Sedimentation from landscape clearance-induced soil erosion threatens waterhole persistence in a semi-arid river system, southern Queensland, Australia
John Tibby



A Geography, Environment and Population, University of Adelaide, Adelaide, SA 5005, Australia.
B Department of Environment and Science, Queensland Government, Brisbane, Qld, Australia.
C Australian Rivers Institute, Griffith University, Brisbane, Qld, Australia.
D Australian School of Petroleum and Energy Resources and Mawson Centre for Geoscience, University of Adelaide, Adelaide, SA 5005, Australia.
Marine and Freshwater Research 74(12) 1050-1070 https://doi.org/10.1071/MF23016
Submitted: 27 January 2023 Accepted: 25 May 2023 Published: 26 June 2023
© 2023 The Author(s) (or their employer(s)). Published by CSIRO Publishing. This is an open access article distributed under the Creative Commons Attribution-NonCommercial-NoDerivatives 4.0 International License (CC BY-NC-ND)
Abstract
Context: In arid and semi-arid river systems, waterholes are often the only refugia for aquatic organisms during no-flow spells. Sediment accumulation in waterholes reduces their persistence.
Aims: To assess this threat, we surveyed the depth of, and dated, waterhole sediments from the Moonie River, a northern tributary of Australia’s largest river system, the Murray–Darling Basin.
Methods: Fine-sediment depth was determined in three of the deepest waterholes in 2010 and 2011 before, and after, the largest flood in over a century. The rate of sediment build up in two waterholes was also determined.
Key results: In the deepest sections (>75th percentile depth), there was between 0.7 and 2 m of fine sediment in 2010. Following flooding, sediment depth reduced by 24–54%, with the largest proportional reductions in sediment occurring in the shallowest waterhole. However, net sediment accumulation is still 1.4–2.0 cm year−1 since the 1950s.
Conclusions: Sedimentation has reduced the persistence of the deepest waterholes by over 200 days, representing an up to 30% reduction. During the longest droughts known in the Moonie River, this would dry many otherwise permanent waterholes.
Implications: Sedimentation is a marked threat to waterhole persistence even following large floods.
Keywords: catchment management, climate change, ecology, erosion, floodplains, limnology, Murray–Darling Basin, sediment processes, sedimentation.
Introduction
In arid and semi-arid zone (dryland) river systems, which cease to flow for lengthy periods, deep parts of the river channel (locally termed waterholes) often represent the only permanent water bodies for hundreds of kilometres (Morón and Amos 2018). As a result, they provide drought refuges for a wide variety of plants and animals that require permanent water for all or part of their life cycle (Sheldon et al. 2010) and are therefore critical to dryland river ecosystems.
The types of aquatic biota utilising drought refuges vary spatially among waterholes and temporally at individual waterholes. Typically, there is more variability in assemblage composition in a single waterhole through time than there is variability among waterholes at a single time (Arthington et al. 2005; McGregor et al. 2006; Marshall et al. 2016). This variability is driven by occasional but critical connectivity among refuges, with population viability also dependent on rates of dispersal and recolonisation during the occasional flow events, which punctuate periods of waterhole isolation (Marshall et al. 2021). This indicates that a mosaic of waterholes in space and time is needed to maintain viable populations of all dependent aquatic species (Sheldon et al. 2010). There are three general dispersal modes evident in the life histories of dryland river biota, including the following: ‘movers’, which are not truly aquatic, can disperse by air or overland and are not reliant on hydrological connectivity; ‘networkers’, which are aquatic species restricted to refugia during isolation phases and which disperse and recolonise rapidly by hydrological connections when they occur; and ‘refugals’, which remain in refuge waterholes even when there is system connectivity (Sheldon et al. 2010). Examples of each class are common in Queensland dryland rivers (Marshall et al. 2021).
The quality of waterhole refuges is also critical to their function. In general, the longer the waterholes remain isolated and the shallower they become, the harsher are the refuge conditions. This is due to several factors, including increasing temperature, decreasing oxygen concentrations and intensifying competition, predation and disease (Magoulick 2000). Yet, aquatic populations must remain viable throughout these periods for species to persist (Sheldon et al. 2010). Waterhole quality is supported by energy subsidies from floodplain production during flood events (Woods et al. 2012), but in situ benthic algal production is vital during isolation phases (Bunn et al. 2006). In turbid systems, light limitation, in conjunction with the morphology of individual waterholes, determines autochthonous production potential, with the influence of hydrology on water depth controlling this interaction at any given time (Lobegeiger 2010; Woods et al. 2012). Thus, changes to connectivity among waterholes, their persistence during dry phases, light limitation by turbidity, and their connections to adjacent floodplains will affect their functioning as refugia and affect the diversity and resilience of the entire dryland river ecosystems (Sheldon et al. 2010; Marshall et al. 2021).
Waterhole persistence, i.e. the duration waterholes retain water in the absence of flow, is a function of the following three primary attributes: how much water is held as flow ceases; the time since flow ceased; and local processes of water loss and gain, such as rainfall, groundwater inputs, seepage loss and evaporation. Evaporation is the dominant loss factor in Australian dryland rivers (Hamilton et al. 2005). By quantifying these parameters, waterhole persistence can be modelled (Lobegeiger 2010; Department of Science, Information Technology and Innovation 2015). In a study of 15 waterholes in the Moonie River, a dryland system in the northern Murray–Darling Basin (MDB), Lobegeiger (2010) showed that measured depth loss over time, in the absence of flow, could be represented accurately by using calibrated models that incorporate waterhole bathymetry, local shallow lake evaporation and rainfall. That research showed that waterhole persistence is a consistent function of water depth, with a 170-day reduction in waterhole persistence for each metre of waterhole depth lost (Lobegeiger 2010). A very similar relationship was found for 30 waterholes elsewhere in the northern MDB (Culgoa, Narran and Barwon–Darling river valleys), with 169 days lost for each metre loss of water depth (Department of Science, Information Technology and Innovation 2015). Owing to the strong relationship between waterhole water depth and persistence, losses of waterhole depth from sediment infilling represents a threat to their persistence and refuge function (Reid et al. 2017), with some waterholes demonstrated to have been altered from permanent refugia to ones that dry because of sedimentation (Negus et al. 2015a).
The form and controls of Australian dryland river waterholes vary. Some are reach-scale, whole-of-channel features in which the channel is notably wider and deeper, others occupy only part of the channel width and may have a fixed location or change location from flow event to flow event (Wakelin-King 2022). Much of the available information about waterhole morphology, formation and ecology are from studies of reach-scale, whole-of-channel waterholes, conducted in Cooper Creek in the eastern Kati Thanda–Lake Eyre Basin (e.g. Knighton and Nanson 2000; Hamilton et al. 2005; Carini et al. 2006), and the Neales River to the west of Kati Thanda–Lake Eyre (Wakelin-King 2011; Morón and Amos 2018).
Waterholes tend to form as a result of increased substrate erodibility, where an accompanying downstream increase in floodplain width results in a planform transition from waterhole to anabranching (e.g. Morón and Amos 2018). Others form from the confinement, or coalescence of, flow (e.g. Knighton and Nanson 2000), perhaps originating from macroturbulent scour during megafloods (e.g. Wakelin-King 2011). In their studies of Cooper Creek waterholes, Knighton and Nanson (Knighton and Nanson 1994, 2000) suggested that these waterholes form at points of flow convergence, resulting in localised high-energy flow, and are self-maintaining scour features that attain more permanent status once they have scoured through the more cohesive muddy alluvium and into underlying more erodible sands. These waterholes are too irregularly distributed to be considered deepened pools in an orderly bed sequence (Knighton and Nanson 2000). Waterhole bank tops are almost always densely vegetated and easily visible on satellite imagery (Wakelin-King 2022). This riparian vegetation is likely to stabilise waterhole banks, and results in steep velocity gradients against bed and banks in waterholes, which is likely to be important for the maintenance of waterholes (Knighton and Nanson 2002).
It is likely that in wider waterhole reaches, shear stress during flow events is decreased and hydrodynamic sorting of sediment occurs (e.g. Constantine et al. 2003; Wohl and Springer 2005; Rengers and Wohl 2007; Morón and Amos 2018). In the narrower reaches between waterholes, shear stress will be increased, and the threshold for transport of available sediment may be exceeded. In some cases, the downstream end of waterholes is associated with a sediment splay, or the development of anabranching (Knighton and Nanson 2000; Morón and Amos 2018). Where there is a downstream bifurcation or transition from waterhole to multiple channels, flow velocity and shear stress reduce markedly, resulting in deposition in coarse clasts at the downstream end of waterholes (Morón and Amos 2018). Deposition of suspended sediment within waterholes will occur from water that is ponded after the cessation of flow. The occurrence and thickness of these fine-grained deposits within waterholes is likely to be a function of availability of silt and clay, which can be the result of natural processes (e.g. rainfall duration and intensity; overland flow and erosion; antecedent rainfall conditions affecting vegetation cover; erosion of upstream material from the stream bed and banks) and anthropogenic factors (e.g. agriculture, vegetation clearance). If channel-reach scours are self-maintained, the preservation potential of sediments deposited within waterholes will be low, unless there is an increase in sediment supply, or a decrease in river discharge, causing a net accumulation of sediments and reduction in waterhole depth.
Water resource development can alter the function of waterhole refugia in several ways. First, the diversion of flow from rivers and overland flow harvesting can extend the duration of periods without flow (no-flow spell duration) beyond the persistence capacity of waterholes. In the northern MDB, this happens as a result of licensed flow event harvesting into off-channel storages, or by the capture of small flow events by in-channel dams and weirs (The State of Queensland 2019). Pumping water directly from waterholes during no-flow spells also reduces their depth and thus persistence. In the northern MDB, the use of waterholes for stock and domestic water supply is a riparian landholder’s right and is unregulated (The State of Queensland 2019). Additionally, some waterholes have water entitlements that permit holders to extract water during no-flow periods (termed zero passing flow entitlements) and these are typically regulated by combinations of maximum daily or annual volume limits and cease to pump waterhole depth thresholds.
The Moonie River is a well-studied example of a northern MDB dryland river (e.g. Sternberg et al. 2008; Lobegeiger 2010; Huey et al. 2011; Bond et al. 2015; Marshall et al. 2016). In the context of the MDB, sedimentation poses a high risk to the aquatic ecology of the Moonie River, whereas hydrological changes affecting waterhole persistence pose a low risk (Negus et al. 2015a, 2015b). Water resource development in the Moonie River is minor relative to most other MDB rivers, with an end of valley reduction in mean annual flow of 27% from natural condition (Webb McKeown and Associates 2007) Most water extraction is for irrigated agriculture in the lower reaches of the Moonie, and the flow regime in the upper parts of the river where this study was undertaken is less altered. For example, at Flinton stream gauge (Fig. 1), despite a 7% increase in the total number of no-flow days, there has been no increase in the maximum no-flow spell duration, and the occurrence of flows at, or above, bankfull stage have decreased only by 10% from natural (Department of Environment and Science 2018). Therefore, the upper reaches of the Moonie River are well suited for investigation of the effects of land use on waterhole sedimentation, because of the relative absence of compounding threats from water resource development.
(a) Location of the Moonie River drainage sub-basin and study sites. (b) Plotted stream segment slope and distance along the Moonie River from the Kurmala to Kooroon waterhole sites, derived from the Australian Hydrological Geospatial Fabric. (c) Kooroon Waterhole looking upstream. (d) A plume of sediment washing down the bank into Verena Waterhole. Rain fell on this day (17 mm; 7 February 2010) and the day before (73 mm).
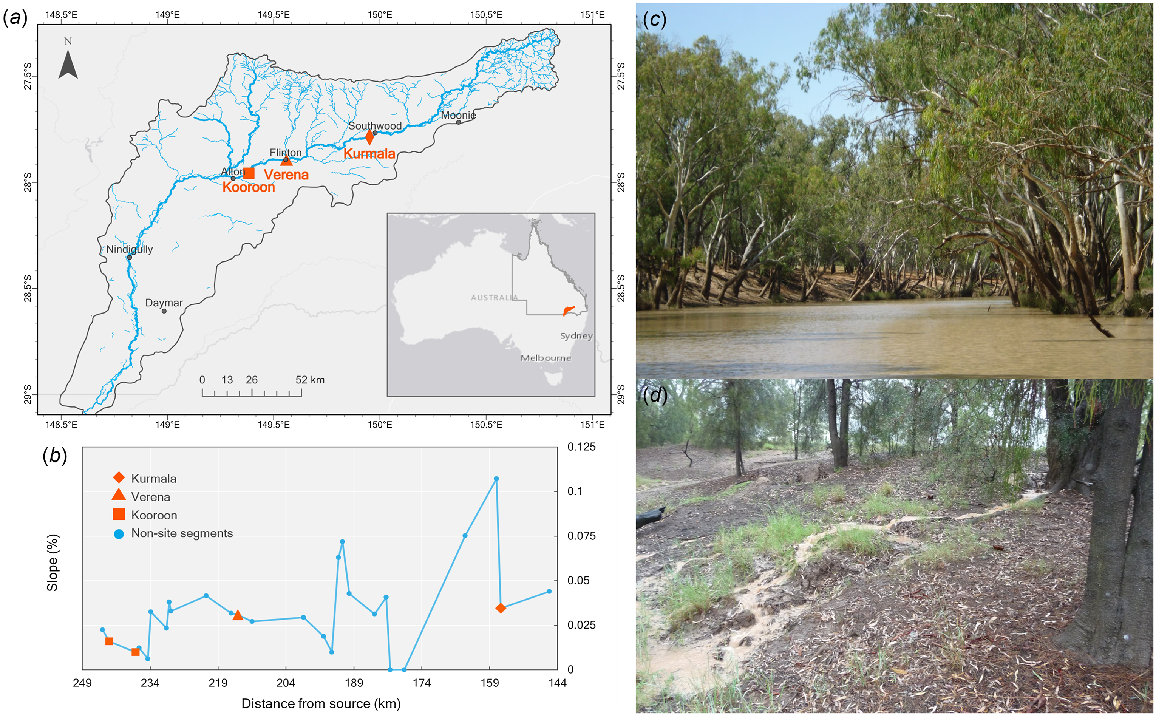
In Australia, European occupation and particularly pastoral land use mobilised large amounts of sediment across the landscape. This process resulted in unprecedented rates of sedimentation in wetlands (Gale and Haworth 2002; Reid et al. 2002). Increased rates of sediment deposition in wetlands following European colonisation have been widely observed in the MDB, inferred to result from clearing of endemic vegetation and, or, the introduction of sheep and cattle (Thoms et al. 1999; Gell et al. 2005). However, these studies have focussed on floodplain wetlands and there have been few investigations into the nature of sedimentation in in-stream waterholes in Australia and, more particularly, in the MDB. A recent investigation of deposits in four waterholes of the Lower Balonne River, in the northern MDB, concluded that there has been an increase in catchment soil erosion, sediment supply and waterhole sedimentation rate since European colonisation (Reid et al. 2017). Reid et al. (2017) estimated an additional 34–64 cm of sediment accumulation in Lower Balonne River waterholes over 150 years post-European colonisation and a reduction in waterhole depth that could have decreased waterhole persistence by 2–4 months during periods without flow. Another study of historical changes in the mapped channel morphology of the Barwon–Darling River in the northern MDB over a 120-year period found that both the maximum depths of waterholes without downstream weirs had declined by an average 1.6 m and the distance between deep waterholes had more than doubled in some reaches (Pearson et al. 2020). These changes were likely as a result of anthropogenic increases in sediment supply and a decline in the river’s flow competence to transport sediment. In Queensland, there has been substantial clearance of terrestrial vegetation to allow for agricultural expansion. In many parts of the state, this clearance escalated from the 1950s and still occurs at a high rate (Reside et al. 2017), including in the Moonie River catchment (Queensland Government 2021). Despite the potential effects of land clearance on aquatic ecosystems in Queensland, there has been little investigation of its effects (however see Tibby et al. (2019) for an example).
To further investigate the effect of land clearance and sedimentation on northern MDB, semi-arid zone rivers, we measured rates and timing of sedimentation in three deep (maximum cease to flow water depth 2.5–3.39 m, Table 1), in-stream waterholes in the Moonie River, south-western Queensland, Australia. The length and depth of these waterholes had previously been established from a survey of 113 km of river between Kurmala Station and Hollymount Road (Marshall et al. 2016; Fig. 1) and bathymetric surveys of 15 refuge waterholes throughout the catchment (Lobegeiger 2010; Supplementary Table S1). This study aimed to identify (1) the rate and drivers of sediment accumulation in Moonie River waterholes, (2) the role of large floods and scouring in mitigating such impact, and (3) the potential threat sediment accumulation poses to the function of these waterholes as drought refugia for fish and other obligate aquatic biota. To address these aims, we conducted a pilot study in 2008, to analyse and date a sediment core. In the summers of 2010 and 2011, we then probed the depth of fine sediment in three of the deepest waterholes in the system (Kooroon, Verena and Kurmala) before, and after, two major floods, and characterised and dated sediment by using radiometric techniques (14C, 210Pb and 137Cs) from cores extracted from all three sites. In addition, we examined the within-waterhole variation in sediments and, in one case, the diatom stratigraphy, to determine the nature of sedimentation (e.g. is accretion from episodic large events, more frequent small events or both?) and whether sedimentation was uniform within and between in-stream waterholes.
Item | Kooroon | Verena | Kurmala |
---|---|---|---|
Total depth to basement at CTF (m) | 5.6 | 4.05 | 4.7 |
2010 maximum water depth at CTF (m) | 3.55 | 2.55 | 3.92 |
2010 sediment depth range (m) | 0.1–2.2 | 0.25–2.55 | 0.2–1.4 |
2010 sediment depth 75th percentile (m) | 2.05 | 1.5 | 0.775 |
2011 maximum water depth at CTF (m) | 4.05 | 3.125 | 4.35 |
2011 sediment depth range (m) | 0.1–2.3 | 0.1–2.0 | 0–1.05 |
2011 sediment depth 75th percentile (m) | 1.55 | 0.925 | 0.35 |
Waterhole persistence with no sediment (days) | 861 | 600 | 709 |
Waterhole persistence with 2010 sediment persistence (days) | 515 | 346 | 579 |
Waterhole persistence with 2011 sediment persistence (days) | 600 | 443 | 650 |
Persistence increase 2010–2011 (%) | 17 | 28 | 11 |
Correlation coefficient between depth to basement and sediment depth (2011) | r2 = 0.74, P < 0.005, n = 16 | r2 = 0.80, P < 0.005, n = 19 | r2 = 0.01, P = 0.651, n = 23 |
Also shown is the relationship between the total depth to basement and 2011 sediment depth.
CTF, cease to flow.
Materials and methods
Study area
The Moonie River is located in the northern Murray–Darling Basin in south-western Queensland, and as such forms part of the headwaters of Australia’s largest river system. The catchment extends in a south-west direction from the Thomby Range in the north, to join the Barwon River ~30 km south of the Queensland–New South Wales border. The catchment covers an area of ~14 870 km2 over a river length of ~475 km (Biggs et al. 2005). The Moonie catchment has a simple dendritic structure, with persistent waterholes being largely limited to the main channel, and surface water in the sparse tributary network being highly ephemeral.
The catchment has been inhabited for more than 25 000 years and includes the traditional lands of the Bigambul, Kamilaroi and Mandandanji nations (Australian Institute of Aboriginal and Torres Strait Islander Studies 1996). European occupation for grazing began in the 1840s and economic development increased from the 1960s, following the establishment of Australia’s first commercial oilfield (O’Sullivan et al. 1991). The Moonie River catchment is heavily cleared and affected by grazing, with highly eroded banks and riparian zones (Murray–Darling Basin Authority 2021). The catchment is one of the most cleared in the MDB, with over 85% of vegetation being removed (CSIRO 2008). Remnant native vegetation covers only 17% of the catchment area, of which more than 30% is fragmented, and is predominantly brigalow scrub, mixed eucalypt woodland and open grassland (Confinas and Creighton 2001; Biggs et al. 2005). The Moonie catchment was viewed by earlier European settlers as prime livestock land (Evans 2007) and was quickly run with cattle and sheep. In 1842, there were ~100 000 sheep and 5000 cattle grazed in the catchment (Evans 2007). By 1914, there were more than 500 000 cattle (Holthouse 1978). Today, the primary land use is dryland pasture, cropping (CSIRO 2008), and livestock grazing (Yee Yet and Silburn 2003).
Climate is semi-arid with low and variable annual rainfall (mean 560 mm at Verena) and high annual evaporation (mean 2070 mm at Verena), with mean daily maximum and minimum temperatures 27 and 13°C (Marshall et al. 2016). Hydrology is rainfall-runoff dominated, with very little base-flow contribution (Sternberg et al. 2008). There is little water resource development in the catchment and the hydrology of the study reach is near-natural, although river flow is somewhat affected by abstraction further downstream (Marshall et al. 2016). Owing to the shallow gradient of the river channel, flow velocities during flow events are low and moderately expansive cracking clay floodplains throughout the mid- and low reaches of the river are inundated from several days to 2–3 weeks at a time, with an average flood event frequency of 1.5–2 years (Marshall et al. 2016 and Fig. 2). The highest flood on record occurred in March 2010 when the river rose to a height of 4.65 m at Nindigully and to 5.50 m at Thallon (Marshall et al. 2016).
Modelled discharge of the Moonie River at Flinton (Station number 417205A) 1889–2011. Also shown are the bankfull discharge volume of 13 305 ML and the timing of the three field trips for this study. Data from 28 to 30 March 1890 are truncated. Discharge was greater than 60 000 ML at this time and peaked at 90 259 ML.
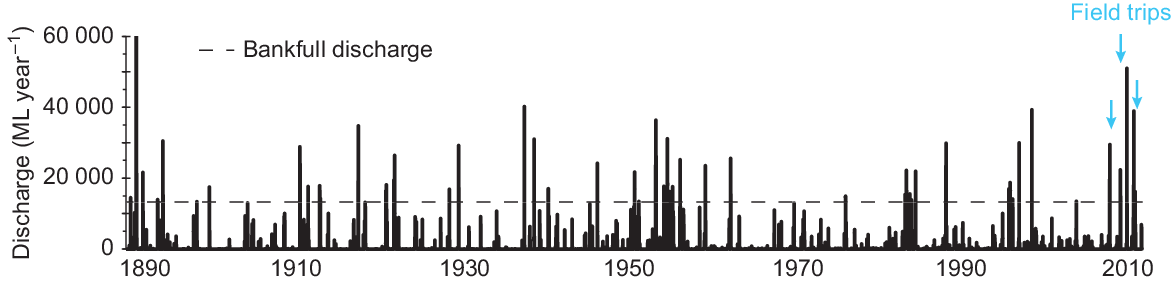
During periods without flow, which are common and can last nearly a year (Marshall et al. 2016), a series of isolated waterholes along the main river channel provide refugia for aquatic biota. This includes fish, if waterholes retain water deeper than 0.5 m (Department of Environment and Science 2018), habitat for terrestrial plants and animals, and water for human and agricultural consumption (Lobegeiger 2010). Waterholes connect only during flow events which tend to be brief and follow large rainfall events, typically during the Australian summer. Small in-stream structures (weirs and crossings) create barriers to flow, which can increase the depth and permanence of some waterholes (Marshall et al. 2016).
In this study, waterholes were defined as sections of the river channel that were >1 m deep for a sustained length (>0.5 km). The waterhole sites chosen, i.e. Kooroon, Verena and Kurmala, are located on the northern part of the river, near the township of Flinton (Fig. 1). They are relatively long and deep (0.75–6 km in length, with maximum cease-to-flow water depths between 2.8 and 4.8 m recorded in 2006), and are morphologically similar to the refugial waterholes that occur elsewhere in the catchment (Lobegeiger 2010; Table S1). We selected these waterholes because they are some of the deepest waterholes in the system (ranked first, second and eigth deepest; Tables 1, S1) and are therefore likely to represent important refuges during extended low flow periods. These waterholes have characteristics similar to those of others in the catchment (Lobegeiger 2010; Marshall et al. 2016; Marshall et al. 2021). Moonie River waterholes exhibit no notable downstream reductions in depth or in the frequency of their occurrence (Marshall et al. 2021). Hence, our findings are likely to be applicable to the whole of the Moonie system. The official site names are Moonie River at Kooroon Waterhole (Site 4172018 in the Queensland surface water database; 27.9567°S, 149.3826°E), Moonie River at Verena Waterhole (Site 4172017; 27.8948°S, 149.5599°E) and Moonie River at Kurmala Waterhole (Site 4172022; 27.78753°S, 149.9572°E).
To select the study waterholes, we used bathymetry data collected in February 2006 immediately after cessation of flow (when the waterholes were full but not flowing). This bathymetry dataset was captured using a boat mounted with a GPS (Garmin GPS 76), depth sounder (Garmin FishFinder 250) and datalogger (Rugged Brookhouse NMEA 0813) that collected paired depth–location measurements at 2-s intervals. Boat speed was kept below 6 km h−1 to ensure a consistent maximum distance between measurements. A long profile and a minimum of 40 cross-sections were traversed at each waterhole. The coordinate-depth data were then used to create three-dimensional digital elevation models (DEMs) for each waterhole by interpolating between-survey points (ESRI ArcGIS ver. 9, Topo-Raster Tool, Redlands, CA, USA). Size and volume statistics were calculated at 0.1-m depth intervals by using the ESRI ArcGIS 3D Analyst tool, to infer changes in waterhole dimensions when drying.
To determine the rate of sedimentation in Kooroon waterhole, the deepest site on the Moonie River, in February 2008, we probed eight approximately equidistant locations along the length of the waterhole and selected the site with the deepest fine sediment and extracted a single sediment core. This was collected from 2.8-m water depth with an impact corer (Chambers and Cameron 2001) and a purpose-built coring platform (Barr et al. 2013). The core was dated, and the sedimentology characterised. Building on this pilot investigation, in February 2010 and March 2011, we surveyed the water depth and used a sediment probe to determine the depth of fine sediment in three in-stream waterholes (Kooroon, Verena and Kurmala) to determine the extent of fine sediment deposition and post-flood scouring following two significant flow events. These large flow events occurred in March 2010 (the largest in over 100 years) and January 2011.
The boat-mounted depth sounder and GPS method described above was also used in February 2010 and March 2011 surveys. The depth of fine sediment was probed in the middle of the channel at a minimum of 13 regular-spaced locations along the bed, to a total length of 805, 700 and 3900 m respectively for Kooroon (Fig. 3), Verena (Fig. 4) and Kurmala (Fig. 5), with records being kept of the start and end of each waterhole. In 2011, a fallen tree blocked passage to the end of the Kurmala waterhole, which reduced the transect recorded by ~1500 m. The base of the fine sediment, defined in this study as silt and clay, although potentially containing larger-sized organic material, was judged to have been reached when probing could not penetrate further or where gravel or sand was audibly or physically detected. We term this depth the basement depth. The probing depths were compared with those collected in the sediment cores and were similar. The relationship between the depth of fine sediment and the depth to basement (i.e. the pre-infilling depth) in each waterhole was assessed with linear regression with the data from 2010 to 2011.
Waterhole bathymetry, sediment probing and coring locations for the Kooroon waterhole. S1, Site 1; C1, Core 1; etc.
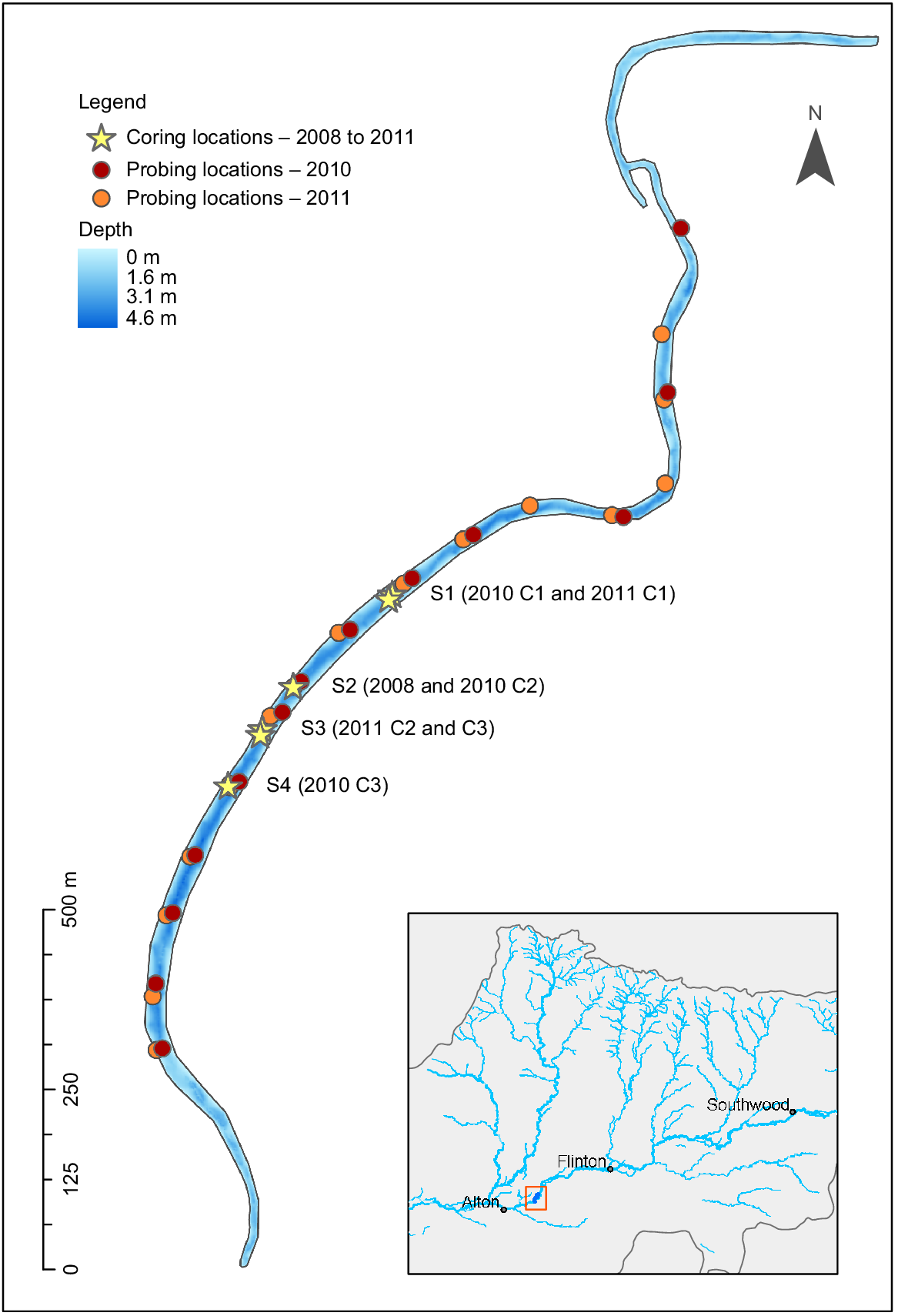
Waterhole bathymetry, sediment probing and coring locations for Verena waterhole. Note that the location for the core site in 2011 is the same as that in 2010.
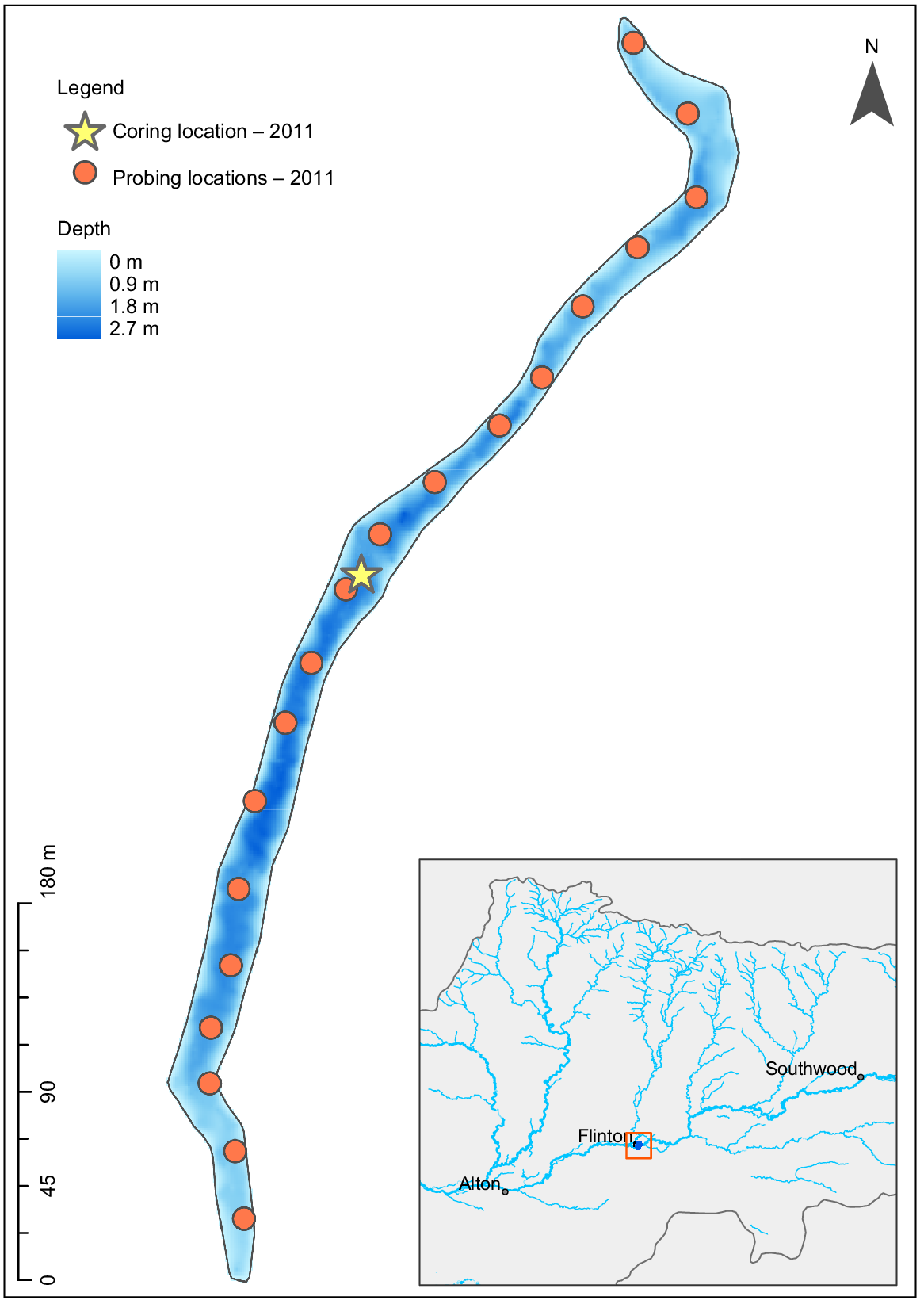
On the trips in 2010 and 2011, we also extracted several sediment cores from the three waterholes, including six from Kooroon, one from Verena and one from Kurmala. Sediment was characterised for some cores and dated for the longest cores collected in 2011 from Kooroon and Verena waterholes. Core extraction was performed using a Livingstone sampler (Livingstone 1955), which recovers a maximum of 1 m of sediment in each drive. A casing pipe fixed between the coring platform and the sediment surface facilitated taking subsequent drives from the same hole which could then be pieced together into a continuous record. Core sections were extruded into PVC trays in-field, wrapped in cling film and transported to the laboratory. The cores were split vertically on arrival at the laboratory and sampling was undertaken from uncontaminated surfaces. In 2011, two cores were extracted from the same adjacent location at Kooroon; however, the second core was shorter because coring was halted by a subterranean obstacle, possibly wood from a buried tree.
The 2008 core from Kooroon and 2011 cores from Kooroon and Verena waterholes were dated from 14C and fallout radioisotopes (210Pb and 137Cs). We assumed a constant rate of sediment compaction for each core section and corrected accordingly. We submitted identifiable terrestrial plant material for 14C dating to the University of Waikato radiocarbon dating laboratory. Radiocarbon dates were a mix of modern and pre-modern ages. Modern ages are defined as those that contain radiocarbon resulting from atmospheric nuclear testing, with the boundary between modern and pre-modern ages being post-1950 common era (CE). Pre-modern ages were calibrated in the computer program CALIB (ver. 8.2, see http://calib.org/calib/), determined using the southern hemisphere calibration curve (SHCal20) (Hogg et al. 2020) and the two sigma distribution of calendar ages were reported. Modern 14C ages were calibrated using the SH1-2 bomb curve in CALIBomb (R. W. Reimer and P. J. Reimer, see http://calib.org; Hua et al. 2022) and reported as years in CE, a secular equivalent to anno domini.
None of the three 210Pb profiles was suitable for age-model development. Hence, we used the presence of 137Cs (in combination with the modern 14C dates) as the main chronostratigraphic marker for 20th century sediments. 137Cs is detectable in sediments deposited in mid-latitude Australian locations after 1955 (Leslie and Hancock 2008) and the ability to determine this age is useful in the Moonie River catchment because widespread catchment clearance accelerated in the mid-1900s (Biggs et al. 2005). Detectabilty in the case of both 137Cs and 210Pb is defined as twice the measurement standard error for that sample. Sedimentation rates are reported as centimetres per year to one decimal place.
To understand the nature of sedimentation in the Moonie River waterholes, we applied a variety of approaches to characterise the sediment, namely, detailed sediment description, magnetic susceptibility profiling and, for the 2008 Kooroon core, diatom analysis. For each core, lithology observations at submillimetre scale were recorded in a graphic sedimentary log. Features were recorded after obtaining a clean surface of the core and included grain size and grain sorting (using a hand-lens and visual comparator), colour, bed or laminae thickness, lithological trends (e.g. grading), key surfaces, sedimentary structures and any physical, biogenic, pedogenic or diagenetic features.
Sediment magnetic susceptibility was determined at centimetre intervals on all cores by using a Bartington MS2-E probe and MS2 meter (Bartington Instruments, Witney, UK). Diatom analysis was conducted on the 2008 Kooroon waterhole core to determine whether sediments were deposited sequentially over time or as a massive deposit. We hypothesised that if the sediments were deposited in a single or small number of events, that the diatom assemblages would exhibit little sample to sample change (see for e.g. Tibby et al. 2010). Approximately 0.5 g of sediment was extracted at 5-cm depth increments. As a result of the dominance of fine clays in the sediment and the high proportion of diatom breakage, a range of methods was used to obtain the best samples. We used standard 10% hydrochloric acid and 30% hydrogen peroxide treatments to remove carbonates and organic matter respectively. Density separation was then used to remove particles of >250 μm. Following these steps, the diatom samples were still impure. Hence, samples were then prepared with a 50:50 molar ratio of concentrated nitric and sulfuric acid and heated in an 80°C bath for 100 min, following Cremer et al. (2001). The slurries were then washed three times with distilled water, with overnight settling after each wash. Slides were made securing the cover slips to the slides by using Naphrax on a hot plate.
To assess the impact of accumulated sediment on waterhole persistence time and, thus, drought refuge function, persistence was modelled under the following three sediment scenarios developed from the probing data:
A ‘no sediment’ scenario: the maximum water depth plus fine sediment depth for each waterhole, adjusted to the cease-to-flow water level. The location of maximum depth represents the deepest, and therefore, most persistent part of the waterhole in the absence of deposited sediments.
A pre-flood scenario: the maximum total depth as above, minus the fine sediment depth measured at each waterhole during the 2010 sampling.
A post-flood scenario: the maximum total depth as above, minus the fine sediment depth measured at each waterhole during the 2011 sampling.
The ‘fine sediment depth’ value for each waterhole was calculated as the 75th percentile of probed sediment depths for that sampling site and year. This provided a summary metric of the deeper sediments, excluding the very shallow sediments at the waterhole ends, and overcame variability in the single maximum sediment depth value associated with fine-scale patterns in sediment deposition and slight differences in probing locations between sampling occasions.
Persistence time was defined as the time it takes a waterhole to dry to a depth of less than 0.5 m during a period without flow inputs. The 0.5 m threshold represents the point at which a waterhole is likely to stop providing suitable aquatic habitat because of steep declines in water quality (e.g. Wallace et al. 2015; Zhai et al. 2022). A model that predicts maximum waterhole persistence time in the absence of water inputs from flow or rainfall had previously been developed for northern Murray–Darling waterholes (Department of Science, Information Technology and Innovation 2015) on the basis of measured water loss behaviour from multiple waterholes. Waterhole persistence (days) was calculated from water depth (m) by using the formula
The model was applied to our waterhole water depths to calculate estimated maximum persistence time under each sediment scenario. Surveyed water depths at the times of the 2010 and 2011 probing surveys were standardised to cease-to-flow depth for each waterhole as the datum for comparisons. Water depths under Scenarios 2 and 3 were relative to the maximum probed basement depth (i.e. under scenario one) at each waterhole. These persistence estimates were compared with the durations of all no-flow spells (when waterholes are at risk of drying) between 1889 and 2016, derived from a modelled daily flow-time series for the nearest model node to each waterhole (Department of Environment and Science 2019) by using EcoModeller (ver. 2.1.2, see https://toolkit.ewater.org.au/Tools/Eco-Modeller). This allowed us to evaluate the effects of fine sediment accumulation on waterhole permanency.
Results
Depth of fine sediment before, and after, large flood events
Sediment probing surveys in 2010 of the three study sites indicated that up to 2.55 m of fine sediment had accumulated in the waterholes above basement levels (Table 1). There was substantial variation in fine sediment depth both between and within waterholes (Table 1). Kurmala waterhole had the shallowest fine sediment accumulation, with an average depth of 0.6 m in 2010. Verena and Kooroon waterholes contained substantially more sediment (Table 1). Regarding the within-waterhole sediment variability, there was a strong tendency for sediment to be deposited in the (formerly) deepest parts of the channel. The relationship between fine sediment depth and the water depth to basement was both strong and statistically significant for the two deepest sites, Verena and Kooroon (Table 1).
There was considerable variation in both the depth and composition of fine sediment over short distances in all three waterholes (Fig. 3, 4, 5). All three waterholes had markedly less sediment after the 2011 flood than before the 2010 flood, with sediment depth reductions of 33–58% (Table 1). Despite these reductions, the maximum depth of fine sediment in the Verena and Kooroon waterholes, following the largest flood in more than 100 years (March 2010), still exceeded 2 m.
Sediment composition
Overall, the cores were dominated by silty-clay lithofacies, with frequent sandy laminae and thin sand beds (1–4 cm thick), and occasional thicker clayey-sand, silty-sand and sand beds of 10–32 cm thick (Fig. 6). These thicker sand beds were found in each waterhole (Kooroon, Kurmala and Verena), in both 2010 and 2011 cores, but not at every core location. Sand sizes in the beds and laminae were dominantly lower very fine to lower medium, with up to upper coarse sand in some Kooroon cores (both 2010 and 2011), and up to very coarse sand in Kurmala and Verena cores. Organic particles, comprising leaf and twig fragments, were abundant, with the exception of the most downstream locations in the Kooroon waterhole (Sites 3 and 4) and the Verena core. The most downstream of the Kooroon core locations were also the least sandy. The Verena core is notably different from Kooroon in that its thicker sand beds did not contain any organic clasts. All cores except the Kurmala core contained thin (1–3 cm) beds of wetter, paler-grey colour clay, interpreted (by feel) to contain no silt. One ripple cross-laminated bed was observed (Koroon Site 4 2010, at 32-cm core depth); a 1-cm-thick medium sand bed preserving ripple foresets and overlying mud drape. Sand-filled desiccation cracks were interpreted in the most downstream locations in the Kooroon waterhole (Sites 3 and 4); observations were vertically aligned pockets of orange-coloured medium sand. Dark orange oxidation layers were observed in the Kooroon Site 1 (2010) core.
Sediment stratigraphy diagram. Ages shown on the y-axis are the minimum age for the corresponding depth. Depths are displayed as the core depth (CD) represented the compacted sediment depth. Uncompacted or true depths (TD) are also shown. Missing core sections are those that were sampled for 14C ages. Also shown are sediment ‘units’, which, although variable, have distinct characteristics that may be shared between the 2010 and 2011 Kooroon cores.
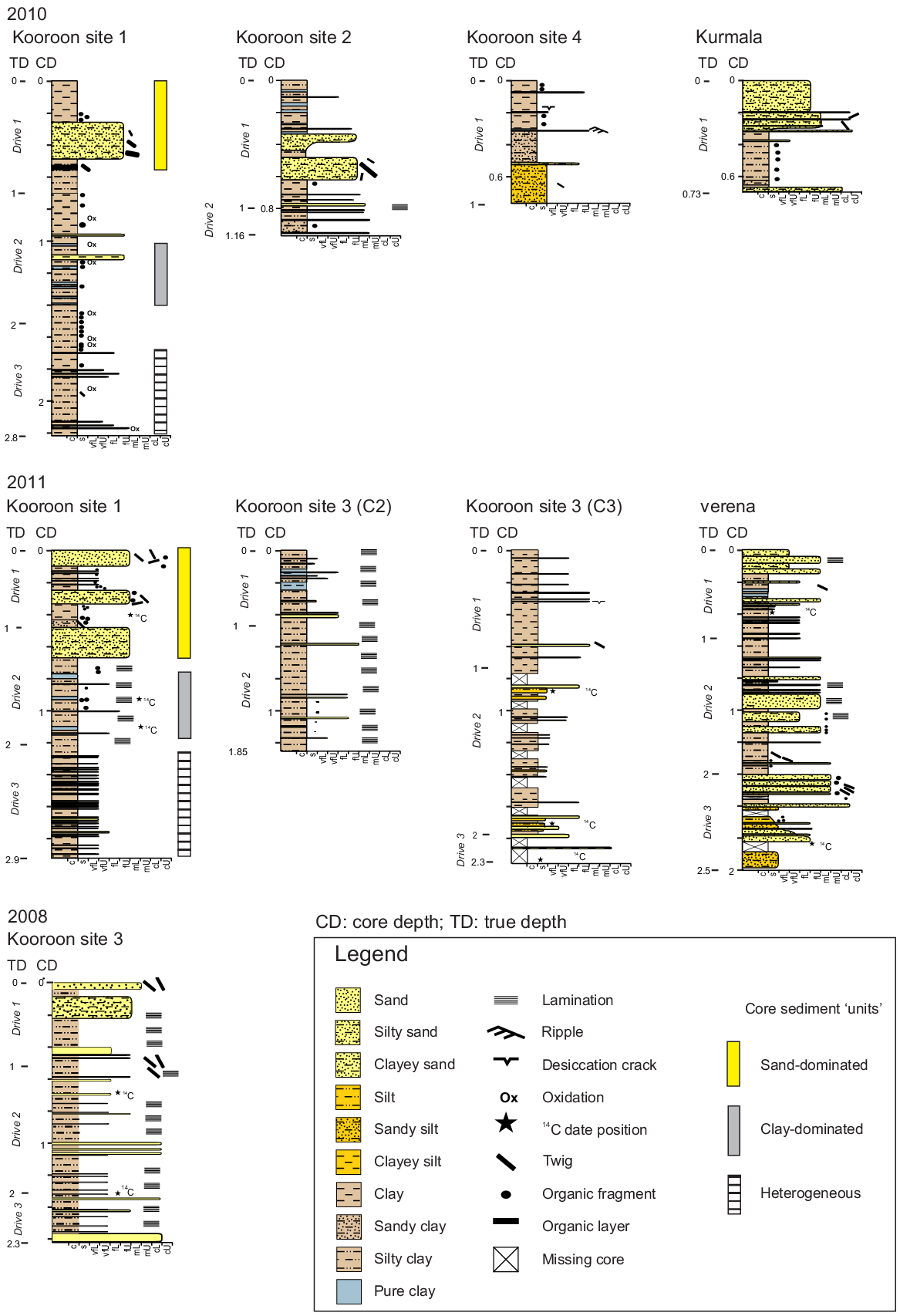
The diatom assemblage of the 2008 Kooroon core (Fig. S6) was dominated by broken diatoms, many of which could not be identified to species level. Of those diatoms that could be identified, Stauroneis phoenicentron and Pinnularia spp. were represented in the lower part of the record, but not above 170 cm. Other taxa, in particular Epithemia spp., were better represented in the upper part of the record.
Age of the sediment
Dates were obtained from three cores, including the 2008 core from Kooroon waterhole, Core 3 extracted in 2011 from Kooroon waterhole (in a location aligned with that from 2008) and a single core extracted in 2011 from Verena waterhole. Two radiocarbon dates were obtained from the 2008 Kooroon core. These indicated that sediment from a depth of 128–129 cm was deposited in, or after, 2003 CE, whereas that from a depth of 202–203 cm was deposited in, or after, 1959 CE. A detectable concentration of 137Cs was found in the 80.8–92.3-cm sample, the deepest analysed, but this does not add chronological information given the radiocarbon dates. In contrast to the 2008 core, 14C dating of the 2011 Kooroon core returned two pre-modern ages, with the deeper date being younger than the upper (Table S2). The youngest 14C date (from 87.5 to 88.5 cm) was modern in age, potentially as old as 1957 CE. Lower samples, 169–171 and 189–193 cm returned pre-modern median calibrated ages of 1764 and 1776 CE respectively. As a result, 14C ages from the 2011 Kooroon core do not alter the depth at which the post-colonisation sediment was definitely detected. However, the probability distributions of the calibrated ages do include the possibility that they were deposited after 1950. 137Cs was detectable in the 105–111-cm sample, indicating that these sediments were deposited in, or after, the mid-1950s. No 210Pbex was detected below this depth.
Radiocarbon dating of three samples from the 2011 Verena waterhole core (Table S2) indicated that the sediments, including those from 35.5 to 37 cm deep, had median probable ages ranging from 1763 to 1844 CE. However, the calibrated ages include the possibility that the material was modern in age (i.e. post-1950). 137Cs was detected at 67–77 cm, whereas 210Pbex was detected at 128–134 cm. The presence of 210Pbex indicates, in contrast to the most probable calibrated 14C dates, that the sediments accumulated in the post-European colonisation period. 210Pbex is detectable in lake sediments, in ideal conditions for up to 150 years (Appleby 2001). Ideal conditions for detection of 210Pb in old sediment occur when the 210Pb flux from the atmosphere dominates 210Pb sources in the sediment and 210Pbex exhibits monotonic decay. Neither of these conditions occur in the Moonie River, and thus it is not possible to utilise the 210Pbex profile to determine sedimentation rates. Nevertheless, we conservatively adopt 150 years before coring (i.e. 1861 CE) as the oldest possible age represented by the presence of 210Pbex. Hence, this means that the accumulation rate in Verena waterhole from 1861 to 1955 CE was, at a minimum, 0.5 cm year−1. The 137Cs data indicate that since the mid-1950s, sediments have accumulated at a minimum of 1.4 cm year−1.
Waterhole persistence with, and without, fine sediment
Maximum persistence time was substantially reduced (5–35%) under the two deposited sediment scenarios in comparison to the no-sediment scenario (Table 1, Fig. 7). The large floods in March 2010 and January 2011 reduced accumulated sediment depths and increased waterhole persistence times at all studied waterholes by 11–28%, but did not restore them to no-sediment conditions. Waterhole persistence under all sediment scenarios exceeded the maximum no-flow spell (315 days under both pre-development and full entitlement hydrology scenarios, Table 2) from the 127-year hydrology simulation. This means that these waterholes would not have dried up over this period, regardless of the scenario.
Modelled waterhole persistence time (days) under different sediment scenarios. Horizontal lines represent the maximum period without flow in the region on the basis of gauged and modelled hydrology data from Flinton (Gauge 417205A).
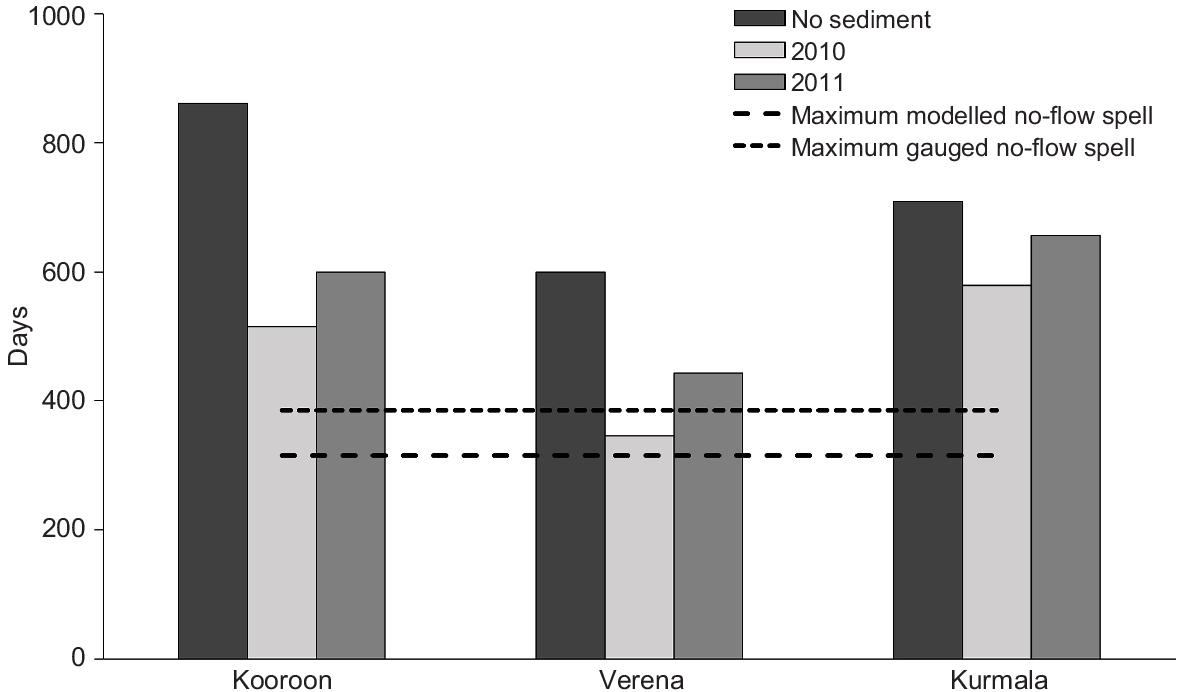
No-flow spells | 417205A Moonie River at Flinton | 417201B Moonie River at Nindigully | 417204A Moonie River at Fenton | |||
---|---|---|---|---|---|---|
Pre-development | Full entitlement | Pre-development | Full entitlement | Pre-development | Full entitlement | |
Maximum (days) | 315 | 315 | 496 | 797 | 728 | 847 |
Median (days) | 10 | 12 | 19 | 18 | 47 | 48 |
Number of spells | 1831 | 1359 | 627 | 539 | 334 | 331 |
Total duration (days) | 33 005 | 35 069 | 30 658 | 32 850 | 34 080 | 35 360 |
Two surface-water development scenarios have been simulated, namely, pre-development, which assumes no water resource development, and full entitlement which is based on the full use of existing entitlements, which represents the maximum possible hydrological change under current rules. Gauging station 417205A is nearest to the sites used in this study.
Discussion
The age of sediment deposited since European occupation
Our results indicated rapid accumulation of fine sediments in Moonie River waterholes since the 1950s. Underlying the fine sediments is a basement layer of hard clays, sand and gravel, which we interpret as representing the pre-European colonisation river bed. The 2008 Kooroon core contained over 2 m of sediment accumulated since the 1950s, with the upper 1.2 m being accumulated since 2003 CE, at the latest. In the 2011 Kooroon core, the oldest definitive post-colonisation age (from 137Cs) was 1955 CE at 105–111-cm depth and, hence, the net minimum sedimentation rate since the mid-1950s was 2.0 cm year−1. In the 2011 Verena core, a minimum of 69 cm of fine sediment has accumulated since 1955 and at least 128 cm of sediment has accumulated in the past 150 years. The hydrology of the study sites was largely unaffected by upstream water abstraction. Hence, the increased accumulation of fine sediments in waterholes since the 1950s is likely to be a result of increased sediment supply, rather than reduced scour and transport capacity owing to water extraction. In the bulk of our dated cores, 14C ages were older than the 210Pbex- and 137Cs-derived ages from equivalent or lower depths. This result is likely to reflect the potential for floods to transport old organic matter from the catchment to waterholes (Reid et al. 2017).
The nature and pattern of sedimentation
The sedimentology of waterhole deposits is characterised by intercalated fine (clay and silt) and coarser (silty sand and sandy silt) laminae and beds (Fig. 6), which suggests that they are the result of multiple episodes of deposition. This interpretation is supported by the magnetic susceptibility profiles from all sites, the variability of diatom stratigraphy from the 2008 Kooroon waterhole (Fig. S1–S6), and the variable nature of the radionuclide profiles (Tables S3, S4) and indicates that sediment has accumulated in the waterholes in episodic fashion from multiple depositional events. Knowing whether the accretion of sediments within waterholes is dominated by large scour and fill-in events (with the sedimentary record reflecting deposition from a single event) or through a more complex, prolonged aggradation from multiple events, as we interpret in this study, is useful for understanding of the controls on waterhole formation and sedimentation processes and in determining whether these waterholes are in equilibrium with present conditions. We interpret that the Moonie River waterholes are not self-maintaining scour features in equilibrium with present conditions (such as interpreted for Cooper Creek waterholes; Knighton and Nanson 2000), given their heterogeneity, lithofacies indicating multiple pulses of deposition, and net deposition of 67–111 cm since the 1950s. We anticipate that where waterholes are contemporary self-maintaining features, in-channel deposits would have a younger age profile and be characterised by substantially less heterogeneity. It may be that these waterholes were self-sustaining scour features before European occupation and probably associated with local increases in stream power. Similar waterholes were observed in nearby systems (the Balonne, Maranoa and Warrego) by Thomas Mitchell, the first European explorer in the northern MDB (Mitchell 1848).
The cores from all three waterholes studied here contain marked variations in composition in terms of siliclastic particle size, sorting, and organic content. The presence of some clean sand (fine to coarse sand-sized clasts) beds indicates reasonably high velocity flows and deposition from bedload transport. The occurrence of sand-beds with a high organic component, comprising organic fragments and twigs, is interpreted to be the result of initial deposition during a high-flow event, or a first pulse of sediment input from local runoff, bringing with it organic debris from upstream channel and tributary or gully beds and adjacent floodplain. The orange-stained laminae are interpreted to be the result of oxidation, indicating a period of waterhole drying. Additional evidence for waterhole drying are the two interpreted sand-filled desiccation cracks in cores Kooroon Site 4 2010 and Kooroon Site 3 2011.
The presence of thin clay beds, which are interpreted to be pure clay and not containing silt or sand-sized clay aggregate particles (slippery and non-gritty texture), indicates clay deposition from suspension, most likely to occur from ponded water after cessation of flow. In many of the cores, these clay beds occur in an interval, through which there are interbedded clay then silty clay layers. These are interpreted to reflect fluctuation between periods of flow that brought in the silts to the waterhole, followed by periods with no flow when the clay layers deposited. If these are the result of local runoff after discrete rainfall events or discharge inflow from upstream, this could reflect deposition over a period of days to weeks to months. However, it is important to note here that the transport and dynamics of fine particles (silt and clay) in fluvial systems is not well understood. Much of the fine-grained suspended load of rivers is cohesive, and this can be transported as flocculated or aggregated particles (Droppo 2001). It is thus not possible to simply extrapolate likely timeframe for clay-bed deposition from particle settling velocities, without measuring the characteristics of fine-particle load in the Moonie River. In addition, it is known that fine particles can be deposited within sand-bed rivers under flow conditions through a variety of mechanisms, such as in localised zones of low-speed fluid, caused by either bedforms or gravel bed roughness, hyporheic exchange owing to the presence of bedforms, filtering of particulates within the bed; and capture by biofilms (e.g. Fries and Taghon 2010; Roche et al. 2017; Mooneyham and Strom 2018). Experiments on mixed clay and sand beds and slurries have shown complex feedback mechanisms among clay concentration, bedform morphodynamics and flow structure (Baas and Best 2002, 2008; Baas et al. 2013). The processes controlling erosion, transport and deposition of fine-grained material within the Moonie River channel bed during flow events is likely to be complex, and would require the sampling of sediment load during a flow event so asr to interpret in more detail.
The variation in 210Pbex and, to a lesser extent 137Cs, with depth in the study sediments is indicative of changes in sediment sources. Where sedimentation is regular and 210Pbex deposition is dominated by atmospheric sources, 210Pbex is expected to decline exponentially with depth. In the absence of such a pattern and in riverine systems where the dominant source of 210Pbex is not atmospheric, the concentration of 210Pbex is an indicator of the depth in the soil profile from which sediment was derived (Wallbrink et al. 1998). In the 2011 Kooroon core record, the maximum 210Pbex concentration was found at a depth of 84–90 cm, and declined towards the surface. In the 2011 Verena core, the pattern was more complex. Near-maximum 210Pbex concentrations occurred in the 92–100- and 128–34-cm samples and then generally declined towards the surface. Usually concentrations of 210Pbex and 137Cs are highest in catchment surface soils and decline deeper in the soil profile. Hence, topsoils are ‘labelled’ with these radionuclides, but they are low or absent in the subsurface (which dominates gully erosion). As a consequence, the declining toward-surface values of 210Pbex in the cores are likely to represent increased proportions of sediment derived from gully or bank erosion depositing in these waterholes in more recent times.
Extent of post-flood scouring
Comparison of sediment-probing results from 2010 to 2011 showed reductions in fine sediment depth following major flooding, but not full scouring of fine sediment deposited since the mid-1950s, and thus incomplete restoration of the waterhole depths to pre-1950s condition. The March 2010 flow event was the largest recorded in the Moonie since flow gauging began in 1954 and was the second-largest event (after an event in 1890) for the catchment, predicted by a daily rainfall-runoff flow simulation model covering 1889–2016 (Department of Environment and Science 2019) (Fig. 2). The second flood in January 2011 was smaller but still represented a large event relative to the gauge record. As we hypothesised, the depth of fine sediment at all sites was reduced following this flooding sequence (Table 1). However, at all sites, but particularly at Kooroon waterhole, substantial amounts of fine sediment deposited after 1955 remained (Table 1). The proportion of the pre-flood soft sediment removed from the deeper parts of each waterhole, the sections which represent the most persistent drought-refuge habitat, increased along a gradient from downstream (Kooroon 25% removed) to upstream (Verena 38%, Kurmala 54%). This scouring resulted in an increase of 0.4–0.6-m water depth at the deepest part of the waterholes (standardised to waterhole cease-to-flow depth) and an increase of ~70–100 days in maximum persistence time across the three waterholes. This represents a noteworthy increase in drought-refuge function. However, despite the benefits, the waterhole maximum depths and persistence times were still far reduced from their pre-1950s capacity, and the post-1950s accumulation of sediment initiated a substantial loss in the benefits of these attributes for refugia function. At Kooroon, in particular, as the most persistent waterhole in the Moonie system, there is a net loss of over 1.5 m of pre-1950 water depth and therefore 260 days of potential drought-refuge function. Climate-change projections, with and without substantial mitigation of greenhouse-gas emissions, raise the possibility that there will be longer and more frequent droughts in the region over the next few decades (Syktus et al. 2020). Indeed, the recent 2018–2020 drought was the longest on record for the region and the Moonie River experienced a record spell with no flow for 660 consecutive days, which was more than double the duration of the previous longest recorded or modelled no-flow spell (Marshall et al. 2021) and was very close to the modelled persistence times of the deepest Moonie waterholes under 2011 sediment levels. Hence, the reduced persistence of waterholes from fine sediment accumulation may be significant to the long-term viability of aquatic species in the Moonie River.
A variety of evidence indicates that sediments in the post-flooding 2011 cores represented material that was deposited sequentially since the 1950s (at the earliest) and retained after flooding, rather than being eroded and replaced with new sediment deposits during flooding. At Site 1 in Kooroon waterhole where cores were collected in both 2010 and 2011, before and after high-flow events, a similar vertical facies association is present, namely, basal silt and clay being interbedded with very fine- to fine-sand laminae, overlain by an interval containing several thin pure clay beds (~1–1.4-m depth in the 2010 core; ~0.95–1.15-m depth in the 2011 core), overlain by the uppermost section of core containing thicker sand beds with a high content of organic clasts and twigs. The thickest sand bed in the 2010 core occurs at 26–49 cm, is a clayey fine sand with large organic fragments associated with sand grains ranging in size between upper fine and lower medium. It is overlain by clay. The thickest sand bed in the 2011 core occurs from 48- to 57-cm depth, and has the same lithology as the upper sand bed in the 2010 core. In contrast, this is overlain by a heterolithic deposit containing two sand beds (at 0–11- and 25–33.5-cm depth) and two clay beds, one with laminae comprising clean very fine sand. Given the similarity in lithology and thickness of the upper thick sand bed in the 2010 and 2011 cores at Karoon Site 1, these could be the same deposit. It is possible that the uppermost mud deposit observed in the 2010 core was eroded during the intervening flow events, and that the uppermost ~40 cm of the 2011 core were the result of one or both of these events. Alternatively, this variability could reflect a high degree of spatial variability in these waterhole sediments.
Thus, it appears that, although a considerable volume of sediment was removed from the waterholes between 2010 and 2011, as outlined above, the bulk of the fine sediment that remained was not the result of a single event from the recent flooding but had been deposited episodically between the late 1950s and 2010. These observations contrast with the model of waterhole scouring developed for dryland rivers in the Lake Eyre Basin, where the existence of waterholes is argued to result from the quasi-regular process of waterhole deepening (Knighton and Nanson 1994). Given that even large floods have not removed the substantial post-colonisation sedimentation, we consider the implications of the continued deposition of large sediment loads on the morphology and refuge function of this river system.
Sedimentation processes
Sediment accumulation in waterholes represents a balance between supply and removal. In many other river systems, there have been changes imposed to both sides of this balance, whereas the near-intact flow regime of the Moonie River provides an opportunity to consider supply-side effects in relative isolation. As the hydrology of the Moonie River in the vicinity, and upstream, of our study waterholes is largely unaltered by water resource development, the accumulation of fine sediments at these sites since the 1950s is interpreted to reflect an increase in sediment supply, rather than a reduction in the competence of the river to scour and transport sediments.
Scouring and transportation of bed sediments is a function of near-bed flow velocity and shear stress. It is generally expected that flow velocity in a waterhole increases with discharge, with a major discontinuity in this trend at bankfull discharge, owing to water lost through spillover onto the floodplain, after which discharge maximum velocity remains constant and mean velocity decreases (Knighton and Nanson 2000, 2002). However, this was not the case at two of three waterholes investigated in Cooper Creek (Knighton and Nanson 2000), nor at some sites in the lower Balonne River system in the northern Murray–Darling Basin (McGregor et al. 2018). These anomalies are probably associated with local variability in the discharge stages at which overbank flow occurred, owing to errors in establishing bankfull discharge in dryland rivers, or because of unstable velocity discharge relationships (Knighton and Nanson 2000).
Information on near-bed flow velocities of northern MDB rivers during floods is sparse, with no research published for the Moonie River. We re-analysed acoustic doppler generated flow-velocity data from the stream gauge closest to our study waterholes (Moonie River at Flinton) collected near the peak of an approximately bankfull flow event on 22 May 2009 at 12:20 hours, when the total discharge was 116.4 m3 s−1 (Queensland Government, unpubl. data). The mid-part of the channel cross-section was near uniform at ~3.8 m deep and ~15 m wide. Near-bed (0 to ~20 cm above the bed) downstream-flow velocity averaged 0.81 m s−1 but was patchy and ranged between 0.07 and 1.87 m s−1 across 110 grid cells. In ideal settings, these velocities are sufficient for the bedload transport of all clast sizes observed in the core sediments, and suspension transport of sand-sized particles (e.g. van Rijn 2020). However, this does not take into consideration factors such as the presence of cohesive particles in transport, nor do we have available data on parameters such as turbulence intensity and bed roughness, to be able to fully evaluate the hydraulics. Given the large amount of net sedimentation in the Moonie River, it is clear that recent sediments (i.e. above basement) have not all been mobilised during this and similar-magnitude or larger events. Given the accumulation of sediments in these waterholes, the episodic nature of sedimentation, and evidence for erosion, the analysis of sediment transport hydraulics at these waterhole sites would be an interesting topic for future investigation, particularly investigation of threshold velocities for erosion of cohesive waterhole sediments, and their subsequent transport morphodynamics and deposition mechanisms.
Inorganic sediment accumulating in the Moonie River waterholes will primarily be a combination of material sourced up-catchment that is in transport as fluvial bed load and suspended load, and local input from rainfall runoff (via overland flow and gullies; Fig. 1). Sediment deposition is likely to occur under different conditions, including from bedload transport and sediment settling out of suspension during flow events, at a range of velocity conditions depending on particle grain size. The thicker sand beds observed in Kooroon waterhole were notably present in the two upstream locations, which could indicate a hydrodynamic control on sediment transport through these waterholes; when sediment input occurs during periods of high water depth in the waterhole and low discharge, such as resulting from local runoff, the reduction in flow velocity on input to the waterhole is likely to result in proximal deposition, and potentially the formation of delta-like features upstream in the waterhole, and near gully mouths. Organic material is likely to be brought into the waterhole both from up-catchment and local runoff events, potentially being deposited along with clastic particles in an initial slurry or debris flow, as bedload or suspension fallout, or settling out through the water column after a flow event is finished. Settling of colloidal clay will occur after flow event has ceased, from still water that is ponded within the waterholes. Some laminae (<1 mm thick) of clean fine sand, orange in colour, were observed in the cores (Kooroon 2011 Site 3 at 157-cm core depth; Kooroon 2011 Site 1 at 114-cm core depth; Kooroon 2011 Site 3 C2 at 90-cm core depth). These could be aeolian-transported sediments, which could be brought into the waterhole during dry periods, either directly onto a dry river bed or settling through the waterhole water column.
Floodplain gully expansion, which supplies sediment to waterholes, occurs in the Moonie catchment both after the recession of floodwater and following local rainfall (Andrew Biggs, pers. comm.). This process has been exacerbated by grazing and de-vegetation of the floodplains and banks (Andrew Biggs, pers. comm.). Studies of other Australian rivers have shown that local sediment erosion and supply through gullying can be significant and is linked to grazing and devegetation (Hughes and Prosser 2012). In semi-arid catchments, sediment load can be supply-limited rather than discharge-limited, and sediment availability can vary on an interannual timescales because of rainfall variability (Amos et al. 2004). As with many Australian dryland rivers, the Moonie Rivers floodplains are characterised by ancient and highly weathered soils with a high content of very fine clay and high sodium concentrations that increase dispersibility (Kirk 1985). During overland flow events, these floodplains generate high suspended sediment loads that raise suspended-sediment concentrations in the river. Because of the colloidal nature of the clay particles, this can cause perpetually elevated turbidity, with values throughout the Moonie River typically ranging from ~300 to 1500 NTU (Queensland Government, unpubl. data). There are many factors involved in colloid stability in natural waters, so it is not possible to accurately predict this phenomenon on theory alone. Experimentation has been recommended (Hahn and Stumm 1970). In an unpublished settlement experiment, the turbidity of water from the Moonie River reduced from 1525 to 1356 NTU in 24 h (Queensland Government, unpubl. data), suggesting that in completely still water, there can be a 10% daily reduction in suspended sediment. However, field observations of stable turbidity over long periods in isolated waterholes suggest that under natural conditions continued colloidal suspension is much more stable, and thus clays may take long periods to settle out of suspension.
Implications of sedimentation in Moonie River waterholes
Recent sedimentation in the Moonie River has led to marked reductions in waterhole depth. Although our study did not determine sedimentation rates before European occupation, it is likely that catchment vegetation clearance has magnified these rates substantially. Thomas Mitchell described many of the region’s rivers as having sandy or gravelly beds (Mitchell 1848); however, today these rivers are characterised by beds of fine sediment. This suggests that the gravel layer intersected at the base of our cores represents the pre-occupation sediments and further supports the notion that sedimentation rates have greatly increased since European occupation. Our study found that there was net sediment accumulation at some sites of up to 2 cm year−1. This accumulation is unlikely to be explained by reductions in flow competence because the proportion of bankfull discharge events was, in fact, higher in the period from 1950 to 2011 than from 1889 to 1950 (Table 3). This sedimentation not only reduced waterhole depth (thereby eliminating potential refuges for aquatic biota during no-flow spells) but also, by differentially greater infilling of previously deeper parts of waterholes, resulted in a reduction in waterhole depth variability, which may have ecological relevance. Pool sedimentation is likely to have adverse effects on fish assemblages across a range of spatial and temporal scales. In individual waterholes, sedimentation degrades refuge quality by reduced habitat complexity. At the whole catchment scale, there is likely to be reduced overall habitat availability and diversity. During droughts, sedimentation-induced loss of waterhole persistence is likely to lead to more local extinctions (reducing population resistance) and, thus, lower capacity for species to repopulate the system following drought (lower resilience).
Flow-management scenario | Period | |
---|---|---|
1889–1949 (22 265 days) | 1950–2011 (22 630 days) | |
Full entitlement | ||
Number of days above bankfull | 66 | 103 |
Proportion of days above bankfull (%) | 0.30 | 0.46 |
Pre-development | ||
Number of days above bankfull | 72 | 115 |
Proportion of days above bankfull (%) | 0.33 | 0.51 |
The Moonie River catchment is one of the most cleared in the MDB and it is likely that this clearance has greatly amplified the mobilisation and delivery of sediment to in-stream waterholes. Reduced persistence times because of deep sediment accumulation at waterholes, particularly Kooroon and Verena, leave them vulnerable to failure during more extreme, future dry spells. Because the waterholes studied are some of the deepest, and therefore most persistent, in the catchment, it is likely that if these sites are affected by sedimentation, the same processes will also shorten the persistence of shallower waterholes. The relatively lower amounts of sediment in Kurmala waterhole than at the other two sites may be due to lower proportion of land clearance and gullying in this part of the catchment, including the fact that the riparian zone is largely fenced around the waterhole. This environmental management challenge is likely to be further amplified further downstream, where no-flow spells are increased as a result of water-resource utilisation (Table 2) and in other parts of the northern Murray–Darling Basin.
There are some key differences in the (albeit limited) literature on in-stream waterholes of arid-zone rivers. It has been interpreted from analysis of topographic and hydrologic data that waterholes of Cooper Creek (Lake Eyre Basin) scour reasonably regularly (Knighton and Nanson 2000), and that their presence and hydraulic geometry are a function of contemporary process controls. In contrast, a study of waterholes in the Lower Balonne River (Murray–Darling Basin) showed that waterhole sediments have persisted for hundreds to thousands of years (Reid et al. 2017). Our results suggest that sediments remain in Moonie River waterholes for decades. Indeed, although there was some scouring following the 2011 floods, much of the sediment deposited following European land clearance remains in the waterholes and has led to marked reductions in the persistence of the deepest waterholes in the system. This may indicate that the Moonie River waterholes are currently infilling and reducing in size, with implications for their longevity as a refuge for biota. Although it is possible that scouring which maintains the cross-sectional area does occur, at multi-decadal or longer intervals, the reductions in depth in the intervening periods may still be sufficient to result in permanent effects on the biota as a result of the loss of refuge function (Marshall et al. 2021).
Our study suggests that substantial scouring of sediment in the Moonie River occurs only after very large flood events (represented in our study by the largest gauged flood). Large flood events in this region occur during summer (Fig. 2) and are predominantly associated with ex-tropical cyclones that penetrate an unusual distance inland, in combination with strong La Niña events (Van den Honert and McAneney 2011). As indicated by the infrequent nature of similarly large-magnitude discharges in the record, such events are rare. In terms of the projected occurrence of future rainfall, there is a wide range in both the direction and magnitude of projected changes in summer rainfall over the 21st century, although declines in the large summer precipitation events are more likely than not (Syktus et al. 2020; Fig. S7). As a result, it seems more probable that there will be fewer potential high-magnitude scour events in the future.
Suggestions for future research
Given the differences in key drivers such as catchment area, slope, discharge regime and geomorphology of the three waterhole study locations on Cooper Creek (Knighton and Nanson 2000), the Lower Balonne (Reid et al. 2017) and Moonie Rivers (this study), it is not surprising that there are differences in their hydraulic, geomorphologic and sedimentologic characteristics. The Cooper Creek study focused on channel geometry and hydrology, without observation of waterhole sediments; in contrast, studies of the Lower Balonne and Moonie River waterholes present descriptions of deposit properties without hydraulic analysis. Future waterhole investigations presenting both deposit properties and hydraulic analysis (across a range of these key drivers) would be of value.
Given our observation of high variability in the depth of deposited sediments within waterholes of the Moonie River presented here, a more structured approach to mapping soft-sediment depth would be beneficial. ‘Water penetrating radar’ has been used for such mapping in other aquatic environments, for example, to provide spatial context to sediment cores and to map sediment deposition within reservoirs (Ruffell and Parker 2021). We suggest that there is potential for similar use in dryland river waterholes.
The application of radiocarbon and 210Pb and 137Cs dating was moderately successful in determining sedimentation rates in the Moonie River system. The method of combining different isotopic markers meant that, depending on the particular circumstance, multiple approaches could be used to determine the most precise age, especially for the post-colonisation sediments. An unfortunate limitation of this method is that the earliest age for these markers is often associated with the commencement of nuclear-weapons testing in the 1950s (represented chemically by the appearance of 137Cs and the 14C ‘bomb spike’). As a result, it is quite possible that sediments attributed to the 1950s are, in fact, considerably younger, but cannot be refined as such without additional information. Our approach could be improved by the application of optically stimulated luminescence (OSL) dating and the use of fallout 239+240Pu as chronomarker (Leslie and Hancock 2008). Additionally, OSL can date sediments with ages ranging from decades to millennia and, therefore, bridge the gap between pre- and post-colonisation sediments. Such approaches could be combined with other techniques to refine sediment dating in the pre-modern period. These include the stratigraphy of stable lead concentrations and stable lead isotopes, which can be related to changes in the production and source of lead deposited in Australian landscapes (Cook and Gale 2005) and, or, using pollen markers specific to introduced plants and land-use changes. The analysis of both in-channel and floodplain sediments would be useful.
Acquisition of a more precise and lengthier chronology would markedly enhance our understanding of the drivers of sedimentation, for example, by allowing specific parts of the stratigraphy to be matched to particular historical events, to climatic drivers or human-induced changes (e.g. Tibby 2003; Rowland et al. 2005; Croke et al. 2011; Donselaar et al. 2022). Perhaps the most useful application of this approach would be to determine the extent to which localised overland flow and gully transport contribute to waterhole sedimentation at times of low or no flow compared with transport during large catchment-scale flow events.
Finally, options for managing sediment oversupply to reduce impacts on dryland river waterhole function should be explored. The source of the sediment contributing to waterhole in-filling within the Moonie system is a critical land management question that remains unanswered. In other rivers in the Murray–Darling Basin, the dominant source of sediment is eroded from subsoils (particularly by gully erosion), rather than topsoils (Wallbrink et al. 1998; Prosser et al. 2001) and this may be the case for the Moonie. In addition, more broadly, specific (sub)catchments can dominate sediment budgets (Wallbrink et al. 1998; Garzon-Garcia et al. 2015; Garzon-Garcia et al. 2017; Dutton et al. 2018). In the Moonie catchment it is not yet possible to determine the origin and spatial distribution of sediments deposited in waterholes. Sediment tracing by using suspended sediment measurement, sediment chemistry and radionuclides (Wallbrink et al. 1998; Prosser et al. 2001; Amos et al. 2009; Dutton et al. 2018) would help identify sediment sources. Source ‘hotspots’ could then be targeted for land rehabilitation to reduce oversupply to the river. Our radionuclide data indicated that the bulk of the sediment delivered to the Moonie in recent times is derived from gully erosion. Owing to the extent of land-use change in the Moonie catchment, measures to reduce sediment supply may also need to be supported by actions to reduce sediment loads in the river. In some rivers, this can be achieved by flow-regime restoration. However, given the near-natural hydrology of the Moonie, this is not a viable option. Sediment could be manually removed with earth-moving equipment, but this may impose other undesirable stressors on the ecosystem. Our consideration of near-bed flow velocities, cursory owing to a dearth of data, could be expanded in further studies to determine variability both within waterholes and at a variety of flow stages, across a variety of waterhole morphologies. This could be combined with study of the hydraulics of Moonie River waterholes, such as nature of sediment bed (compactness, particle size distribution, roughness), and cross-sectional geometries, to enable hydraulic modelling and estimation of critical velocities for sediment erosion and transport. In combination, these would allow predictions to be made of hydraulic conditions and sediment transportation potentials, which remain uncertain. With such information, there is potential to design structures that focus flow energy and enhance scour to deepen key waterholes (e.g. Rosgen 2001).
Conclusions
In the deepest waterholes in the Moonie River system, large amounts of sediment have accumulated since the mid-20th century. This accumulation is likely to be related to an increase in sedimentation rates resulting from colonial catchment-land clearance and grazing on river banks and adjacent floodplains. Despite the recent occurrence of some of the largest flood events in the system’s recorded history, substantial amounts of recently deposited fine sediment remain in these waterholes. The deposition of this sediment has reduced the modelled persistence of the deepest waterhole in the system from 861 to 600 days, which was the approximate duration of the record consecutive days without flow during the recent 2018–2020 drought. Given that there is reasonably little water extraction from the Moonie River (e.g. for irrigated agriculture), sedimentation, along with the likely intensification of drought under climate change, represent the greatest threats to the persistence of refugia in the system. Future research should focus on identifying the source of sediments entering these waterholes as a basis for prioritising erosion control measures. Given that large flood events have scoured some deposited sediment from the waterholes, thereby extending their persistence, it is important that future water resource development does not reduce the occurrence of such bankfull and greater flows.
Data availability
Radionuclide data are available in the supplementary material. Diatom and magnetic susceptibility data will be available on the University of Adelaide’s online data repository (https://doi.org/10.25909/23512053)
Acknowledgements
We acknowledge the Bigambul people, Traditional Owners and Custodians of the land on which this research was conducted. We thank Melanie Shaw, Joanna Blessing and Peter Negus for assistance with field work, Hossain Siddiqui for assistance in the preparation and submission of sediments for dating, and Tessa Lane for drawing the sediment logs. Queensland government agency staff collected various types of unpublished data used in our study to support its conclusions.
References
Amos KJ, Alexander J, Horn A, Pocock GD, Fielding CR (2004) Supply limited sediment transport in a high-discharge event of the tropical Burdekin River, North Queensland, Australia. Sedimentology 51(1), 145-162.
| Crossref | Google Scholar |
Amos KJ, Croke JC, Timmers H, Owens PN, Thompson C (2009) The application of caesium-137 measurements to investigate floodplain deposition in a large semi-arid catchment in Queensland, Australia: a low-fallout environment. Earth Surface Processes and Landforms 34(4), 515-529.
| Crossref | Google Scholar |
Arthington AH, Balcombe SR, Wilson GA, Thoms MC, Marshall J (2005) Spatial and temporal variation in fish-assemblage structure in isolated waterholes during the 2001 dry season of an arid-zone floodplain river, Cooper Creek, Australia. Marine and Freshwater Research 56(1), 25-35.
| Crossref | Google Scholar |
Australian Institute of Aboriginal and Torres Strait Islander Studies (1996) AIATSIS map of Indigenous Australia. Available at https://aiatsis.gov.au/explore/articles/aiatsis-map-indigenous-australia [Verified 1 March 2019]
Baas JH, Best JL (2002) Turbulence modulation in clay-rich sediment-laden flows and some implications for sediment deposition. Journal of Sedimentary Research 72(3), 336-340.
| Crossref | Google Scholar |
Baas JH, Best JL (2008) The dynamics of turbulent, transitional and laminar clay-laden flow over a fixed current ripple. Sedimentology 55(3), 635-666.
| Crossref | Google Scholar |
Baas JH, Davies AG, Malarkey J (2013) Bedform development in mixed sand–mud: the contrasting role of cohesive forces in flow and bed. Geomorphology 182(15), 19-32.
| Crossref | Google Scholar |
Barr C, Tibby J, Marshall JC, McGregor GB, Moss PT, Halverson GP, Fluin J (2013) Combining monitoring, models and palaeolimnology to assess ecosystem response to environmental change at monthly to millennial timescales: the stability of Blue Lake, North Stradbroke Island, Australia. Freshwater Biology 58(8), 1614-1630.
| Crossref | Google Scholar |
Bond NR, Balcombe SR, Crook DA, Marshall JC, Menke N, Lobegeiger JS (2015) Fish population persistence in hydrologically variable landscapes. Ecological Applications 25(4), 901-913.
| Crossref | Google Scholar |
Bunn SE, Thoms MC, Hamilton SK, Capon SJ (2006) Flow variability in dryland rivers: boom, bust and the bits in between. River Research and Applications 22(2), 179-186.
| Crossref | Google Scholar |
Carini G, Hughes JM, Bunn SE (2006) The role of waterholes as ‘refugia’ in sustaining genetic diversity and variation of two freshwater species in dryland river systems (Western Queensland, Australia). Freshwater Biology 51(8), 1434-1446.
| Crossref | Google Scholar |
Chambers JW, Cameron NG (2001) A rod-less piston corer for lake sediments: an improved, rope-operated percussion corer. Journal of Paleolimnology 25(1), 117-122.
| Crossref | Google Scholar |
Constantine CR, Mount JF, Florsheim JL (2003) The effects of longitudinal differences in gravel mobility on the downstream fining pattern in the Cosumnes River, California. The Journal of Geology 111(2), 233-241.
| Crossref | Google Scholar |
Cook DE, Gale SJ (2005) The curious case of the date of introduction of leaded fuel to Australia: implications for the history of southern hemisphere atmospheric lead pollution. Atmospheric Environment 39, 2553-2557.
| Crossref | Google Scholar |
Cremer H, Wagner B, Melles M, Hubberten H-W (2001) The postglacial environmental development of Raffles So, East Greenland: inferences from a 10,000 year diatom record. Journal of Paleolimnology 26(1), 67-87.
| Crossref | Google Scholar |
Croke J, Jansen JD, Amos K, Pietsch TJ (2011) A 100 ka record of fluvial activity in the Fitzroy River Basin, tropical northeastern Australia. Quaternary Science Reviews 30(13–14), 1681-1695.
| Crossref | Google Scholar |
Donselaar ME, Cuevas Gozalo MC, van Toorenenburg KA, Wallinga J (2022) Spatio-temporal reconstruction of avulsion history at the terminus of a modern dryland river system. Earth Surface Processes and Landforms 47(5), 1212-1228.
| Crossref | Google Scholar |
Droppo IG (2001) Rethinking what constitutes suspended sediment. Hydrological Processes 15(9), 1551-1564.
| Crossref | Google Scholar |
Dutton CL, Subalusky AL, Anisfeld SC, Njoroge L, Rosi EJ, Post DM (2018) The influence of a semi-arid sub-catchment on suspended sediments in the Mara River, Kenya. PLoS ONE 13(2), e0192828.
| Crossref | Google Scholar |
Fries JS, Taghon GL (2010) Particle fluxes into permeable sediments: comparison of mechanisms mediating deposition. Journal of Hydraulic Engineering 136(4), 214-221.
| Google Scholar |
Gale SJ, Haworth RJ (2002) Beyond the Limits of Location: human environmental disturbance prior to official European contact in early colonial Australia. Archaeology in Oceania 37, 123-136.
| Crossref | Google Scholar |
Garzon-Garcia A, Olley JM, Bunn SE (2015) Controls on carbon and nitrogen export in an eroding catchment of south-eastern Queensland, Australia. Hydrological Processes 29(5), 739-751.
| Crossref | Google Scholar |
Garzon-Garcia A, Laceby JP, Olley JM, Bunn SE (2017) Differentiating the sources of fine sediment, organic matter and nitrogen in a subtropical Australian catchment. Science of The Total Environment 575, 1384-1394.
| Crossref | Google Scholar |
Gell P, Tibby J, Fluin J, Leahy P, Reid M, Adamson K, Bulpin S, MacGregor A, Wallbrink P, Hancock G, Walsh B (2005) Accessing limnological change and variability using fossil diatom assemblages, south-east Australia. River Research and Applications 21(2-3), 257-269.
| Crossref | Google Scholar |
Hahn HH, Stumm W (1970) The role of coagulation in natural waters. American Journal of Science 268(4), 354-368.
| Crossref | Google Scholar |
Hamilton SK, Bunn SE, Thoms MC, Marshall JC (2005) Persistence of aquatic refugia between flow pulses in a dryland river system (Cooper Creek, Australia). Limnology and Oceanography 50(3), 743-754.
| Crossref | Google Scholar |
Hogg AG, Heaton TJ, Hua Q, Palmer JG, Turney CSM, Southon J, Bayliss A, Blackwell PG, Boswijk G, Bronk Ramsey C, Pearson C, Petchey F, Reimer P, Reimer R, Wacker L (2020) SHCal20 Southern Hemisphere Calibration, 0–55,000 years cal BP. Radiocarbon 62(4), 759-778.
| Crossref | Google Scholar |
Hua Q, Turnbull JC, Santos GM, Rakowski AZ, Ancapichún S, De Pol-Holz R, Hammer S, Lehman SJ, Levin I, Miller JB, Palmer JG, Turney CSM (2022) Atmospheric radiocarbon for the period 1950–2019. Radiocarbon 64(4), 723-745.
| Crossref | Google Scholar |
Huey JA, Schmidt DJ, Balcombe SR, Marshall JC, Hughes JM (2011) High gene flow and metapopulation dynamics detected for three species in a dryland river system. Freshwater Biology 56(11), 2378-2390.
| Crossref | Google Scholar |
Hughes AO, Prosser IP (2012) Gully erosion prediction across a large region: Murray–Darling Basin, Australia. Soil Research 50(4), 267-277.
| Crossref | Google Scholar |
Kirk JTO (1985) Effects of suspensoids (turbidity) on penetration of solar radiation in aquatic ecosystems. Hydrobiologia 125(1), 195-208.
| Crossref | Google Scholar |
Knighton AD, Nanson GC (1994) Waterholes and their significance in the anastomosing channel system of Cooper Creek, Australia. Geomorphology 9(4), 311-324.
| Crossref | Google Scholar |
Knighton AD, Nanson GC (2000) Waterhole form and process in the anastomosing channel system of Cooper Creek, Australia. Geomorphology 35(1), 101-117.
| Crossref | Google Scholar |
Knighton AD, Nanson GC (2002) Inbank and overbank velocity conditions in an arid zone anastomosing river. Hydrological Processes 16(9), 1771-1791.
| Crossref | Google Scholar |
Leslie C, Hancock GJ (2008) Estimating the date corresponding to the horizon of the first detection of 137Cs and 239+240Pu in sediment cores. Journal of Environmental Radioactivity 99(3), 483-490.
| Crossref | Google Scholar |
Livingstone DA (1955) A lightweight piston sampler for lake deposits. Ecology 36(1), 137-139.
| Crossref | Google Scholar |
Magoulick DD (2000) Spatial and temporal variation in fish assemblages of drying stream pools: the role of abiotic and biotic factors. Aquatic Ecology 34(1), 29-41.
| Crossref | Google Scholar |
Marshall JC, Menke N, Crook DA, Lobegeiger JS, Balcombe SR, Huey JA, Fawcett JH, Bond NR, Starkey AH, Sternberg D, Linke S, Arthington AH (2016) Go with the flow: the movement behaviour of fish from isolated waterhole refugia during connecting flow events in an intermittent dryland river. Freshwater Biology 61(8), 1242-1258.
| Crossref | Google Scholar |
Marshall JC, Lobegeiger JS, Starkey A (2021) Risks to fish populations in dryland rivers from the combined threats of drought and instream barriers. Frontiers in Environmental Science 9, 671556.
| Crossref | Google Scholar |
McGregor GB, Marshall JC, Thoms MC (2006) Spatial and temporal variation in algal-assemblage structure in isolated dryland river waterholes, Cooper Creek and Warrego River, Australia. Marine and Freshwater Research 57(4), 453-466.
| Crossref | Google Scholar |
Mooneyham C, Strom K (2018) Deposition of suspended clay to open and sand-filled framework gravel beds in a laboratory flume. Water Resources Research 54(1), 323-344.
| Crossref | Google Scholar |
Morón S, Amos KJ (2018) Downstream grain-size changes associated with a transition from single channel to anabranching. Sedimentology 65(5), 1590-1610.
| Crossref | Google Scholar |
Murray–Darling Basin Authority (2021) Moonie. (MDBA) Available at https://www.mdba.gov.au/water-management/catchments/moonie [Verified 6 January 2023]
Negus P, Blessing J, Steward A, Clifford S, Hansen D, Hammill B (2015b) Ecological risk assessment and threat prioritisation in Queensland’s Eastern Murray Darling Rivers: Condamine, Balonne and Maranoa; Lower Balonne; Moonie; and Border Rivers, 2012. Q–Catchments Program. Department of Science Information Technology and Innovation, Queensland Government, Brisbane, Qld, Australia.
O’Sullivan T, AM DJM, Kamenar A, Brown RS (1991) The discovery and development of Moonie – Australia’s first commercial oilfield. The APPEA Journal 31(1), 1-12.
| Crossref | Google Scholar |
Pearson MR, Reid MA, Miller C, Ryder D (2020) Comparison of historical and modern river surveys reveal changes to waterhole characteristics in an Australian dryland river. Geomorphology 356, 107089.
| Crossref | Google Scholar |
Prosser IP, Rutherfurd ID, Olley JM, Young WJ, Wallbrink PJ, Moran CJ (2001) Large-scale patterns of erosion and sediment transport in river networks, with examples from Australia. Marine and Freshwater Research 52(1), 81-99.
| Crossref | Google Scholar |
Queensland Government (2021) 2018–19 SLATS report. Available at https://www.qld.gov.au/environment/land/management/mapping/statewide-monitoring/slats/slats-reports/2018-19-report [Verified 19 January 2023]
Reid M, Fluin J, Ogden R, Tibby J, Kershaw P (2002) Long-term perspectives on human impacts on floodplain–river ecosystems, Murray–Darling Basin, Australia. SIL Proceedings, 1922-2010 28(2), 710-716.
| Crossref | Google Scholar |
Reid MA, Thoms MC, Chilcott S, Fitzsimmons K (2017) Sedimentation in dryland river waterholes: a threat to aquatic refugia? Marine and Freshwater Research 68(4), 668.
| Crossref | Google Scholar |
Rengers F, Wohl E (2007) Trends of grain sizes on gravel bars in the Rio Chagres, Panama. Geomorphology 83(3), 282-293.
| Crossref | Google Scholar |
Reside AE, Beher J, Cosgrove AJ, Evans MC, Seabrook L, Silcock JL, Wenger AS, Maron M (2017) Ecological consequences of land clearing and policy reform in Queensland. Pacific Conservation Biology 23(3), 219-230.
| Crossref | Google Scholar |
Roche KR, Drummond JD, Boano F, Packman AI, Battin TJ, Hunter WR (2017) Benthic biofilm controls on fine particle dynamics in streams. Water Resources Research 53(1), 222-236.
| Crossref | Google Scholar |
Rosgen DL (2001) The Cross-Vane, W-Weir and J-Hook Vane structures…their description, design and application for stream stabilization and river restoration. In ‘Wetlands Engineering and River Restoration 2001’, 27–31 August 2001, Reno, NV, USA. pp. 1–22. (American Society of Civil Engineers) doi:10.1061/40581(2001)72
Rowland JC, Lepper K, Dietrich WE, Wilson CJ, Sheldon R (2005) Tie channel sedimentation rates, oxbow formation age and channel migration rate from optically stimulated luminescence (OSL) analysis of floodplain deposits. Earth Surface Processes and Landforms 30(9), 1161-1179.
| Crossref | Google Scholar |
Ruffell A, Parker R (2021) Water penetrating radar. Journal of Hydrology 597, 126300.
| Crossref | Google Scholar |
Sheldon F, Bunn SE, Hughes JM, Arthington AH, Balcombe SR, Fellows CS (2010) Ecological roles and threats to aquatic refugia in arid landscapes: dryland river waterholes. Marine and Freshwater Research 61(8), 885-895.
| Crossref | Google Scholar |
Sternberg D, Balcombe S, Marshall J, Lobegeiger J (2008) Food resource variability in an Australian dryland river: evidence from the diet of two generalist native fish species. Marine and Freshwater Research 59(2), 137-144.
| Crossref | Google Scholar |
Syktus J, Trancoso R, Ahrens D, Toombs N, Wong K (2020) The Long Paddock: Queensland future climate. (The State of Queensland) Available at https://www.longpaddock.qld.gov.au/qld-future-climate [Verified 14 June 2023]
The State of Queensland (2019) Water Act 2000. Water Plan (Border Rivers and Moonie) 2019. Australia. Available at https://www.legislation.qld.gov.au/view/pdf/2019-02-22/sl-2019-0012
Thoms MC, Ogden RW, Reid MA (1999) Establishing the condition of lowland floodplain rivers: a palaeo-ecological approach. Freshwater Biology 41, 407-423.
| Crossref | Google Scholar |
Tibby J (2003) Explaining lake and catchment change using sediment derived and written histories: an Australian perspective. Science of The Total Environment 310(1-3), 61-71.
| Crossref | Google Scholar |
Tibby J, Gell P, Hancock G, Clark M (2010) Complex reservoir sedimentation revealed by an unusual combination of sediment records, Kangaroo Creek Reservoir, South Australia. Journal of Paleolimnology 43(3), 535-549.
| Crossref | Google Scholar |
Tibby J, Barr C, Marshall JC, Richards J, Perna C, Fluin J, Cadd HR (2019) Assessing the relative impacts of land-use change and river regulation on Burdekin River (Australia) floodplain wetlands. Aquatic Conservation: Marine and Freshwater Ecosystems 29(10), 1712-1725.
| Crossref | Google Scholar |
Van den Honert RC, McAneney J (2011) The 2011 Brisbane floods: causes, impacts and implications. Water 3(4), 1149-1173.
| Crossref | Google Scholar |
van Rijn LC (2020) Literature review of critical bed-shear stresses for mud–sand mixtures. Available at https://www.leovanrijn-sediment.com/papers/Threshsandmud2020.pdf [Verified 14 June 2023]
Wakelin-King GA (2022) Landscapes of the Lake Eyre Basin: the catchment-scale context that creates fluvial diversity. Transactions of the Royal Society of South Australia 146(1), 109-167.
| Crossref | Google Scholar |
Wallace J, Waltham N, Burrows D, McJannet D (2015) The temperature regimes of dry-season waterholes in tropical northern Australia: potential effects on fish refugia. Freshwater Science 34(2), 663-678.
| Crossref | Google Scholar |
Wallbrink PJ, Murray AS, Olley JM, Olive LJ (1998) Determining sources and transit times of suspended sediment in the Murrumbidgee River, New South Wales, Australia, using fallout 137Cs and 210Pb. Water Resources Research 34(4), 879-887.
| Crossref | Google Scholar |