019) Functional annotation of animal genomes (FAANG): current achievements and roadmap. Annual Review of Animal Biosciences 7, 65-88.
| Crossref | Google Scholar | PubMed |
Gonzalez LM, Williamson I, Piedrahita JA, Blikslager AT, Magness ST (2013) Cell lineage identification and stem cell culture in a porcine model for the study of intestinal epithelial regeneration. PLoS ONE 8, e66465.
| Crossref | Google Scholar | PubMed |
Gordon JW, Ruddle FH (1981) Integration and stable germ line transmission of genes injected into mouse pronuclei. Science 214, 1244-1246.
| Crossref | Google Scholar | PubMed |
Guo D, Zhang L, Wang X, Zheng J, Lin S (2022) Establishment methods and research progress of livestock and poultry immortalized cell lines: a review. Frontiers in Veterinary Science 9, 956357.
| Crossref | Google Scholar | PubMed |
Hammer RE, Pursel VG, Rexroad CE, Jr, Wall RJ, Bolt DJ, Ebert KM, Palmiter RD, Brinster RL (1985) Production of transgenic rabbits, sheep and pigs by microinjection. Nature 315, 680-683.
| Crossref | Google Scholar | PubMed |
Hennig SL, Owen JR, Lin JC, Young AE, Ross PJ, Van Eenennaam AL, Murray JD (2020) Evaluation of mutation rates, mosaicism and off target mutations when injecting Cas9 mRNA or protein for genome editing of bovine embryos. Scientific Reports 10, 22309.
| Crossref | Google Scholar | PubMed |
Hill KG, Curry J, DeMayo FJ, Jones-Diller K, Slapak JR, Bondioli KR (1992) Production of transgenic cattle by pronuclear injection. Theriogenology 37, 222.
| Crossref | Google Scholar |
Hirata M, Tanihara F, Wittayarat M, Hirano T, Nguyen NT, Le QA, Namula Z, Nii M, Otoi T (2019) Genome mutation after introduction of the gene editing by electroporation of Cas9 protein (GEEP) system in matured oocytes and putative zygotes. In Vitro Cellular & Developmental Biology – Animal 55, 237-242.
| Crossref | Google Scholar | PubMed |
Hwang S-U, Eun K, Kim M, Yoon JD, Cai L, Choi H, Oh D, Lee G, Kim H, Kim E, Hyun S-H (2021) Establishment of 3D neuro-organoids derived from pig embryonic stem-like cells. International Journal of Molecular Sciences 22, 2600.
| Crossref | Google Scholar | PubMed |
Jiang C, Li L, Xue M, Zhao L, Liu X, Wang W, Feng L, Liu P (2022) Long-term expanding porcine airway organoids provide insights into the pathogenesis and innate immunity of porcine respiratory coronavirus infection. Journal of Virology 96, e0073822.
| Crossref | Google Scholar | PubMed |
Jinek M, Chylinski K, Fonfara I, Hauer M, Doudna JA, Charpentier E (2012) A programmable dual-RNA-guided DNA endonuclease in adaptive bacterial immunity. Science 337, 816-821.
| Crossref | Google Scholar | PubMed |
Johnsson M, Hickey JM, Jungnickel MK (2024) Building in vitro tools for livestock genomics: chromosomal variation within the PK15 cell line. BMC Genomics 25, 49.
| Crossref | Google Scholar | PubMed |
Kasloff SB, Weingartl HM (2016) Swine alveolar macrophage cell model allows optimal replication of influenza A viruses regardless of their origin. Virology 490, 91-98.
| Crossref | Google Scholar | PubMed |
Kawasaki M, Goyama T, Tachibana Y, Nagao I, Ambrosini YM (2022) Farm and companion animal organoid models in translational research: a powerful tool to bridge the gap between mice and humans. Frontiers in Medical Technology 4, 895379.
| Crossref | Google Scholar | PubMed |
Khalil HA, Lei NY, Brinkley G, Scott A, Wang J, Kar UK, Jabaji ZB, Lewis M, Martín MG, Dunn JCY, Stelzner MG (2016) A novel culture system for adult porcine intestinal crypts. Cell and Tissue Research 365, 123-134.
| Crossref | Google Scholar | PubMed |
Koltes DA, Gabler NK (2016) Characterization of porcine intestinal enteroid cultures under a lipopolysaccharide challenge. Journal of Animal Science 94, 335-339.
| Crossref | Google Scholar |
Komor AC, Kim YB, Packer MS, Zuris JA, Liu DR (2016) Programmable editing of a target base in genomic DNA without double-stranded DNA cleavage. Nature 533, 420-424.
| Crossref | Google Scholar | PubMed |
Komor AC, Zhao KT, Packer MS, Gaudelli NM, Waterbury AL, Koblan LW, Kim YB, Badran AH, Liu DR (2017) Improved base excision repair inhibition and bacteriophage Mu Gam protein yields C:G-to-T:A base editors with higher efficiency and product purity. Science Advances 3, eaao4774.
| Crossref | Google Scholar |
Korinek V, Barker N, Moerer P, Van Donselaar E, Huls G, Peters PJ, Clevers H (1998) Depletion of epithelial stem-cell compartments in the small intestine of mice lacking Tcf-4. Nature Genetics 19, 379-383.
| Crossref | Google Scholar | PubMed |
Kuhnert F, Davis CR, Wang H-T, Chu P, Lee M, Yuan J, Nusse R, Kuo CJ (2004) Essential requirement for Wnt signaling in proliferation of adult small intestine and colon revealed by adenoviral expression of Dickkopf-1. Proceedings of the National Academy of Sciences 101, 266-271.
| Crossref | Google Scholar |
Lamas-Toranzo I, Galiano-Cogolludo B, Cornudella-Ardiaca F, Cobos-Figueroa J, Ousinde O, Bermejo-Álvarez P (2019) Strategies to reduce genetic mosaicism following CRISPR-mediated genome edition in bovine embryos. Scientific Reports 9, 14900.
| Crossref | Google Scholar | PubMed |
Lee K, Prather RS (2013) Advancements in somatic cell nuclear transfer and future perspectives. Animal Frontiers 3, 56-61.
| Crossref | Google Scholar |
Lee K, Farrell K, Uh K (2019) Application of genome-editing systems to enhance available pig resources for agriculture and biomedicine. Reproduction, Fertility and Development 32, 40-49.
| Crossref | Google Scholar | PubMed |
Lee K, Uh K, Farrell K (2020) Current progress of genome editing in livestock. Theriogenology 150, 229-235.
| Crossref | Google Scholar | PubMed |
Li W-R, Liu C-X, Zhang X-M, Chen L, Peng X-R, He S-G, Lin J-P, Han B, Wang L-Q, Huang J-C, Liu M-J (2017) CRISPR/Cas9-mediated loss of FGF5 function increases wool staple length in sheep. The FEBS Journal 284, 2764-2773.
| Crossref | Google Scholar | PubMed |
Li J, Li J, Jr, Zhang SY, Li RX, Lin X, Mi YL, Zhang CQ (2018) Culture and characterization of chicken small intestinal crypts. Poultry Science 97, 1536-1543.
| Crossref | Google Scholar | PubMed |
Li L, Fu F, Guo S, Wang H, He X, Xue M, Yin L, Feng L, Liu P (2019) Porcine intestinal enteroids: a new model for studying enteric coronavirus porcine epidemic diarrhea virus infection and the host innate response. Journal of Virology 93, e01682-18.
| Crossref | Google Scholar |
Li Y, Yang N, Chen J, Huang X, Zhang N, Yang S, Liu G, Liu G (2020) Next-generation porcine intestinal organoids: an apical-out organoid model for swine enteric virus infection and immune response investigations. Journal of Virology 94, e01006-20.
| Crossref | Google Scholar |
Li M, Guo X, Cheng L, Zhang H, Zhou M, Zhang M, Yin Z, Guo T, Zhao L, Liu H, Liang X, Li R (2024) Porcine kidney organoids derived from naïve-like embryonic stem cells. International Journal of Molecular Sciences 25, 682.
| Crossref | Google Scholar | PubMed |
Liu Y, Li X, He S, Huang S, Li C, Chen Y, Liu Z, Huang X, Wang X (2020a) Efficient generation of mouse models with the prime editing system. Cell Discovery 6, 27.
| Crossref | Google Scholar | PubMed |
Liu M, Yu W, Jin J, Ma M, An T, Nie Y, Teng C-B (2020b) Copper promotes sheep pancreatic duct organoid growth by activation of an antioxidant protein 1-dependent MEK-ERK pathway. American Journal of Physiology-Cell Physiology 318, C806-C816.
| Crossref | Google Scholar | PubMed |
Madsen O, Rikkers RSC, Wells JM, Bergsma R, Kar SK, Taverne N, Taverne-Thiele AJ, Ellen ED, Woelders H (2024) Transcriptomic analysis of intestinal organoids, derived from pigs divergent in feed efficiency, and their response to Escherichia coli. BMC Genomics 25, 173.
| Crossref | Google Scholar | PubMed |
Mao Z, Bozzella M, Seluanov A, Gorbunova V (2008) Comparison of nonhomologous end joining and homologous recombination in human cells. DNA Repair 7, 1765-1771.
| Crossref | Google Scholar | PubMed |
Miles LA, Burga LN, Gardner EE, Bostina M, Poirier JT, Rudin CM (2017) Anthrax toxin receptor 1 is the cellular receptor for Seneca Valley virus. The Journal of Clinical Investigation 127, 2957-2967.
| Crossref | Google Scholar | PubMed |
Mitchell J, Sutton K, Elango JN, Borowska D, Perry F, Lahaye L, Santin E, Arsenault RJ, Vervelde L (2024) Chicken intestinal organoids: a novel method to measure the mode of action of feed additives. Frontiers in Immunology 15, 1368545.
| Crossref | Google Scholar | PubMed |
Norris AL, Lee SS, Greenlees KJ, Tadesse DA, Miller MF, Lombardi HA (2020) Template plasmid integration in germline genome-edited cattle. Nature Biotechnology 38, 163-164.
| Crossref | Google Scholar | PubMed |
Orkin RW, Gehron P, McGoodwin EB, Martin GR, Valentine T, Swarm R (1977) A murine tumor producing a matrix of basement membrane. The Journal of Experimental Medicine 145, 204-220.
| Crossref | Google Scholar | PubMed |
Owen JR, Hennig SL, Mcnabb BR, Mansour TA, Smith JM, Lin JC, Young AE, Trott JF, Murray JD, Delany ME, Ross PJ, Van Eenennaam AL (2021) One-step generation of a targeted knock-in calf using the CRISPR-Cas9 system in bovine zygotes. BMC Genomics 22, 118.
| Crossref | Google Scholar | PubMed |
Palmiter RD, Brinster RL, Hammer RE, Trumbauer ME, Rosenfeld MG, Birnberg NC, Evans RM (1982) Dramatic growth of mice that develop from eggs microinjected with metallothionein-growth hormone fusion genes. Nature 300, 611-615.
| Crossref | Google Scholar | PubMed |
Perisse IV, Fan Z, Singina GN, White KL, Polejaeva IA (2021) Improvements in gene editing technology boost its applications in livestock. Frontiers in Genetics 11, 614688.
| Crossref | Google Scholar |
Petri K, Zhang W, Ma J, Schmidts A, Lee H, Horng JE, Kim DY, Kurt IC, Clement K, Hsu JY, Pinello L, Maus MV, Joung JK, Yeh J-RJ (2022) CRISPR prime editing with ribonucleoprotein complexes in zebrafish and primary human cells. Nature Biotechnology 40, 189-193.
| Crossref | Google Scholar | PubMed |
Pierzchalska M, Panek M, Czyrnek M, Gielicz A, Mickowska B, Grabacka M (2017) Probiotic Lactobacillus acidophilus bacteria or synthetic TLR2 agonist boost the growth of chicken embryo intestinal organoids in cultures comprising epithelial cells and myofibroblasts. Comparative Immunology, Microbiology and Infectious Diseases 53, 7-18.
| Crossref | Google Scholar | PubMed |
Pinto D, Gregorieff A, Begthel H, Clevers H (2003) Canonical Wnt signals are essential for homeostasis of the intestinal epithelium. Genes & Development 17, 1709-1713.
| Crossref | Google Scholar | PubMed |
Polejaeva IA (2021) 25th anniversary of cloning by somatic cell nuclear transfer: generation of genetically engineered livestock using somatic cell nuclear transfer. Reproduction 162, F11-F22.
| Crossref | Google Scholar | PubMed |
Powell RH, Behnke MS (2017) WRN conditioned media is sufficient for in vitro propagation of intestinal organoids from large farm and small companion animals. Biology Open 6, 698-705.
| Crossref | Google Scholar | PubMed |
Quah PS, Tran BM, Corbin VDA, Chang JJ-Y, Wong CY, Diaz-Méndez A, Hartley CA, Zeng W, Hanssen E, Trifunovic Z, Reading PC, Jackson DC, Vincan E, Coin LJM, Deliyannis G (2023) Development of matrix-embedded bovine tracheal organoids to study the innate immune response against bovine respiratory disease. Organoids 2, 82-101.
| Crossref | Google Scholar |
Redel BK, Prather RS (2016) Meganucleases revolutionize the production of genetically engineered pigs for the study of human diseases. Toxicologic Pathology 44, 428-433.
| Crossref | Google Scholar | PubMed |
Redel BK, Yoon J, Reese E, An H, Uh K, Chen PR, Prather RS, Lee K (2024) Novel off-targeting events identified after genome wide analysis of CRISPR-Cas edited pigs. The CRISPR Journal 7, 141-149.
| Crossref | Google Scholar | PubMed |
Rodríguez-Rodríguez DR, Ramírez-Solís R, Garza-Elizondo MA, Garza-Rodríguez MDL, Barrera-Saldaña HA (2019) Genome editing: a perspective on the application of CRISPR/Cas9 to study human diseases (Review). International Journal of Molecular Medicine 43, 1559-1574.
| Crossref | Google Scholar | PubMed |
Ryu J, Prather RS, Lee K (2018) Use of gene-editing technology to introduce targeted modifications in pigs. Journal of Animal Science and Biotechnology 9, 5.
| Crossref | Google Scholar | PubMed |
Sakib S, Uchida A, Valenzuela-Leon P, Yu Y, Valli-Pulaski H, Orwig K, Ungrin M, Dobrinski I (2019) Formation of organotypic testicular organoids in microwell culture. Biology of Reproduction 100, 1648-1660.
| Crossref | Google Scholar | PubMed |
Sander JD, Joung JK (2014) CRISPR-Cas systems for editing, regulating and targeting genomes. Nature Biotechnology 32, 347-355.
| Crossref | Google Scholar | PubMed |
Sato T, Vries RG, Snippert HJ, Van De Wetering M, Barker N, Stange DE, Van Es JH, Abo A, Kujala P, Peters PJ, Clevers H (2009) Single Lgr5 stem cells build crypt-villus structures in vitro without a mesenchymal niche. Nature 459, 262-265.
| Crossref | Google Scholar | PubMed |
Schwarz JS, De Jonge HR, Forrest JN, Jr (2015) Value of organoids from comparative epithelia models. The Yale Journal of Biology and Medicine 88, 367-374.
| Google Scholar | PubMed |
Sharbati J, Hanisch C, Pieper R, Einspanier R, Sharbati S (2015) Small molecule and RNAi induced phenotype transition of expanded and primary colonic epithelial cells. Scientific Reports 5, 12681.
| Crossref | Google Scholar | PubMed |
Sheets T, Park C-H, Park K-E, Powell A, Donovan D, Telugu B (2016) Somatic cell nuclear transfer followed by CRIPSR/Cas9 microinjection results in highly efficient genome editing in cloned pigs. International Journal of Molecular Sciences 17, 2031.
| Crossref | Google Scholar |
Sheltzer JM, Torres EM, Dunham MJ, Amon A (2012) Transcriptional consequences of aneuploidy. Proceedings of the National Academy of Sciences of the United States of America 109, 12644-12649.
| Crossref | Google Scholar | PubMed |
Sidik SM, Huet D, Ganesan SM, Huynh M-H, Wang T, Nasamu AS, Thiru P, Saeij JPJ, Carruthers VB, Niles JC, Lourido S (2016) A genome-wide CRISPR screen in toxoplasma identifies essential apicomplexan genes. Cell 166, 1423-1435.e12.
| Crossref | Google Scholar |
Tanihara F, Takemoto T, Kitagawa E, Rao S, Do LTK, Onishi A, Yamashita Y, Kosugi C, Suzuki H, Sembon S, Suzuki S, Nakai M, Hashimoto M, Yasue A, Matsuhisa M, Noji S, Fujimura T, Fuchimoto D-I, Otoi T (2016) Somatic cell reprogramming-free generation of genetically modified pigs. Science Advances 2, e1600803.
| Crossref | Google Scholar | PubMed |
van Der Hee B, Loonen LMP, Taverne N, Taverne-Thiele JJ, Smidt H, Wells JM (2018) Optimized procedures for generating an enhanced, near physiological 2D culture system from porcine intestinal organoids. Stem Cell Research 28, 165-171.
| Crossref | Google Scholar | PubMed |
Vilarino M, Suchy FP, Rashid ST, Lindsay H, Reyes J, Mcnabb BR, Van Der Meulen T, Huising MO, Nakauchi H, Ross PJ (2018) Mosaicism diminishes the value of pre-implantation embryo biopsies for detecting CRISPR/Cas9 induced mutations in sheep. Transgenic Research 27, 525-537.
| Crossref | Google Scholar | PubMed |
von Furstenberg RJ, Li J, Stolarchuk C, Feder R, Campbell A, Kruger L, Gonzalez LM, Blikslager AT, Cardona DM, Mccall SJ, Henning SJ, Garman KS (2017) Porcine esophageal submucosal gland culture model shows capacity for proliferation and differentiation. Cellular and Molecular Gastroenterology and Hepatology 4, 385-404.
| Crossref | Google Scholar | PubMed |
Wall RJ (1996) Transgenic livestock: progress and prospects for the future. Theriogenology 45, 57-68.
| Crossref | Google Scholar |
Whitworth KM, Prather RS (2010) Somatic cell nuclear transfer efficiency: how can it be improved through nuclear remodeling and reprogramming? Molecular Reproduction and Development 77, 1001-1015.
| Crossref | Google Scholar | PubMed |
Whitworth KM, Rowland RRR, Ewen CL, Trible BR, Kerrigan MA, Cino-Ozuna AG, Samuel MS, Lightner JE, Mclaren DG, Mileham AJ, Wells KD, Prather RS (2016) Gene-edited pigs are protected from porcine reproductive and respiratory syndrome virus. Nature Biotechnology 34, 20-22.
| Crossref | Google Scholar | PubMed |
Whitworth KM, Benne JA, Spate LD, Murphy SL, Samuel MS, Murphy CN, Richt JA, Walters E, Prather RS, Wells KD (2017) Zygote injection of CRISPR/Cas9 RNA successfully modifies the target gene without delaying blastocyst development or altering the sex ratio in pigs. Transgenic Research 26, 97-107.
| Crossref | Google Scholar | PubMed |
Whitworth KM, Green JA, Redel BK, Geisert RD, Lee K, Telugu BP, Wells KD, Prather RS (2022) Improvements in pig agriculture through gene editing. CABI Agriculture and Bioscience 3, 41.
| Crossref | Google Scholar | PubMed |
Whyte JJ, Zhao J, Wells KD, Samuel MS, Whitworth KM, Walters EM, Laughlin MH, Prather RS (2011) Gene targeting with zinc finger nucleases to produce cloned eGFP knockout pigs. Molecular Reproduction and Development 78, 2.
| Crossref | Google Scholar | PubMed |
Wiedenheft B, Sternberg SH, Doudna JA (2012) RNA-guided genetic silencing systems in bacteria and archaea. Nature 482, 331-338.
| Crossref | Google Scholar | PubMed |
Workman AM, Heaton MP, Vander Ley BL, Webster DA, Sherry L, Bostrom JR, Larson S, Kalbfleisch TS, Harhay GP, Jobman EE, Carlson DF, Sonstegard TS (2023) First gene-edited calf with reduced susceptibility to a major viral pathogen. PNAS Nexus 2, pgad125.
| Crossref | Google Scholar |
World Food Programme (2024) Global operational response plan #11. Available at https://www.wfp.org/ending-hunger [Accessed June 2024]
World Organisation for Animal Health (2024) Animal Health Welfare. Available at https://www.woah.org/en/what-we-do/animal-health-and-welfare/ [Accessed 22 February 2024]
Yang H, Wu Z (2018) Genome editing of pigs for agriculture and biomedicine. Frontiers in Genetics 9, 360.
| Crossref | Google Scholar | PubMed |
Yang X, Wu X, Wang Y, Li W, Wu X, Yuan L, Yu T, Li N, Zhang S, Hua J (2024) Induction of lung progenitor cell-like organoids by porcine pluripotent stem cells. The FASEB Journal 38, e23481.
| Crossref | Google Scholar | PubMed |
Zarei K, Stroik MR, Gansemer ND, Thurman AL, Ostedgaard LS, Ernst SE, Thornell IM, Powers LS, Pezzulo AA, Meyerholz DK, Stoltz DA (2020) Early pathogenesis of cystic fibrosis gallbladder disease in a porcine model. Laboratory Investigation 100, 1388-1399.
| Crossref | Google Scholar | PubMed |
Zhang Q, Ke H, Blikslager A, Fujita T, Yoo D (2018) Type III interferon restriction by porcine epidemic diarrhea virus and the role of viral protein nsp1 in IRF1 signaling. Journal of Virology 92 e01677-17.
| Crossref | Google Scholar |
Zhao Z, Chen X, Dowbaj AM, Sljukic A, Bratlie K, Lin L, Fong ELS, Balachander GM, Chen Z, Soragni A, Huch M, Zeng YA, Wang Q, Yu H (2022) Organoids. Nature Reviews Methods Primers 2, 94.
| Crossref | Google Scholar | PubMed |
Zhou S, Lenk LJ, Gao Y, Wang Y, Zhao X, Pan M, Huang S, Sun K, Kalds P, Luo Q, Lillico S, Sonstegard T, Scholl UI, Ma B, Petersen B, Chen Y, Wang X (2023) Generation of sheep with defined FecBB and TBXT mutations and porcine blastocysts with KCNJ5G151R/+ mutation using prime editing. BMC Genomics 24, 313.
| Crossref | Google Scholar | PubMed |
Zhu M, Qin Y-C, Gao C-Q, Yan H-C, Li X-G, Wang X-Q (2019) Extracellular glutamate-induced mTORC1 activation via the IR/IRS/PI3K/Akt Pathway enhances the expansion of porcine intestinal stem cells. Journal of Agricultural and Food Chemistry 67, 9510-9521.
| Crossref | Google Scholar | PubMed |
Zilova L, Weinhardt V, Tavhelidse T, Schlagheck C, Thumberger T, Wittbrodt J (2021) Fish primary embryonic pluripotent cells assemble into retinal tissue mirroring in vivo early eye development. eLife 10, e66998.
| Crossref | Google Scholar | PubMed |