Current status of embryo models created from pluripotent stem cells
Maria Carolina Zimara A , Toshihiko Ezashi A and Ye Yuan
A
Abstract
The mystery of genesis has fascinated human inquiry ever since Aristotle in the 4th century BCE and likely even earlier. Observations of carcinomas in the last century led to the derivation of embryonic stem cells and induced pluripotent stem cells. These remarkable cells recently allowed scientists to develop embryo models that emulate the natural developmental behavior of embryos. In this review, we present how this line of research is unfolding, and discuss its applications in human in vitro fertilization, regenerative medicine, animal reproduction and conservation. As we navigate this frontier, human genesis continues to inspire and challenge, shaping our understanding of life’s fundamental processes and opening doors to unprecedented applications of stem cell technologies.
Keywords: agriculture animal, blastoids, developmental biology, embryo models, embryogenesis, embryoids, gastrulation, human IVF, peri-implantation development, pluripotent stem cells, pre-implantation development, regenerative medicine.
Introduction
As early as 1936, some morphological similarities were noticed between early human embryos and small structures found in human testicular teratoma. These structures were subsequently termed ‘embryoid bodies’ by Peyron et al. (1936) due to their embryonic appearance (Fig. 1). This observation suggests a fascinating connection between embryology and certain cancerous formations. A few decades later, this same intuition led Leroy Stevens and his cohort to develop an interest in teratocarcinomas, unique tumors in mice that contain cells from three lineages, showcasing the same pluripotency characteristics of early embryos. Stevens’ pioneering transplantation studies in mice highlighted that these embryoid bodies originated from undifferentiated embryonal carcinoma stem cells (Stevens 1967). Remarkably, these carcinoma stem cells could be cultured in vitro and mimic early post-implantation embryo development, offering a novel model for studying human development and the principles of pluripotency.
An image of a cystic embryoid body developed by a suspension culture of embryonal carcinoma cells in vitro. Embryoid bodies are produced in a bacteriological dish by culturing a single-cell suspension of a clonal pluripotent embryonal carcinoma cell line for 4 days. After 7 or 10 days, the embryoid bodies become cystic, with a fluid-filled cavity (left) corresponding to the physiological extra-embryonic yolk sac, and a cellular mass (right) that can be compared to the fetal portion of a day 8 embryo. Sourced from fig. 8 in Martin (1975) with permission.
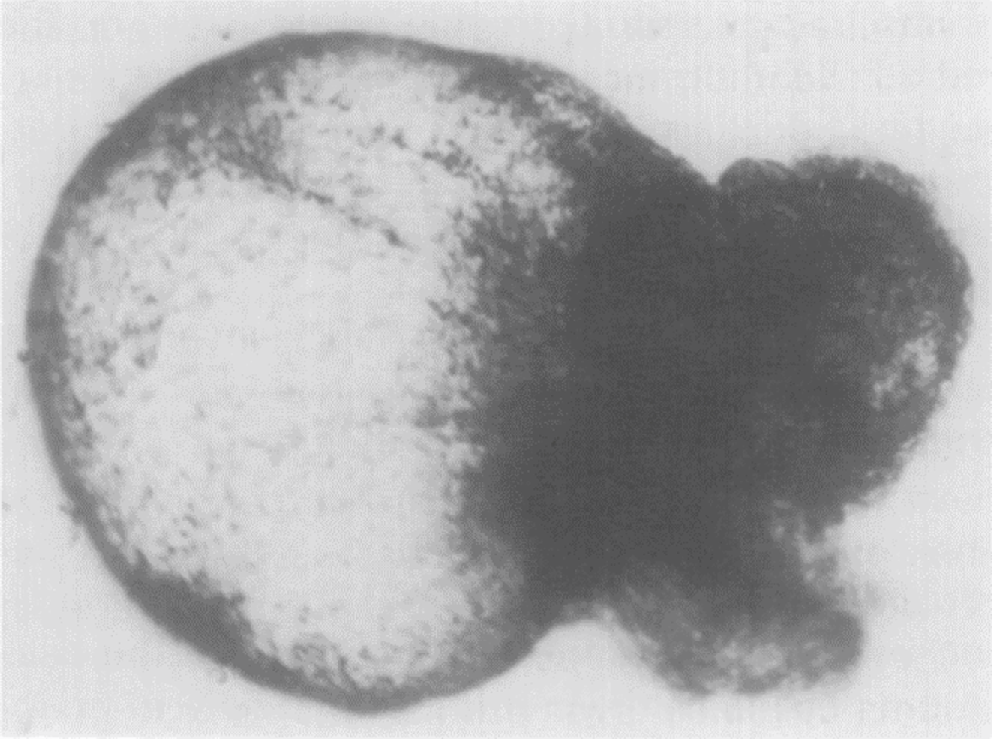
Developmental biologists extensively studied the plasticity of embryonic cells derived from teratomas. Studies on the ability of these cells to differentiate into various cell types ultimately led to a groundbreaking discovery. In 1981, Sir Martin Evans, along with Matthew Kaufman, successfully derived mouse embryonic stem cells (ESCs) from mouse embryos (Evans and Kaufman 1981). Unlike carcinoma stem cells, these mouse ESCs were karyotypically normal, a critical feature for more accurate scientific and therapeutic applications. This achievement not only transformed our understanding of pluripotency but also set Sir Evans on the path to being awarded the Nobel Prize, recognizing the profound impact of this discovery on the field of regenerative medicine and beyond.
In 1995, Dr James Thomson and his colleagues derived ESCs from a nonhuman primate species, rhesus monkey (Macaca mulatta) (Thomson et al. 1995). The physiological affinity shared between Macaca mulatta and Homo sapiens sapiens allowed culture conditions to be conveniently adapted to human ESCs (hESCs). Three years later, Thomson et al. (1998) reported the successful derivation of hESCs, not without arousing some ethical concerns from the public. Despite a generalized feeling of uneasiness hovering around these revolutionizing cell lines, scientists around the globe were able to employ it to make pivotal discoveries.
In 2006, Dr Shinya Yamanaka successfully reprogrammed mouse adult fibroblasts into induced pluripotent stem cells (iPSCs) by retrovirus-mediated transduction of four transcription factors (Myc, Klf4, Sox2, and Pou5f1, previously known as Oct3/4), that today are remembered under his name (Takahashi and Yamanaka 2006). The feasibility of iPSC unveiled new routes for investigation, and hardly a year later, Yamanaka in Japan and Thomson in America, both offered the same resolution to the moral crisis that hESCs previously generated, by proposing a protocol to reprogram adult somatic cells into human iPSCs, equivalent to hESCs (Takahashi et al. 2007; Yu et al. 2007).
The physiology of human embryogenesis has been haunting the dreams of an entire branch of scientists for centuries. While research on other mammalian embryos has been crucial, the animal study is only partially translatable and there is a need to develop scientific models that more accurately reflect human development. The ethical concerns and the practical limitations of employing human embryos for scientific purposes limited understanding of the subject. Consequently, our grasp of human embryogenesis remains incomplete. Scientists around the world have been seeking alternative approaches. The availability of various kinds of stem cells mentioned above offers ways to explore embryogenesis in a more ethical and scalable manner. Here, we review the recent progress of modeling embryogenesis using stem cells made by scientists at a breathtaking pace in recent years.
Self-organization property of embryos and stem cells
Upon implantation in the uterine endometrium, the trophectoderm (TE) gives rise to the extra-embryonic ectoderm (ectoplacental cone in mouse) and trophoblast giant cells (Gardner and Papaioannou 1975; Rossant and Cross 2001; Red-Horse et al. 2004). Simultaneously, the blastocyst’s inner cell mass epithelializes into two distinct layers known as a bilaminar embryonic disc: the epiblast (EPI) (or primitive ectoderm), which will develop into the fetus proper, and the hypoblast (or primitive endoderm (PE); visceral endoderm in the mouse), which will partially contribute to the formation of the yolk sac and regulate pro-amniotic cavity formation through apoptosis (Coucouvanis and Martin 1995). Once the primitive dorsoventral and proximodistal axes are established and embryonic and extra-embryonic regions have been delineated, the embryo undergoes a set of modifications known as gastrulation, where movement and differentiation of highly proliferative cells give rise to three distinct germ layers, namely ectoderm, mesoderm, and endoderm, that provide the lineage-specific precursors to generate adult organisms.
The formation of the embryonic axes can take place without any maternal cues. As initially shown in mice by Bedzhov and Zernicka-Goetz (2014), and subsequently confirmed in humans by Deglincerti et al. (2016) and Shahbazi et al. (2016), mammalian embryos possess amazing self-organization properties. They are capable of progressing through implantation stage to gastrulation during an extended in vitro culture without maternal input. Although it is challenging to examine human development beyond early gastrulation stage due to ethical concerns, such extended embryo culture has significantly advanced our understanding of human implantation and peri-implantation stages of embryogenesis via imaging techniques and single-cell omics (West et al. 2019; Zhou et al. 2019; Lv et al. 2019; Xiang et al. 2020). In vitro culture of cynomolgus monkey embryos has enabled researchers to bypass ethical limitations and gain insights into the mechanisms of gastrulation in primates, which may be highly relevant to humans (Ma et al. 2019; Niu et al 2019). These teams cultured monkey embryos up to embryonic day 20 and observed several critical developmental processes, including the formation of the amniotic and yolk sac cavities, appearance of the primordial germ cells, establishment of the anterior–posterior axis, and the emergence of neural groove-like structures. These findings proved that mammalian embryos possess an inherent self-organization potential that extends beyond gastrulation and includes the early stages of organogenesis.
As axis formation and gastrulation do not depend on maternal cues and are completely self-organizable, the stem cells hold much potential to recapitulate such cellular events in vitro. At the turn of the millennium, a team of researchers showed that hESC aggregates can respond to various factors, including Activin-A, transforming growth factor β (TGF-β), retinoic acid, and bone morphogenetic protein 4 (BMP4), and differentiate into cells with different epithelial or mesenchymal morphologies (Schuldiner et al. 2000). This discovery led scientists to consider using hESCs to investigate other differentiation-inhibiting and differentiation-inducing factors, resulting in a widespread use of growth factors that are nowadays considered essential in many stem cell culture and differentiation protocols. Despite the use of exogenous factors to manipulate stem cell fate, the local cellular microenvironment and spatial organization of the colonies significantly impact the fate of hESCs. Peerani et al. (2007) illustrated that the spatial organization of hESC colonies creates heterogeneous microenvironments, or niches, which affect hESC differentiation. This concept is further exemplified by Warmflash et al. (2014), who cultured hESC in circular colonies using micropatterned surfaces. Providing geometrical boundaries to a defined number of cells resulted in reproducible spatial differentiation upon treatment with BMP4. Cells positive for markers for all three germ layers and extra-embryonic tissue form concentric rings at particular radii in the colony that spontaneously recapitulate apical–basal patterning during early embryo development (Fig. 2). Such spatial patterning and self-organization behaviors were also achieved by culturing three-dimensional embryoid bodies (ten Berge et al. 2008; van den Brink et al. 2014). These stem cell features of self-organization and morphogenesis associated with gastrulation demonstrate their potential as a model system to study embryogenesis.
Recapitulating early embryonic spatial patterning with embryonic stem cells. Immunofluorescence staining for markers of different lineages in a micropatterned hESC colony 42 h after BMP4 treatment (left). Schematic of correspondence between the micropatterned hESC colony (middle) and gastrulating human embryo (right). Sourced from fig. 2 in Heemskerk and Warmflash (2016) with permission.
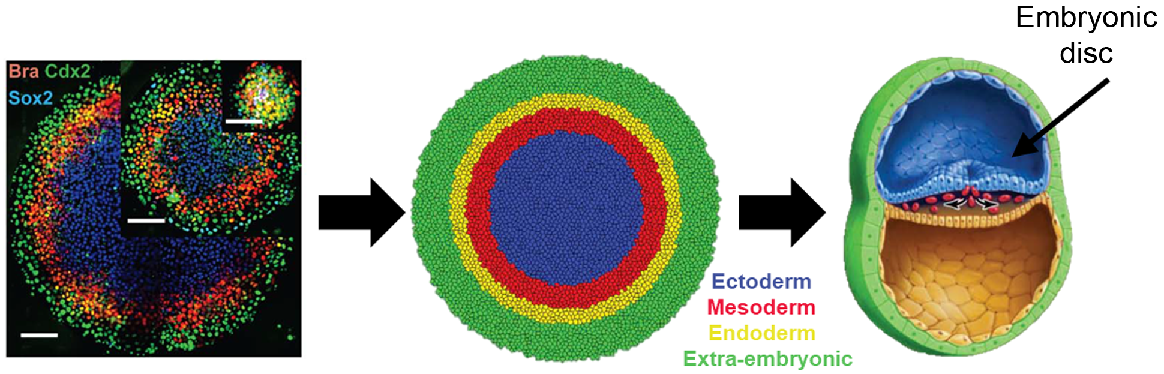
Mouse embryo models pave the way for human work
Modeling mouse peri-implantation development
The stem cell tools mentioned above, including embryoid bodies or micro-patterned colonies, are valuable but do not acquire the cellular architecture of an embryo that can accurately recapitulate the key spatial and temporal steps of embryogenesis. The breakthrough was made in 2017, when Harrison et al. (2017) combined mouse ESC and extra-embryonic trophoblast stem cells (TSCs) in a three-dimensional extracellular matrix scaffold and found that these so-called ETS-embryos were able to self-organize and develop into structures similar to that of a natural post-implantation-stage mouse embryo (Fig. 3). They were able to observe pro-amniotic cavity formation, the initiation of mesoderm differentiation in response to Wnt signaling, specification of primordial germ-cell-like cells, and the cross talk between ESCs and TSCs through Nodal/Activin signaling (Harrison et al. 2017). This is considered to be the first embryo model to mimic the morphogenetic events taking place in natural embryogenesis. One caveat of this ETS-embryo model is lack of PE; therefore, the key morphogenetic events of gastrulation were absent. Consequently, they introduced the extra-embryonic-endoderm (XEN) cells in their system, and the new structures exhibited the key spatio-temporal events of gastrulation, including the epithelial–mesenchymal transition and the specification of mesoderm and definitive endoderm (Sozen et al. 2018). Around the same time, Zhang et al. (2019) developed an ETX-embryoid model, which was assembled by co-culturing ESCs, TSCs, and XEN cells in a non-adherent-suspension-shaking system. These ETX-embryoids were similarly demonstrated to possess the ability to undergo lumenogenesis, form primordial germ-cell-like cells, and undergo gastrulation. They also demonstrated the ability to initiate implantation and trigger endometrial decidualization in mouse uterus (Zhang et al. 2019).
The first embryo model derived from stem cells. A schematic representation of mouse embryos from E4.5 to E6.5 (top) and ETS-embryos in culture between 50 h and 110 h (bottom) mimicking the development from implantation through gastrulation stages (right). The absence of primitive endoderm in the ETS-embryos is noted here. Three-dimensional depiction of canonical (top left) and ETS-embryos of 96 h (bottom left). Bars represent 30 μm. Sourced from Harrison et al. (2017) with permission.
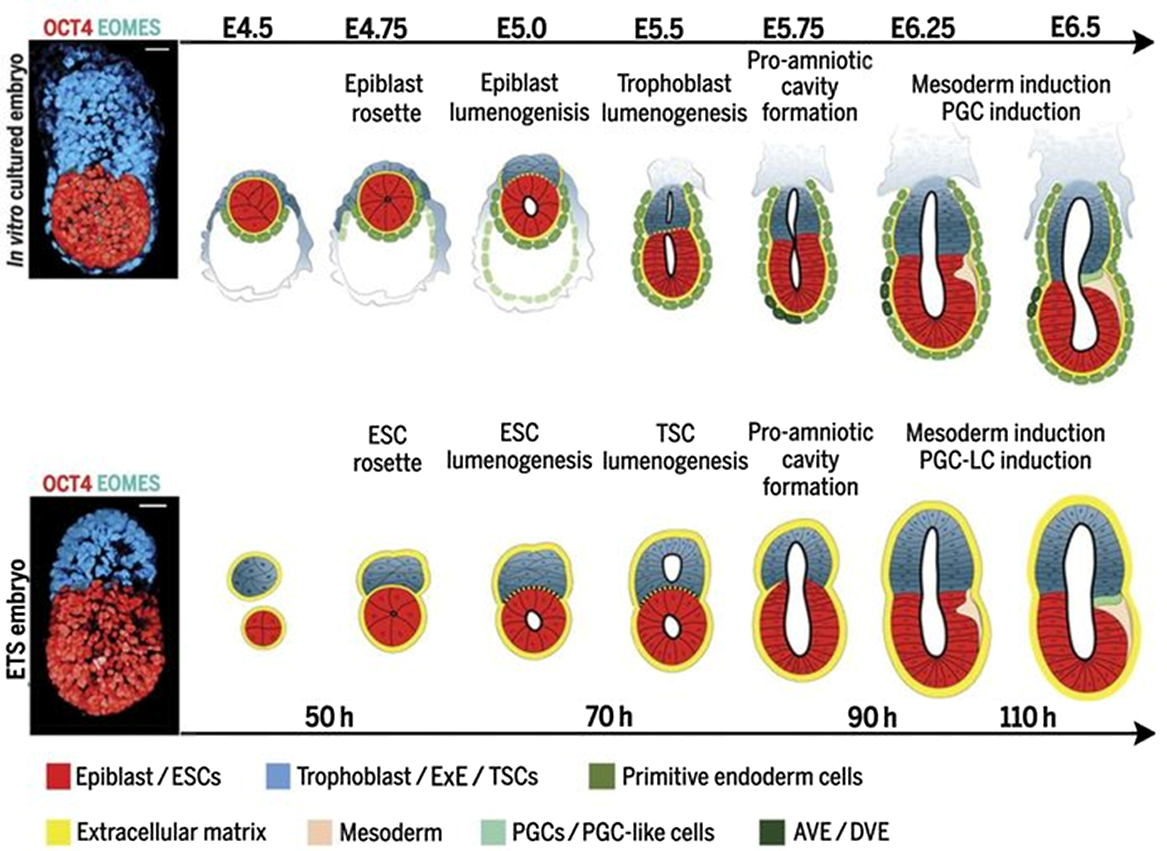
After these early successes, numerous peri-implantation and gastrulation models have emerged, which have been systematically reviewed elsewhere (Arias et al. 2022). Notably, the recent application of a rotating bottle culture system has allowed the mouse embryo models to progress beyond gastrulation and initiate early organogenesis (Aguilera-Castrejon et al. 2021). Early organogenesis events, such as brain and heart development, were also observed in several other studies (Amadei et al. 2022; Lau et al. 2022; Tarazi et al. 2022). These findings continue to make the headlines and have drawn significant attention from the general public.
Modeling mouse pre-implantation development
An equally exciting breakthrough compared to these post-implantation embryo models is the development of mouse ‘blastoids’, a term coined by Rivron et al. (2018). These blastocyst-like structures were assembled by first allowing ESCs to form non-adherent aggregates in microwells within 24 h, followed by laying TSCs onto these aggregates (Rivron et al. 2018). As the TSCs alone can also form cavities, and the blastoids do not follow the typical developmental trajectories of blastomeres undergoing compaction and cavitation, the superficial morphological resemblance between blastoids and blastocysts alone does not qualify blastoids as a novel embryo model. What is noteworthy is that the ESCs facilitated TSC proliferation and epithelial morphogenesis via FGF4/BMP4/Nodal production, which in turn activated TGF-β activities in the TSCs, a cellular crosstalk that may be only seen in blastocysts. This interaction resulted in a significantly enhanced implantation rate of blastoids compared to TSC-derived trophospheres when transferred to the uterus. However, the development of the blastoids into post-implantation embryo-like structures has yet to be achieved in this early effort, likely due to the inability of the ESCs to further segregate into EPI-like and PE-like cells. This issue was addressed by a different group by solely using the mouse extended pluripotent stem cells (EPSCs) as starting material (Li et al. 2019). The EPSCs carry the totipotent-like features that can efficiently contribute to both embryonic and extra-embryonic lineages (Yang et al. 2017a, 2017b). By treating the EPSCs with a mixture of stem cell medium and TSC differentiation medium, the cells were able to complete lineage segregation and form self-organized blastoids consisting of EPI-, PE-, and TE-like cells. These so-called EPS-blastoids also showed developmental progression to post-implantation egg cylinder structures, demonstrating striking similarities compared to mouse embryos (Li et al. 2019). By combining the EPSCs and TSCs (Sozen et al. 2019), or by inducing PE differentiation of the ESCs before introducing TSCs (Vrij et al. 2022), different groups were also able to achieve three spatially segregated lineages in their blastoid models and demonstrate their ability to form egg cylinder morphology in vitro. Additional mouse blastoid models continued to be made by chemical reprogramming of primed mouse ESC (Kime et al. 2019) or the use of totipotent stem cells (Xu et al. 2022; Yang et al. 2022; Zhang et al. 2023). However, all the blastoid models showed limited potential to progress through gastrulation after initiating implantation.
Creation of human embryo models from stem cells
Derivation of human naïve pluripotent stem cells and trophoblast stem cells
Unlike the readily available naïve mouse ESCs and TSCs, access to proper stem cell types has been a bottleneck for generating human embryo models. The derivation of human TSCs was achieved recently through activation of WNT and EGF, and inhibition of TGF-β, histone deacetylase, and Rho-associated protein kinase (Okae et al. 2018). Several naïve human pluripotency conditions were debated and turned out to be the intermediate states between naïve and primed states (Gafni et al. 2013; Ware et al. 2014). Only the 5iLA (Theunissen et al. 2014) and t2ilGo (Takashima et al. 2014) conditions met the most established molecular criteria of naïve pluripotency, making them useful for the development of human embryo models. More recently, the HENSM human naïve culture conditions (Bayerl et al. 2021) and an eight-cell-stage-like cell culture condition (Mazid et al. 2022; Taubenschmid-Stowers et al. 2022; Yu et al. 2022) were developed and may further enrich the tools for generating human embryo models.
Modeling pre-implantation development
In the wake of these breakthroughs in human stem cell derivation, two groups independently reported the generation of human blastoids at the same time (Liu et al. 2021; Yu et al. 2021a). Yu et al. (2021a) drew inspiration from their earlier success in mouse blastoid generation (Li et al. 2019) and exposed the 5iLA and PXGL (also known as t2ilGo) naïve human PSCs to hypoblast differentiation medium (HDM, Linneberg-Agerholm et al. 2019) and trophoblast differentiation medium (TDM; Okae et al. 2018) in confined microwells. The human blastoids resemble human blastocysts in morphology, lineage composition, and transcriptional profile (Fig. 4). Extended culture of these blastoids also demonstrated some features of peri-implantation development.
Human blastoids mimic human blastocyst morphology. Phase contrast pictures of human blastoids (top) and human blastocysts (bottom) reveal astounding similarities in cavitation, trophectoderm, and inner cell mass development. Bars represent 100 μm. Sourced from Yu et al. (2023) with permission.
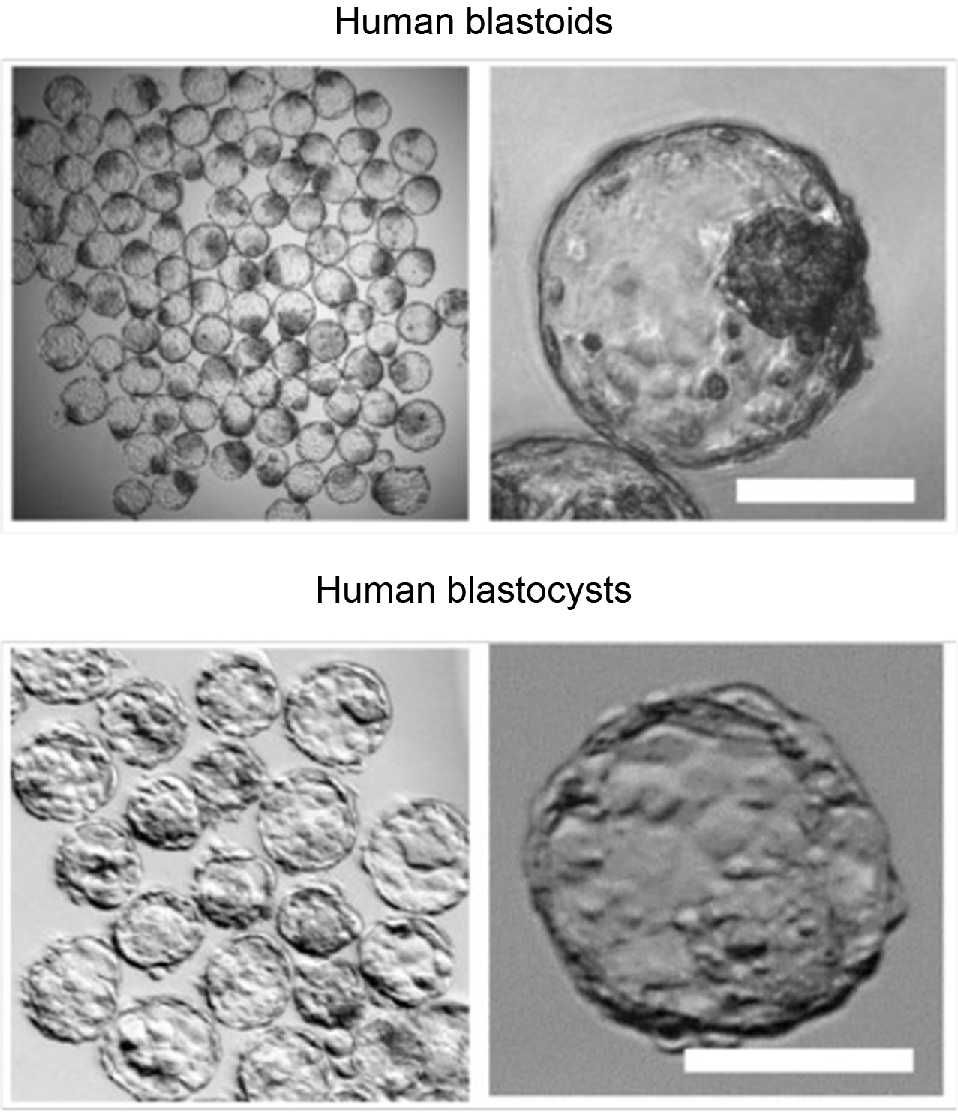
Liu et al. (2021) took a different approach, demonstrating that blastoid production is also attainable by reprogramming human dermal fibroblasts into pluripotent stem cells using a combination of POU5F1, KLF4, SOX2, and MYC. After introducing the four transcription factors into fibroblasts, the cell gene expression dramatically changed, leading to their conversion into a higher pluripotent state. By day 21 post-infection, which marks the near completion of the reprogramming process with Sendai virus carrying the four factors, the cells temporarily exhibited transcriptional signatures of the three key lineages: EPI, TE, and PE. Subsequently, on day 21, the cells in 2D culture acquired a three-dimensional structure, forming blastocyst-like cavities in microwells. Similar to the blastoids made by Yu et al. (2021a), these so-called iblastoids also resembled human blastocysts in morphology, lineage composition and transcriptomic profile. However, recent studies suggested that TE-like cells in iblastoids may represent post-implantation amniotic ectoderm cells, highlighting the risk of cell mislabeling when lineage annotations are lacking (Zhao et al. 2024; Zheng et al. 2022).
Kagawa et al. (2022) also reported the generation of human blastoids using naïve human PSCs cultured in PXGL condition. By inhibiting Hippo, TGF-β, and ERK signaling, they achieved more than 70% efficiency in blastoid generation, compared to the less than 20% efficiency reported by Yu et al. (2021a). The inhibition of the Hippo pathway seems to be key for such improved efficiency. In addition to corroborating the findings of the two earlier studies, they observed that the EPI induced local maturation of the polar TE in their blastoids, directing their attachment to endometrial cells that were previously stimulated with hormones. These observations support the claim that the blastoids are a faithful, scalable, and ethical model for investigating human implantation and development.
Optimization of the human blastoids has continued. The inclusion of a cocktail of cell apoptotic inhibitors, comprising chroman 1, emricasan, polyamines, and trans-ISRIB, in both the HDM and TDM media enhanced cell survival. When using this cocktail in combination with the inhibition of the Hippo pathway during TE differentiation, a dramatic increase in blastoid production efficiency was achieved (Yu et al. 2023). This improved efficiency could allow for the generation of around 20,000 blastoids per AggreWell plate, a decisive landmark in the scalability of this model. Moreover, this study generated the first reference single-cell RNA-seq dataset of human blastocysts using the 10X Chromium platform, confirming that earlier concerns about cell mismatches in blastoids compared to blastocysts were due to biases introduced by cross-platform comparisons. Protein analysis and chemical perturbation experiments identified PI3K/AKT, mTOR, and AMPK as indispensable pathways for human blastoid derivation, being also activated in human blastocysts. Additionally, this work also provided single-cell transcriptomic analysis to decipher the crosstalk between blastoids and endometrial stromal cells during extended culture, with human blastocysts as a side-by-side comparison. This study provided much more in-depth understanding of how blastoids mimic embryos during peri-implantation-stage development (Yu et al. 2023).
Karvas et al. (2023) also reported a refined blastoid generation protocol using a simplified blastocyst induction medium. Importantly, by culturing blastoids on a 3D matrix during extended culture, this study not only observed trophoblast expansion and invasion but also demonstrated the initiation of primitive streak formation and gastrulation, unifying post-implantation-stage embryonic and extra-embryonic development in the same model. A recent publication provided detailed protocols of how to generate blastoids and basic criteria to validate the formation of blastoids (Heidari Khoei et al. 2023). The protocol also demonstrated that much like human embryos, blastoids formed in clinical embryo culture medium can be vitrified and thawed, and continue to develop in extended culture conditions. This information allowed a broader scientific community to have access to such embryo models and establish their research programs using blastoids as an ethical alternative to human embryos.
Modeling human peri-implantation development and gastrulation
Studying human peri-implantation development and gastrulation has always been an attractive but challenging topic for developmental biologists. For several decades, knowledge of this stage of human development came from histological studies (Hertig et al. 1956; O’Rahilly and Müller 1987). Recent progress in studying the development of blastocysts in extended in vitro culture past implantation has opened new avenues for understanding these important events (Deglincerti et al. 2016; Shahbazi et al. 2016; Xiang et al. 2020). However, access to human embryos is limited, and in vitro culture of human embryos beyond 14 days after fertilization has faced both social disapproval and legal restrictions (US Department of Health, E., Welfare Ethics Advisory Board, 1979, Human Fertilization and Embryology Act 1990, Chapter 37, 1990). Therefore, embryo models made by stem cells are very attractive as ethical alternatives to human embryos.
Although human blastoids show features of amniotic cavity, yolk sac formation, and the initiation of gastrulation (Karvas et al. 2023; Yu et al. 2023), it is generally accepted that the current blastoid models have extremely low efficiency in reaching these developmental milestones, hindering their application in studying peri-implantation development and gastrulation. Such limitations may be mitigated by future development of better blastoid models and improved extended culture protocols, such as the optimization of blastoid extended culture conditions by including human endometrial stromal cells (Logsdon et al. 2022; Yu et al. 2023). In addition to human blastoids, stem cell models that recapitulate specific aspects of the developing embryos may hold bigger promises. Taking advantage of a 3D microfluidic system, human primed PSCs have been used to assemble a post-implantation amniotic sac embryoid that effectively mimics ectoderm and EPI development up to the formation of the posterior primitive streak (Shao et al. 2017). Zheng et al. (2019) and Simunovic et al. (2019) similarly recapitulated phenomena that usually accompany natural EPI and amniotic ectoderm development, including pro-amniotic cavity lumenogenesis, bipolar embryonic sac morphogenesis, primitive streak formation, and primordial germ cell specification. These studies corroborate earlier evidence that gastruloid self-organizational properties are linked to their differential polarization and inductive, or inhibitory, signal diffusion dynamics, with BMP4, WNT, and their inhibitors having a role in breaking symmetry of stem-cell-derived embryo models for human EPI development (Etoc et al. 2016). Simunovic et al. (2022) made a post-implantation embryo model that contains both embryonic and extra-embryonic tissues by assembling ESCs and extra-embryonic cells. They created the extra-embryonic portion by treating the primed ESCs with BMP4 and FGF2 on a soft substrate, which resulted in a mixture of trophoblast, amnion, and extra-embryonic mesoderm cells. This human embryo model could then be assembled by mixing the 3D EPI spheroids with single cell dissociated extra-embryonic cells. Moreover, this model can attach to the dish and achieve anteroposterior symmetry breaking and early gastrulation without exogenous morphogens (Simunovic et al. 2022).
More recently, the availability of human PSCs in more heightened pluripotent states has led to a surge in the generation of embryo models for studying peri-implantation development and gastrulation (Ai et al. 2023; Liu et al. 2023; Oldak et al. 2023; Pedroza et al. 2023; Weatherbee et al. 2023; Okubo et al. 2024; Hislop et al. 2024). These studies achieved their goals by assembling different cell types (Ai et al. 2023; Oldak et al. 2023; Weatherbee et al. 2023; Okubo et al. 2024; Hislop et al. 2024), or by aggregating a single cell type followed by sequential differentiation (Liu et al. 2023; Pedroza et al. 2023). Many of these studies demonstrated complex cellular differentiation and organization patterns containing nearly all known cell lineages proper of peri-implantation development. Bilaminar disc formation, amniotic cavity formation, anteroposterior symmetry breaking, PGC specification, yolk sac formation, and chorionic cavity formation were nicely illustrated in these embryo models. For example, by mixing wild type human iPSCs and transgene-inducible hypoblasts that express human GATA6, Hislop et al. (2024) reported multilineage yolk sac tissue formation; this tissue harbors the process of hematopoiesis. In one study, by partially differentiating EPSC aggregates in a titrated hypoblast differentiation medium, Liu et al. (2023) even generated the so-called peri-gastruloids, which hold the ability to commence neurulation and early organogenesis. Although gastrulation and organogenesis have recently been reported in mouse models, Liu et al. (2023) are the first to demonstrate the ability of a human embryo model to emulate human gastrulation and early organogenesis. This represents the state of the art of human embryo modeling. How faithfully these embryo models can mimic human development still needs further validation; however, it is undeniable that further refinement of the protocols would allow these models to be widely used as experimental tools to reveal the mystery of human peri-implantation development and gastrulation.
Creation of embryo models in other animal species
Derivation of pluripotent stem cells from domestic animals has been slow due to the lack of proper culture conditions. Many early attempts to adopt either mouse naïve-state culture conditions or human primed-state conditions failed to derive ESCs that had a stable phenotype. Additionally, most domestic animal iPSC lines depend on the continued expression of ectopic genes, which hindered their utility for further applications (reviewed in Ezashi et al. 2016).
Recent advancements in stem cell research have demonstrated that embryonic pluripotency can be captured in vitro across a spectrum of pluripotent states under different culture conditions (Wu and Izpisua Belmonte 2015). One such condition, which captures mouse and human stem cells at a region-selective pluripotent state, has been utilized to derive stable bovine ESCs using a custom TeSR medium containing FGF2 and the WNT signaling inhibitor IWR1 (CFTR) (Bogliotti et al. 2018; Yuan 2018). Building on these methods, researchers have applied the EPSC condition developed from mouse to other species. Gao et al. (2019) successfully derived porcine EPSCs capable of differentiating into three germ layers and trophoblast stem-cell-like cells. This protocol was later adapted to generate bovine EPSCs (Zhao et al. 2021). Similarly, horse ESCs were derived from blastocysts in an intermediate pluripotent state by activating FGF, TGF-β, and WNT signaling pathways (Yu et al. 2021b). The derivation of bovine TSCs was recently achieved by adopting the EPSC conditions initially developed for mice and humans, providing a new cell source for studying bovine extra-embryonic tissue development (Wang et al. 2023). These foundational achievements in domestic animal stem cells have paved the way for the recent success in generating bovine blastoids (Pinzón-Arteaga et al. 2023).
In this recent breakthrough, CFTR bovine ESCs (Bogliotti et al. 2018) were adapted to the bovine EPSC culture condition (Zhao et al. 2021) and then mixed with an equal amount of bovine TSCs (Wang et al. 2023) to generate blastoids. These bovine blastoids exhibit molecular and cellular similarities to blastocysts, can grow in suspension for more than two weeks, and initiate maternal recognition of pregnancy following embryo transfer. Although further characterization of the conceptus is needed to fully assess the blastoid developmental potential, the current model offers a valuable tool for studying bovine embryo development and early pregnancy loss (Pinzón-Arteaga et al. 2023).
Future applications
Aside from answering basic developmental biology questions, the latest advancements in embryo models have the potential for numerous translational applications that we would like to discuss here.
Embryo models for human IVF
Since the birth of the first IVF baby in 1978, the demand for human IVF has steadily increased globally. There is a constant need to improve IVF success rates, and technological advancements have become critically important to making IVF more accessible. Human embryo culture media are mainly developed based on animal models, yet only less than 50% embryos develop to blastocyst stage, indicating that suboptimal in vitro culture conditions cannot be resolved solely through animal studies. The fact that blastoids and human blastocysts share common features in signaling activation during cavitation (Yu et al. 2023) and that blastoids can adapt to clinical embryo culture medium (Heidari Khoei et al. 2023) suggests the potential for using blastoid models to optimize human embryo culture medium. The ability to produce embryo models in large quantities also has the potential to allow more thorough screening of chemical or genetic components that may impact or predict the success of implantation and pregnancy. Recently, we have shown the potential for using human blastoids to optimize embryo vitrification and warming protocols (Jannaman et al. 2023). The similarities in morphology, physical properties, and sensitivity to the in vitro environment between blastoids and human blastocysts may also make them a valuable tool for training embryologists, testing new lab devices, performing quality control in the IVF lab, and other unexplored scenarios.
Another intriguing aspect of these embryo models that could be harnessed to improve human IVF lies in their ability to generate primordial germ cells during peri-implantation development. So far, in vitro gametogenesis from stem cells has only been achieved in rodents (Hikabe et al. 2016). Chemically induced primordial germ-cell-like cells have led to human oogonia-like cell differentiation, but these cells failed to enter meiosis (Yamashiro et al. 2018). Although largely speculative, the formation of primordial germ cells through a more natural developmental process during the development of embryo models could provide more authentic materials for studying human gametogenesis in vitro. This potential application could revolutionize human IVF.
Embryo models for regenerative medicine
For many life-threating diseases, whole organ transplantation remains the sole treatment option. Yet, the world faces a shortage of donor organs. Although notable progress has been made in xenotransplantation using animal organs, tissue engineering, bioprinting, and organoid technologies, embryo models hold unique promise in the regenerative medicine field, as they echo the natural processes of differentiation and spatial organization of different cell lineages for organ formation. With recent breakthroughs in modeling human peri-implantation development and gastrulation, we may be on the brink of transforming regenerative medicine research. As reviewed eloquently by Wu and Fu (2024), embryo models offer promising potential for generating various organ primordia, such as the heart, gut tube, neural tube, somites, and more. With the development of gene-editing technologies, interspecies organogenesis, and other organ engineering technologies, the scarcity of donor organs may soon become a distant memory.
Embryo models for animal reproduction and conservation
With mouse embryo models now capable of emulating the development of limbs, brain, gut, and heart, scientists are eager to advance these models to more closely resemble bona fide embryos, aiming for full-term development and live birth. While achieving this milestone will require significant effort in mice, and even more so for a stem-cell-derived calf, such a breakthrough, as stated by Pinzón-Arteaga et al. (2023), would represent a paradigm shift in livestock reproduction. The scalability of embryo models could make nuclear transfer cloning quickly outdated. Paired with genetic editing, it may also be offered as a new approach to in vitro breeding to significantly accelerate genetic improvement in the livestock industry, as many have previously postulated through stem cell gametogenesis (Hou et al. 2018; Goszczynski et al. 2019).
Considering the recent effort in using stem cell technologies for animal conservation (Hildebrandt et al. 2018; Yoshimatsu et al. 2021), it seems logical that the possible applications of the embryo model will continue to emerge. Church and Lamm’s notable quest to defy nature and revive the mammoth has recently made progress, with the team successfully deriving iPSCs from Elephas maximus, a critical step toward achieving their final goal (Appleton et al. 2024). It is possible that the team might consider using embryo models in their continued journey.
Conclusion
The field of stem cell biology and embryo modeling is advancing at a breathtaking pace. While these technological developments are undoubtedly challenging, they also raise significant ethical and social concerns. Continuous and proactive ethical discussions are essential to establish and maintain ethical guidelines before research can take place. Engaging experts from academia, policymakers, government administrations, and the public in these dialogues is crucial to establish clear ethical guidelines, promote transparency, and ensure public engagement. This collaborative approach is vital for steering research and innovation to the desired direction.
Data availability
Data sharing is not applicable as no new data was generated or analyzed during this study.
Declaration of funding
The authors are supported by internal research funds provided by Colorado Center for Reproductive Medicine.
References
Aguilera-Castrejon A, Oldak B, Shani T, Ghanem N, Itzkovich C, Slomovich S, Tarazi S, Bayerl J, Chugaeva V, Ayyash M, Ashouokhi S, Sheban D, Livnat N, Lasman L, Viukov S, Zerbib M, Addadi Y, Rais Y, Cheng S, Stelzer Y, Keren-Shaul H, Shlomo R, Massarwa R, Novershtern N, Maza I, Hanna JH (2021) Ex utero mouse embryogenesis from pre-gastrulation to late organogenesis. Nature 593(7857), 119-124.
| Crossref | Google Scholar | PubMed |
Ai Z, Niu B, Yin Y, Xiang L, Shi G, Duan K, Wang S, Hu Y, Zhang C, Zhang C, Rong L, Kong R, Chen T, Guo Y, Liu W, Li N, Zhao S, Zhu X, Mai X, Li Y, Wu Z, Zheng Y, Fu J, Ji W, Li T (2023) Dissecting peri-implantation development using cultured human embryos and embryo-like assembloids. Cell Research 33(9), 661-678.
| Crossref | Google Scholar | PubMed |
Amadei G, Handford CE, Qiu C, De Jonghe J, Greenfeld H, Tran M, Martin BK, Chen DY, Aguilera-Castrejon A, Hanna JH, Elowitz MB, Hollfelder F, Shendure J, Glover DM, Zernicka-Goetz M (2022) Embryo model completes gastrulation to neurulation and organogenesis. Nature 610(7930), 143-153.
| Crossref | Google Scholar | PubMed |
Appleton E, Hong K, Rodríguez-Caycedo C, Tanaka Y, Ashkenazy-Titelman A, Bhide K, Rasmussen-Ivey C, Ambriz-Peña X, Korover N, Bai H, Quieroz A, Nelson J, Rathod G, Knox G, Morgan M, Malviya N, Zhang K, McNutt B, Kehler J, Kowalczyk A, Bow A, McLendon B, Cantarel B, James M, Mason CE, Gray C, Koehler KR, Pearson V, Lamm B, Church G, Hysolli E (2024) Derivation of elephant induced pluripotent stem cells. bioRxiv
| Crossref | Google Scholar |
Arias AM, Marikawa Y, Moris N (2022) Gastruloids: Pluripotent stem cell models of mammalian gastrulation and embryo engineering. Developmental Biology 488, 35-46.
| Crossref | Google Scholar |
Bayerl J, Ayyash M, Shani T, Manor YS, Gafni O, Massarwa R, Kalma Y, Aguilera-Castrejon A, Zerbib M, Amir H, Sheban D, Geula S, Mor N, Weinberger L, Naveh Tassa S, Krupalnik V, Oldak B, Livnat N, Tarazi S, Tawil S, Wildschutz E, Ashouokhi S, Lasman L, Rotter V, Hanna S, Ben-Yosef D, Novershtern N, Viukov S, Hanna JH (2021) Principles of signaling pathway modulation for enhancing human naïve pluripotency induction. Cell Stem Cell 28(9), 1549-1565.e12.
| Crossref | Google Scholar |
Bedzhov I, Zernicka-Goetz M (2014) Self-organizing properties of mouse pluripotent cells initiate morphogenesis upon implantation. Cell 156(5), 1032-1044.
| Crossref | Google Scholar | PubMed |
Bogliotti YS, Wu J, Vilarino M, Okamura D, Soto DA, Zhong C, Sakurai M, Sampaio RV, Suzuki K, Izpisua Belmonte JC, Ross PJ (2018) Efficient derivation of stable primed pluripotent embryonic stem cells from bovine blastocysts. Proceedings of the National Academy of Sciences of the United States of America 115(9), 2090-2095.
| Crossref | Google Scholar |
Coucouvanis E, Martin GR (1995) Signals for death and survival: a two-step mechanism for cavitation in the vertebrate embryo. Cell 83(2), 279-287.
| Crossref | Google Scholar | PubMed |
Deglincerti A, Croft GF, Pietila LN, Zernicka-Goetz M, Siggia ED, Brivanlou AH (2016) Self-organization of the in vitro attached human embryo. Nature 533(7602), 251-254.
| Crossref | Google Scholar | PubMed |
Etoc F, Metzger J, Ruzo A, Kirst C, Yoney A, Ozair MZ, Brivanlou AH, Siggia ED (2016) A balance between secreted inhibitors and edge sensing controls gastruloid self-organization. Developmental Cell 39(3), 302-315.
| Crossref | Google Scholar | PubMed |
Evans MJ, Kaufman MH (1981) Establishment in culture of pluripotential cells from mouse embryos. Nature 292(5819), 154-156.
| Crossref | Google Scholar | PubMed |
Ezashi T, Yuan Y, Roberts RM (2016) Pluripotent stem cells from domesticated mammals. Annual Review of Animal Biosciences 4, 223-253.
| Crossref | Google Scholar | PubMed |
Gafni O, Weinberger L, Mansour AAF, Manor YS, Chomsky E, Ben-Yosef D, Kalma Y, Viukov S, Maza I, Zviran A, Rais Y, Shipony Z, Mukamel Z, Krupalnik V, Zerbib M, Geula S, Caspi I, Schneir D, Shwartz T, Gilad S, Amann-Zalcenstein D, Benjamin S, Amit I, Tanay A, Massarwa R, Novershtern N, Hanna JH (2013) Derivation of novel human ground state naive pluripotent stem cells. Nature 504(7479), 282-286.
| Crossref | Google Scholar | PubMed |
Gao X, Nowak-Imialek M, Chen X, Chen D, Herrmann D, Ruan D, Chen ACH, Eckersley-Maslin MA, Ahmad S, Lee YL, Kobayashi T, Ryan D, Zhong J, Zhu J, Wu J, Lan G, Petkov S, Yang J, Antunes L, Campos LS, Fu B, Wang S, Yong Y, Wang X, Xue SG, Ge L, Liu Z, Huang Y, Nie T, Li P, Wu D, Pei D, Zhang Y, Lu L, Yang F, Kimber SJ, Reik W, Zou X, Shang Z, Lai L, Surani A, Tam PPL, Ahmed A, Yeung WSB, Teichmann SA, Niemann H, Liu P (2019) Establishment of porcine and human expanded potential stem cells. Nature Cell Biology 21(6), 687-699.
| Crossref | Google Scholar | PubMed |
Goszczynski DE, Cheng H, Demyda-Peyrás S, Medrano JF, Wu J, Ross PJ (2019) In vitro breeding: application of embryonic stem cells to animal production. Biology of Reproduction 100(4), 885-895.
| Crossref | Google Scholar | PubMed |
Harrison SE, Sozen B, Christodoulou N, Kyprianou C, Zernicka-Goetz M (2017) Assembly of embryonic and extraembryonic stem cells to mimic embryogenesis in vitro. Science 356(6334), eaal1810.
| Crossref | Google Scholar |
Heemskerk I, Warmflash A (2016) Pluripotent stem cells as a model for embryonic patterning: From signaling dynamics to spatial organization in a dish. Developmental Dynamics 245(10), 976-990.
| Crossref | Google Scholar | PubMed |
Heidari Khoei H, Javali A, Kagawa H, Sommer TM, Sestini G, David L, Slovakova J, Novatchkova M, Scholte Op Reimer Y, Rivron N (2023) Generating human blastoids modeling blastocyst-stage embryos and implantation. Nature Protocols 18(5), 1584-1620.
| Crossref | Google Scholar | PubMed |
Hertig AT, Rock J, Adams EC (1956) A description of 34 human ova within the first 17 days of development. American Journal of Anatomy 98(3), 435-493.
| Crossref | Google Scholar | PubMed |
Hikabe O, Hamazaki N, Nagamatsu G, Obata Y, Hirao Y, Hamada N, Shimamoto S, Imamura T, Nakashima K, Saitou M, Hayashi K (2016) Reconstitution in vitro of the entire cycle of the mouse female germ line. Nature 539(7628), 299-303.
| Crossref | Google Scholar | PubMed |
Hildebrandt TB, Hermes R, Colleoni S, Diecke S, Holtze S, Renfree MB, Stejskal J, Hayashi K, Drukker M, Loi P, Göritz F, Lazzari G, Galli C (2018) Embryos and embryonic stem cells from the white rhinoceros. Nature Communications 9(1), 2589.
| Crossref | Google Scholar | PubMed |
Hislop J, Song Q, Keshavarz FK, Alavi A, Schoenberger R, LeGraw R, Velazquez JJ, Mokhtari T, Taheri MN, Rytel M, Chuva de Sousa Lopes SM, Watkins S, Stolz D, Kiani S, Sozen B, Bar-Joseph Z, Ebrahimkhani MR (2024) Modelling post-implantation human development to yolk sac blood emergence. Nature 626(7998), 367-376.
| Crossref | Google Scholar | PubMed |
Hou Z, An L, Han J, Yuan Y, Chen D, Tian J (2018) Revolutionize livestock breeding in the future: an animal embryo-stem cell breeding system in a dish. Journal of Animal Science and Biotechnology 9, 1-11.
| Crossref | Google Scholar |
Jannaman EA, Liu K, Schoolcraft WB, Yuan Y (2023) Optimization of embryo vitrification media using stem cell derived human blastoids. Fertility and Sterility 120(4), e67-e68.
| Crossref | Google Scholar |
Kagawa H, Javali A, Khoei HH, Sommer TM, Sestini G, Novatchkova M, Scholte Op Reimer Y, Castel G, Bruneau A, Maenhoudt N, Lammers J, Loubersac S, Freour T, Vankelecom H, David L, Rivron N (2022) Human blastoids model blastocyst development and implantation. Nature 601(7894), 600-605.
| Crossref | Google Scholar | PubMed |
Karvas RM, Zemke JE, Ali SS, Upton E, Sane E, Fischer LA, Dong C, Park KM, Wang F, Park K, Hao S, Chew B, Meyer B, Zhou C, Dietmann S, Theunissen TW (2023) 3D-cultured blastoids model human embryogenesis from pre-implantation to early gastrulation stages. Cell Stem Cell 30(9), 1148-1165.e7.
| Crossref | Google Scholar |
Kime C, Kiyonari H, Ohtsuka S, Kohbayashi E, Asahi M, Yamanaka S, Takahashi M, Tomoda K (2019) Induced 2C expression and implantation-competent blastocyst-like cysts from primed pluripotent stem cells. Stem Cell Reports 13(3), 485-498.
| Crossref | Google Scholar | PubMed |
Lau KYC, Rubinstein H, Gantner CW, Hadas R, Amadei G, Stelzer Y, Zernicka-Goetz M (2022) Mouse embryo model derived exclusively from embryonic stem cells undergoes neurulation and heart development. Cell Stem Cell 29(10), 1445-1458.e8.
| Crossref | Google Scholar |
Li R, Zhong C, Yu Y, Liu H, Sakurai M, Yu L, Min Z, Shi L, Wei Y, Takahashi Y, Liao HK, Qiao J, Deng H, Nuñez-Delicado E, Rodriguez Esteban C, Wu J, Izpisua Belmonte JC (2019) Generation of blastocyst-like structures from mouse embryonic and adult cell cultures. Cell 179(3), 687-702.e18.
| Crossref | Google Scholar |
Linneberg-Agerholm M, Wong YF, Romero Herrera JA, Monteiro RS, Anderson KGV, Brickman JM (2019) Naïve human pluripotent stem cells respond to Wnt, Nodal and LIF signalling to produce expandable naïve extra-embryonic endoderm. Development 146(24), dev180620.
| Crossref | Google Scholar |
Liu X, Tan JP, Schröder J, Aberkane A, Ouyang JF, Mohenska M, Lim SM, Sun YBY, Chen J, Sun G, Zhou Y, Poppe D, Lister R, Clark AT, Rackham OJL, Zenker J, Polo JM (2021) Modelling human blastocysts by reprogramming fibroblasts into iBlastoids. Nature 591(7851), 627-632.
| Crossref | Google Scholar | PubMed |
Liu L, Oura S, Markham Z, Hamilton JN, Skory RM, Li L, Sakurai M, Wang L, Pinzon-Arteaga CA, Plachta N, Hon GC, Wu J (2023) Modeling post-implantation stages of human development into early organogenesis with stem-cell-derived peri-gastruloids. Cell 186(18), 3776-3792.e16.
| Crossref | Google Scholar |
Logsdon DM, Ezashi T, Yu L, Schoolcraft WB, Katz-Jaffe M, Wu J, Yuan Y (2022) Optimizing extended culture conditions of stem cell derived blastoids to mimic human implantation in vitro. Fertility and Sterility 118(4), e37.
| Crossref | Google Scholar |
Lv B, An Q, Zeng Q, Zhang X, Lu P, Wang Y, Zhu X, Ji Y, Fan G, Xue Z (2019) Single-cell RNA sequencing reveals regulatory mechanism for trophoblast cell-fate divergence in human peri-implantation conceptuses. PLOS Biology 17(10), e3000187.
| Crossref | Google Scholar |
Ma H, Zhai J, Wan H, Jiang X, Wang X, Wang L, Xiang Y, He X, Zhao ZA, Zhao B, Zheng P, Li L, Wang H (2019) In vitro culture of cynomolgus monkey embryos beyond early gastrulation. Science 366(6467), eaax7890.
| Crossref | Google Scholar |
Martin GR (1975) Teratocarcinomas as a model system for the study of embryogenesis and neoplasia. Cell 5(3), 229-243.
| Crossref | Google Scholar | PubMed |
Mazid MA, Ward C, Luo Z, Liu C, Li Y, Lai Y, Wu L, Li J, Jia W, Jiang Y, Liu H, Fu L, Yang Y, Ibañez DP, Lai J, Wei X, An J, Guo P, Yuan Y, Deng Q, Wang Y, Liu Y, Gao F, Wang J, Zaman S, Qin B, Wu G, Maxwell PH, Xu X, Liu L, Li W, Esteban MA (2022) Rolling back human pluripotent stem cells to an eight-cell embryo-like stage. Nature 605(7909), 315-324.
| Crossref | Google Scholar | PubMed |
Niu Y, Sun N, Li C, Lei Y, Huang Z, Wu J, Si C, Dai X, Liu C, Wei J, Liu L, Feng S, Kang Y, Si W, Wang H, Zhang E, Zhao L, Li Z, Luo X, Cui G, Peng G, Izpisúa Belmonte JC, Ji W, Tan T (2019) Dissecting primate early post-implantation development using long-term in vitro embryo culture. Science 366(6467), eaaw5754.
| Crossref | Google Scholar |
Okae H, Toh H, Sato T, Hiura H, Takahashi S, Shirane K, Kabayama Y, Suyama M, Sasaki H, Arima T (2018) Derivation of human trophoblast stem cells. Cell Stem Cell 22(1), 50-63.e6.
| Crossref | Google Scholar | PubMed |
Okubo T, Rivron N, Kabata M, Masaki H, Kishimoto K, Semi K, Nakajima-Koyama M, Kunitomi H, Kaswandy B, Sato H, Nakauchi H, Woltjen K, Saitou M, Sasaki E, Yamamoto T, Takashima Y (2024) Hypoblast from human pluripotent stem cells regulates epiblast development. Nature 626(7998), 357-366.
| Crossref | Google Scholar | PubMed |
Oldak B, Wildschutz E, Bondarenko V, Comar MY, Zhao C, Aguilera-Castrejon A, Tarazi S, Viukov S, Pham TXA, Ashouokhi S, Lokshtanov D, Roncato F, Ariel E, Rose M, Livnat N, Shani T, Joubran C, Cohen R, Addadi Y, Chemla M, Kedmi M, Keren-Shaul H, Pasque V, Petropoulos S, Lanner F, Novershtern N, Hanna JH (2023) Complete human day 14 post-implantation embryo models from naive ES cells. Nature 622(7983), 562-573.
| Crossref | Google Scholar | PubMed |
O’Rahilly R, Müller F (1987) Developmental stages in human embryos. Carnegie Institution of Washington. Available at https://www.ehd.org/developmental-stages/stage0.php
Pedroza M, Gassaloglu SI, Dias N, Zhong L, Hou TCJ, Kretzmer H, Smith ZD, Sozen B (2023) Self-patterning of human stem cells into post-implantation lineages. Nature 622(7983), 574-583.
| Crossref | Google Scholar | PubMed |
Peerani R, Rao BM, Bauwens C, Yin T, Wood GA, Nagy A, Kumacheva E, Zandstra PW (2007) Niche-mediated control of human embryonic stem cell self-renewal and differentiation. The EMBO Journal 26(22), 4744-4755.
| Crossref | Google Scholar | PubMed |
Peyron A, Limousin H, Lafay B (1936) Sur quelques notions fondamentales, dans l’étude des embryomes. Facteurs d’organisation et polyembryonie. Bulletin de l’Association Française pour l’Étude du Cancer 25, 850-873 [In French].
| Google Scholar |
Pinzón-Arteaga CA, Wang Y, Wei Y, Ribeiro Orsi AE, Li L, Scatolin G, Liu L, Sakurai M, Ye J, Ming H, Yu L, Li B, Jiang Z, Wu J (2023) Bovine blastocyst-like structures derived from stem cell cultures. Cell Stem Cell 30(5), 611-616.e7.
| Crossref | Google Scholar |
Red-Horse K, Zhou Y, Genbacev O, Prakobphol A, Foulk R, McMaster M, Fisher SJ (2004) Trophoblast differentiation during embryo implantation and formation of the maternal-fetal interface. Journal of Clinical Investigation 114(6), 744-754.
| Crossref | Google Scholar | PubMed |
Rivron NC, Frias-Aldeguer J, Vrij EJ, Boisset JC, Korving J, Vivié J, Truckenmüller RK, van Oudenaarden A, van Blitterswijk CA, Geijsen N (2018) Blastocyst-like structures generated solely from stem cells. Nature 557(7703), 106-111.
| Crossref | Google Scholar | PubMed |
Rossant J, Cross JC (2001) Placental development: lessons from mouse mutants. Nature Reviews Genetics 2(7), 538-548.
| Crossref | Google Scholar | PubMed |
Schuldiner M, Yanuka O, Itskovitz-Eldor J, Melton DA, Benvenisty N (2000) Effects of eight growth factors on the differentiation of cells derived from human embryonic stem cells. Proceedings of the National Academy of Sciences of the United States of America 97(21), 11307-11312.
| Crossref | Google Scholar |
Shahbazi MN, Jedrusik A, Vuoristo S, Recher G, Hupalowska A, Bolton V, Fogarty NME, Campbell A, Devito L, Ilic D, Khalaf Y, Niakan KK, Fishel S, Zernicka-Goetz M (2016) Self-organization of the human embryo in the absence of maternal tissues. Nature Cell Biology 18(6), 700-708.
| Crossref | Google Scholar | PubMed |
Shao Y, Taniguchi K, Townshend RF, Miki T, Gumucio DL, Fu J (2017) A pluripotent stem cell-based model for post-implantation human amniotic sac development. Nature Communications 8(1), 208.
| Crossref | Google Scholar | PubMed |
Simunovic M, Metzger JJ, Etoc F, Yoney A, Ruzo A, Martyn I, Croft G, You DS, Brivanlou AH, Siggia ED (2019) A 3D model of a human epiblast reveals BMP4-driven symmetry breaking. Nature Cell Biology 21(7), 900-910.
| Crossref | Google Scholar | PubMed |
Simunovic M, Siggia ED, Brivanlou AH (2022) In vitro attachment and symmetry breaking of a human embryo model assembled from primed embryonic stem cells. Cell Stem Cell 29(6), 962-972.e4.
| Crossref | Google Scholar |
Sozen B, Amadei G, Cox A, Wang R, Na E, Czukiewska S, Chappell L, Voet T, Michel G, Jing N, Glover DM, Zernicka-Goetz M (2018) Self-assembly of embryonic and two extra-embryonic stem cell types into gastrulating embryo-like structures. Nature Cell Biology 20(8), 979-989.
| Crossref | Google Scholar | PubMed |
Sozen B, Cox AL, De Jonghe J, Bao M, Hollfelder F, Glover DM, Zernicka-Goetz M (2019) Self-organization of mouse stem cells into an extended potential blastoid. Developmental Cell 51(6), 698-712.e8.
| Crossref | Google Scholar |
Stevens LC (1967) The biology of teratomas. Advances in Morphogenesis 6, 1-31.
| Crossref | Google Scholar | PubMed |
Takahashi K, Yamanaka S (2006) Induction of pluripotent stem cells from mouse embryonic and adult fibroblast cultures by defined factors. Cell 126(4), 663-676.
| Crossref | Google Scholar | PubMed |
Takahashi K, Tanabe K, Ohnuki M, Narita M, Ichisaka T, Tomoda K, Yamanaka S (2007) Induction of pluripotent stem cells from adult human fibroblasts by defined factors. Cell 131(5), 861-872.
| Crossref | Google Scholar | PubMed |
Takashima Y, Guo G, Loos R, Nichols J, Ficz G, Krueger F, Oxley D, Santos F, Clarke J, Mansfield W, Reik W, Bertone P, Smith A (2014) Resetting transcription factor control circuitry toward ground-state pluripotency in human. Cell 158(6), 1254-1269.
| Crossref | Google Scholar | PubMed |
Tarazi S, Aguilera-Castrejon A, Joubran C, Ghanem N, Ashouokhi S, Roncato F, Wildschutz E, Haddad M, Oldak B, Gomez-Cesar E, Livnat N, Viukov S, Lokshtanov D, Naveh-Tassa S, Rose M, Hanna S, Raanan C, Brenner O, Kedmi M, Keren-Shaul H, Lapidot T, Maza I, Novershtern N, Hanna JH (2022) Post-gastrulation synthetic embryos generated ex utero from mouse naïve ESCs. Cell 185(18), 3290-3306.e25.
| Crossref | Google Scholar |
Taubenschmid-Stowers J, Rostovskaya M, Santos F, Ljung S, Argelaguet R, Krueger F, Nichols J, Reik W (2022) 8C-like cells capture the human zygotic genome activation program in vitro. Cell Stem Cell 29(3), 449-459.e6.
| Crossref | Google Scholar |
ten Berge D, Koole W, Fuerer C, Fish M, Eroglu E, Nusse R (2008) Wnt signaling mediates self-organization and axis formation in embryoid bodies. Cell Stem Cell 3(5), 508-518.
| Crossref | Google Scholar | PubMed |
Theunissen TW, Powell BE, Wang H, Mitalipova M, Faddah DA, Reddy J, Fan ZP, Maetzel D, Ganz K, Shi L, Lungjangwa T, Imsoonthornruksa S, Stelzer Y, Rangarajan S, D’Alessio A, Zhang J, Gao Q, Dawlaty MM, Young RA, Gray NS, Jaenisch R (2014) Systematic identification of culture conditions for induction and maintenance of naïve human pluripotency. Cell Stem Cell 15(4), 471-487.
| Crossref | Google Scholar | PubMed |
Thomson JA, Kalishman J, Golos TG, Durning M, Harris CP, Becker RA, Hearn JP (1995) Isolation of a primate embryonic stem cell line. Proceedings of the National Academy of Sciences of the United States of America 92(17), 7844-7848.
| Crossref | Google Scholar |
Thomson JA, Itskovitz-Eldor J, Shapiro SS, Waknitz MA, Swiergiel JJ, Marshall VS, Jones JM (1998) Embryonic stem cell lines derived from human blastocysts. Science 282(5391), 1145-1147.
| Crossref | Google Scholar | PubMed |
van den Brink SC, Baillie-Johnson P, Balayo T, Hadjantonakis AK, Nowotschin S, Turner DA, Martinez Arias A (2014) Symmetry breaking, germ layer specification and axial organisation in aggregates of mouse embryonic stem cells. Development 141(22), 4231-4242.
| Crossref | Google Scholar | PubMed |
Vrij EJ, Scholte Op Reimer YS, Roa Fuentes L, Misteli Guerreiro I, Holzmann V, Frias Aldeguer J, Sestini G, Koo BK, Kind J, van Blitterswijk CA, Rivron NC (2022) A pendulum of induction between the epiblast and extra-embryonic endoderm supports post-implantation progression. Development 149(20), dev192310.
| Crossref | Google Scholar |
Wang Y, Ming H, Yu L, Li J, Zhu L, Sun HX, Pinzon-Arteaga CA, Wu J, Jiang Z (2023) Establishment of bovine trophoblast stem cells. Cell Reports 42(5), 112439.
| Crossref | Google Scholar | PubMed |
Ware CB, Nelson AM, Mecham B, Hesson J, Zhou W, Jonlin EC, Jimenez-Caliani AJ, Deng X, Cavanaugh C, Cook S, Tesar PJ, Okada J, Margaretha L, Sperber H, Choi M, Blau CA, Treuting PM, Hawkins RD, Cirulli V, Ruohola-Baker H (2014) Derivation of naive human embryonic stem cells. Proceedings of the National Academy of Sciences of the United States of America 111(12), 4484-4489.
| Crossref | Google Scholar |
Warmflash A, Sorre B, Etoc F, Siggia ED, Brivanlou AH (2014) A method to recapitulate early embryonic spatial patterning in human embryonic stem cells. Nature Methods 11(8), 847-854.
| Crossref | Google Scholar | PubMed |
Weatherbee BAT, Gantner CW, Iwamoto-Stohl LK, Daza RM, Hamazaki N, Shendure J, Zernicka-Goetz M (2023) Pluripotent stem cell-derived model of the post-implantation human embryo. Nature 622(7983), 584-593.
| Crossref | Google Scholar | PubMed |
West RC, Ming H, Logsdon DM, Sun J, Rajput SK, Kile RA, Schoolcraft WB, Roberts RM, Krisher RL, Jiang Z, Yuan Y (2019) Dynamics of trophoblast differentiation in peri-implantation–stage human embryos. Proceedings of the National Academy of Sciences of the United States of America 116(45), 22635-22644.
| Crossref | Google Scholar |
Wu J, Fu J (2024) Toward developing human organs via embryo models and chimeras. Cell 187(13), 3194-3219.
| Crossref | Google Scholar | PubMed |
Wu J, Izpisua Belmonte JC (2015) Dynamic pluripotent stem cell states and their applications. Cell Stem Cell 17(5), 509-525.
| Crossref | Google Scholar | PubMed |
Xiang L, Yin Y, Zheng Y, Ma Y, Li Y, Zhao Z, Guo J, Ai Z, Niu Y, Duan K, He J, Ren S, Wu D, Bai Y, Shang Z, Dai X, Ji W, Li T (2020) A developmental landscape of 3D-cultured human pre-gastrulation embryos. Nature 577(7791), 537-542.
| Crossref | Google Scholar | PubMed |
Xu Y, Zhao J, Ren Y, Wang X, Lyu Y, Xie B, Sun Y, Yuan X, Liu H, Yang W, Fu Y, Yu Y, Liu Y, Mu R, Li C, Xu J, Deng H (2022) Derivation of totipotent-like stem cells with blastocyst-like structure forming potential. Cell Research 32(6), 513-529.
| Crossref | Google Scholar | PubMed |
Yamashiro C, Sasaki K, Yabuta Y, Kojima Y, Nakamura T, Okamoto I, Yokobayashi S, Murase Y, Ishikura Y, Shirane K, Sasaki H, Yamamoto T, Saitou M (2018) Generation of human oogonia from induced pluripotent stem cells in vitro. Science 362(6412), 356-360.
| Crossref | Google Scholar |
Yang J, Ryan DJ, Wang W, Tsang JCH, Lan G, Masaki H, Gao X, Antunes L, Yu Y, Zhu Z, Wang J, Kolodziejczyk AA, Campos LS, Wang C, Yang F, Zhong Z, Fu B, Eckersley-Maslin MA, Woods M, Tanaka Y, Chen X, Wilkinson AC, Bussell J, White J, Ramirez-Solis R, Reik W, Göttgens B, Teichmann SA, Tam PPL, Nakauchi H, Zou X, Lu L, Liu P (2017a) Establishment of mouse expanded potential stem cells. Nature 550(7676), 393-397.
| Crossref | Google Scholar | PubMed |
Yang Y, Liu B, Xu J, Wang J, Wu J, Shi C, Xu Y, Dong J, Wang C, Lai W, Zhu J, Xiong L, Zhu D, Li X, Yang W, Yamauchi T, Sugawara A, Li Z, Sun F, Li X, Li C, He A, Du Y, Wang T, Zhao C, Li H, Chi X, Zhang H, Liu Y, Li C, Duo S, Yin M, Shen H, Belmonte JCI, Deng H (2017b) Derivation of pluripotent stem cells with in vivo embryonic and extraembryonic potency. Cell 169(2), 243-257.e25.
| Crossref | Google Scholar |
Yang M, Yu H, Yu X, Liang S, Hu Y, Luo Y, Izsvák Z, Sun C, Wang J (2022) Chemical-induced chromatin remodeling reprograms mouse ESCs to totipotent-like stem cells. Cell Stem Cell 29(3), 400-418.e13.
| Crossref | Google Scholar |
Yoshimatsu S, Nakajima M, Iguchi A, Sanosaka T, Sato T, Nakamura M, Nakajima R, Arai E, Ishikawa M, Imaizumi K, Watanabe H, Okahara J, Noce T, Takeda Y, Sasaki E, Behr R, Edamura K, Shiozawa S, Okano H (2021) Non-viral induction of transgene-free iPSCs from somatic fibroblasts of multiple mammalian species. Stem Cell Reports 16(4), 754-770.
| Crossref | Google Scholar | PubMed |
Yu J, Vodyanik MA, Smuga-Otto K, Antosiewicz-Bourget J, Frane JL, Tian S, Nie J, Jonsdottir GA, Ruotti V, Stewart R, Slukvin II, Thomson JA (2007) Induced pluripotent stem cell lines derived from human somatic cells. Science 318(5858), 1917-1920.
| Crossref | Google Scholar | PubMed |
Yu L, Wei Y, Duan J, Schmitz DA, Sakurai M, Wang L, Wang K, Zhao S, Hon GC, Wu J (2021a) Blastocyst-like structures generated from human pluripotent stem cells. Nature 591(7851), 620-626.
| Crossref | Google Scholar | PubMed |
Yu L, Wei Y, Sun HX, Mahdi AK, Pinzon Arteaga CA, Sakurai M, Schmitz DA, Zheng C, Ballard ED, Li J, Tanaka N, Kohara A, Okamura D, Mutto AA, Gu Y, Ross PJ, Wu J (2021b) Derivation of intermediate pluripotent stem cells amenable to primordial germ cell specification. Cell Stem Cell 28(3), 550-567.e12.
| Crossref | Google Scholar |
Yu X, Liang S, Chen M, Yu H, Li R, Qu Y, Kong X, Guo R, Zheng R, Izsvák Z, Sun C, Yang M, Wang J (2022) Recapitulating early human development with 8C-like cells. Cell Reports 39(12), 110994.
| Crossref | Google Scholar |
Yu L, Logsdon D, Pinzon-Arteaga CA, Duan J, Ezashi T, Wei Y, Ribeiro Orsi AE, Oura S, Liu L, Wang L, Liu K, Ding X, Zhan L, Zhang J, Nahar A, Stobbe C, Katz-Jaffe M, Schoolcraft WB, Tan T, Hon GC, Yuan Y, Wu J (2023) Large-scale production of human blastoids amenable to modeling blastocyst development and maternal-fetal cross talk. Cell Stem Cell 30(9), 1246-1261.e9.
| Crossref | Google Scholar | PubMed |
Yuan Y (2018) Capturing bovine pluripotency. Proceedings of the National Academy of Sciences of the United States of America 115(9), 1962-1963.
| Crossref | Google Scholar |
Zhang S, Chen T, Chen N, Gao D, Shi B, Kong S, West RC, Yuan Y, Zhi M, Wei Q, Xiang J, Mu H, Yue L, Lei X, Wang X, Zhong L, Liang H, Cao S, Belmonte JCI, Wang H, Han J (2019) Implantation initiation of self-assembled embryo-like structures generated using three types of mouse blastocyst-derived stem cells. Nature Communications 10(1), 496.
| Crossref | Google Scholar | PubMed |
Zhang P, Zhai X, Huang B, Sun S, Wang W, Zhang M (2023) Highly efficient generation of blastocyst-like structures from spliceosomes-repressed mouse totipotent blastomere-like cells. Science China Life Sciences 66(3), 423-435.
| Crossref | Google Scholar | PubMed |
Zhao L, Gao X, Zheng Y, Wang Z, Zhao G, Ren J, Zhang J, Wu J, Wu B, Chen Y, Sun W, Li Y, Su J, Ding Y, Gao Y, Liu M, Bai X, Sun L, Cao G, Tang F, Bao S, Liu P, Li X (2021) Establishment of bovine expanded potential stem cells. Proceedings of the National Academy of Sciences of the United States of America 118, e2018505118.
| Crossref | Google Scholar |
Zhao C, Reyes AP, Schell JP, Weltner J, Ortega NM, Zheng Y, Björklund ÅK, Baqué-Vidal L, Sokka J, Torokovic R, Cox B, Rossant J, Fu J, Petropoulos S, Lanner F (2024) A comprehensive human embryogenesis reference tool using single-cell RNA-sequencing data. BioRxiv [Preprint].
| Crossref | Google Scholar |
Zheng Y, Xue X, Shao Y, Wang S, Esfahani SN, Li Z, Muncie JM, Lakins JN, Weaver VM, Gumucio DL, Fu J (2019) Controlled modelling of human epiblast and amnion development using stem cells. Nature 573(7774), 421-425.
| Crossref | Google Scholar | PubMed |
Zheng Y, Yan RZ, Sun S, Kobayashi M, Xiang L, Yang R, Goedel A, Kang Y, Xue X, Esfahani SN, Liu Y, Resto Irizarry AM, Wu W, Li Y, Ji W, Niu Y, Chien KR, Li T, Shioda T, Fu J (2022) Single-cell analysis of embryoids reveals lineage diversification roadmaps of early human development. Cell Stem Cell 29(9), 1402-1419.e8.
| Crossref | Google Scholar |
Zhou F, Wang R, Yuan P, Ren Y, Mao Y, Li R, Lian Y, Li J, Wen L, Yan L, Qiao J, Tang F (2019) Reconstituting the transcriptome and DNA methylome landscapes of human implantation. Nature 572(7771), 660-664.
| Crossref | Google Scholar | PubMed |