The implications of brief floodplain inundation for local and landscape-scale ecosystem function in an intermittent Australian river
Oliver P. Pratt




A
Abstract
Floodplain inundation creates a diversity of aquatic habitats that diverge in their physical, chemical and biological characteristics through space and time, influencing site-scale ecological processes, with implications for local and landscape-scale ecosystem functioning.
In this study, we characterise conditions of pools on the floodplain and pools in the main channel of the Fitzroy River, north-western Australia.
We used linear models to investigate the spatial and temporal dynamics of top–down and bottom–up forces acting on phytoplankton and zooplankton.
Floodplain pools showed considerable heterogeneity compared with the main channel and were shallow and turbid with high nutrient loads, whereas main-channel pools were deep, clear and nutrient-limited. Phytoplankton and zooplankton biomass (mass per unit volume) were considerably greater and more variable in floodplain pools than in the main channel, where both were largely absent. On the floodplain, bottom–up processes drove water-column productivity (e.g. nutrients to phytoplankton to zooplankton) to a degree not observed in the main channel, providing a valuable resource pathway supporting consumer populations locally and catchment wide. We detected no top–down effects in floodplain pools and no top–down or bottom–up effects in the main channel.
Maintaining flows that inundate the floodplain and promote habitat heterogeneity in the Fitzroy River is crucial for preserving local and landscape-scale ecosystem functioning.
Water managers should take into account the important contribution of floodplain pools to the wider riverine ecosystem and ensure these habitats are not unduely affected by water resource development.
Keywords: bottom–up, consumer control, Fitzroy River, floodplain, Martuwarra, northern Australia, phytoplankton, primary production, resource control, secondary production, top–down, zooplankton.
Introduction
The natural flow regime of a river has been described as the ‘master variable’, where the magnitude, timing, predictability and duration of flow underpin ecological integrity across the riverine landscape (Poff et al. 1997). On the floodplain, this natural hydrological variability through space and time interacts with geomorphology, promoting habitat heterogeneity (Junk et al. 1989; Poff and Ward 1990; Poff et al. 1997; Amoros and Bornette 2002), where local environmental conditions drive ecosystem structure and functioning (Thorp et al. 2006; Warfe et al. 2011; Rolls et al. 2012). This is particularly evident in systems with discrete ‘wet’ and ‘dry’ seasons, such as those in the tropics, where seasonal extremes of water availability across the landscape influence ecological processes in line with the prevailing physical and chemical conditions (Junk et al. 1989; Lewis et al. 2000; Winemiller et al. 2014; Feitosa et al. 2019).
In the tropics, seasonal flooding during the wet season drives patterns of floodplain productivity, where terrestrial nutrient subsidies, warm water temperatures and high light availability stimulate primary productivity with flow-on effects to higher trophic levels (Junk et al. 1989; Lewis et al. 2000; Pettit et al. 2011, 2017). However, there is an assumption that only large flood events with long water-residence periods provide a significant contribution to both local and landscape-scale ecosystem functioning (Junk et al. 1989). Indeed, much of the relevant literature pertains to systems with vast floodplains, where inundation may persist for several months (Junk and Furch 1993; Sparks 1995; Thomaz et al. 2007; Pettit et al. 2011; Molinari et al. 2021; Mosepele et al. 2022). There is comparatively limited information regarding rivers that exhibit brief floodplain inundation, where floodplain waterbodies isolate quickly from the main river channel and reconnect only the following wet season. Many of these floodplain habitats are ephemeral or semi-permanent, and pool persistence is generally dependent on the timing, magnitude and spatial extent of wet-season flood flows.
Local environmental conditions drive ecosystem structure and functioning (Thorp et al. 2006; Warfe et al. 2011; Rolls et al. 2012). Where physical, chemical and biological characteristics differ among habitats, it is likely that ecological processes, such as predation and primary production, vary in strength also. The relative influence of these fundamental ecological processes can be inferred by assessing the strength of consumer regulation of food resources (top–down forces) relative to resource limitation (bottom–up forces). Top–down and bottom–up forces can influence food-web structure (Koning and McIntyre 2021), biotic community structure (das Candeias et al. 2022) and ecological functioning (Power 1990) in riverine ecosystems. In floodplain rivers of the neo-tropics, bottom–up forces predominate (Trevisan and Forsberg 2007; Rejas and Muylaert 2010; Frau et al. 2019), although top–down control can vary in strength through space and time as low-water conditions increase predator densities (Winemiller et al. 2006, 2014). The literature examining top–down and bottom–up processes across northern Australia show a similar pattern (Garcia et al. 2015; Turschwell et al. 2019), although much of this research pertains to the main river channel and often with a focus on benthic algae in recognition of the importance of this resource in food webs across the wet–dry tropics (Douglas et al. 2005; Jardine et al. 2012a; Pettit et al. 2017). However, in a recent review Burrows et al. (2022) concluded that other energy sources may provide a significant contribution to productivity, depending on the stage of an individual’s lifecycle, hydrological period, or habitat. Indeed, there is strong evidence that the phytoplankton resource pathway, from zooplankton, is important for many fish species found in northern and central Australia (Medeiros and Arthington 2008; Pusey et al. 2021; Pratt et al. 2023). Moreover, zooplankton are typically the main food item consumed by most fish species during the larval life-stage (Nunn et al. 2012). Zooplankton are often highly abundant in floodplain pools relative to the main river channel (Godfrey et al. 2021; Pratt et al. 2023) and thus may provide an important food resource in these habitats.
In the Fitzroy River, located in the wet–dry tropical region of north-western Australia, floodplain inundation typically lasts for days to several weeks (Jardine et al. 2012a). This contrasts with other large rivers across northern Australia where water may remain on the floodplain for several months, for example, the East Alligator River, up to 6 months, the Daly River, up to 4 months, and the Mitchell river, up to 2 months (Pettit et al. 2011; Jardine et al. 2012a). Currently, there is limited knowledge of the physical, chemical and biological characteristics of floodplain pools across northern Australia in general, and no thorough characterisation of these habitats has been conducted in the Fitzroy River. Existing research regarding floodplain pools in the Fitzroy River has primarily focussed on fish (Jardine et al. 2012a; Beesley et al. 2020; Lear et al. 2023; Pratt et al. 2023) and macroinvertebrates (Beesley et al. 2023); thus, knowledge of their contribution to local and landscape-scale ecosystem functioning is incomplete. These habitats are under threat from water-resource development and information regarding the role these habitats play in the wider riverine landscape can help craft water planning policy to maintain ecological integrity across the catchment.
In this study, we characterise the physical, chemical and biological conditions of floodplain waterbodies and the main channel of the Fitzroy River. We also examine the spatial and temporal dynamics of top–down and bottom–up processes influencing a primary producer (phytoplankton) and a primary consumer (zooplankton). The Fitzroy River is an ideal system to conduct this study because floodplain inundation is typically brief, meaning floodplain pools isolate quickly from each other and the main channel, providing spatially distinct habitat types that diverge in their physical and chemical character over time. We hypothesise that (1) bottom–up processes will dominate in both main-channel and floodplain pools, as is typical in other tropical rivers, and that (2) top–down forces acting on zooplankton (fish predation) will be strong in floodplain pools, owing to the abundance of zooplanktivorous fish. Finally, we expect (3) the relative strength of top–down forces on the floodplain to increase over time as the dry season progresses and consumer densities increase. Understanding the dynamics of fundamental ecological processes at a local and landscape scale can assist managers when crafting water planning policy, protecting aspects of the natural flow regime that promote habitat heterogeneity and maintain ecosystem functioning across the catchment.
Materials and methods
Study site
The Fitzroy River is located in a savanna landscape in the wet–dry tropical region of north-western Australia (Fig. 1). The river is set within a catchment of 94,000 km2, with a main channel ~700 km long and mean annual discharge of 6600 GL (Petheram et al. 2018a). The lower ~350 km of the river, downstream of Geikie Gorge (Fig. 1), is characterised by a broad flat floodplain and wide meandering main channel with occasional anabranches (Taylor 1999), contrasting with the narrow channel and marginal floodplain associated with steep-sided gorges typical in the upper reaches. The distinct wet (November–April) and dry (May–November) seasons are predictable (Bureau of Meteorology 2024) and marked by significant hydrological variability leading to extreme periods of water availability (both high and low) across the catchment. Inter-annual variability in flow is high and the river is classified as ‘wet-season highly intermittent’ by Kennard et al. (2010). Wet-season flows connect main channel pools and backfill floodplain distributary creeks, facilitating ecological exchange between main channel and floodplain habitats. Overbank inundation of the floodplain is typically brief (days to weeks), in contrast to many other large rivers in the wet–dry tropics where water may remain on the floodplain for up to several months (Jardine et al. 2012a). During the dry season, the main channel disaggregates into a series of elongated pools separated by shallow runs. Deep water creek systems on or adjacent to the floodplain, but separate from the main channel, hold water year round (Lear et al. 2023). Shallow-water (<2 m), semi-permanent floodplain pools formed in topographic depressions and distributary creeks create a mosaic across the landscape and contract under drying conditions. Flow in the river is mostly unmodified with the main regulatory feature a 3-m-high barrage at Camballin (Fig. 1). However, there is potential for water-resource development to support irrigated agriculture in the lower river catchment (Petheram et al. 2018b). If development proceeds, there is a possibility of diverting water from distributary creeks into off-stream storage facilities, an action that could decrease hydrological connectivity between the river and its floodplain (Karim et al. 2018).
Study design and sampling methods
Sampling was conducted in the lowland reaches of the river where the floodplain is most extensive. Sampling occurred in the dry season of 2020 and 2021 and targeted two macro-habitat types, namely, main-channel pools and floodplain pools, the latter being ephemeral or semi-permanent. Logistical constraints resulted in an unbalanced sample design. In total, 31 pools were sampled, 11 in the main-channel and 20 on the floodplain. All main-channel pools were sampled during the late dry season, 5 in October 2020 and 6 in September 2021. Floodplain pools were categorised into meso-habitats on the basis of their formation processes; ‘pond-form pools’ (n = 14 sites) formed through pooling processes in topographic depressions, and ‘channel-form pools’ (n = 6 sites) created by fluvial mechanisms. Twelve floodplain pools (n = 8 pond form and n = 4 channel form) were sampled in the late dry season in October 2020 and eight (n = 7 pond form and n = 1 channel form) in the early dry season in June 2021.
We characterised the physical and chemical condition of floodplain and main channel pools to contextualise any observed differences in biological communities and ecological processes. Physical and chemical data were collected at all 20 floodplain pools but only at 6 of 11 main-channel pools. Environmental and water-quality data were collected prior to any biological sampling to avoid site disturbance. We measured dissolved oxygen (mg L−1), pH, electrical conductivity (μs cm−1), salinity (total dissolved ions calculated from conductivity and temperature data, ppt) and turbidity (NTU) at a depth of ~15 cm by using a YSI ProDSS (Xylem, USA) at three locations within each site. The mean value was used in statistical analysis. One water sample was collected from the approximate centre of each site at a depth of 15 cm for nutrient analysis. Samples were passed through a 0.45-μm membrane and frozen immediately for subsequent measurement of dissolved inorganic nitrogen (NH4-N + NOx-N, μg L−1) and phosphorus (PO4-P, μg L−1), which represent the primary nutrients available to phytoplankton (Reynolds 2006). Nutrient analysis followed standard methods (American Public Health Association et al. 2012). Mean water depth at each site was estimated from a series of point measurements (13–45 per site) along transects (2–5 per site). The number of transects increased with an increasing depth heterogeneity. We estimated available habitat by visual assessment of substrate type (%), large woody debris (%), small woody debris (%) and macrophytes (%). These data, combined with water depth, were used to create a relative metric of habitat complexity modified from Beesley (2006).
We collected data describing the biological characteristics of floodplain and main-channel habitats. Sampling occurred in all 20 floodplain pools and 10 of 11 main-channel pools. Phytoplankton biomass in the water column was estimated from chlorophyll-a (Chl-a) concentration (mg m−3) in seston (particulate material in suspension) (Webster et al. 2005). Water samples from each site were collected at a depth of 30 cm and a subsample of known volume was filtered through GF/F glass-fibre filters (Whatman Ltd, Kent, UK), kept in the dark and frozen until fluorometric determination of Chl-a concentration as per American Public Health Association et al. (2012). Zooplankton were collected using a plankton tow net with a mesh size of 100 μm. Three replicate water samples, each 40 L in volume, were collected at a depth of 30 cm and poured through the net using a 5-L measuring jug (eight per replicate sample). Trapped seston was rinsed down the sides of the net into a 50-mL vial and preserved with 70% ethanol. Fish assemblages were sampled in all floodplain pools but not the main channel because of saltwater crocodile risk. Sampling was conducted with a 7-m-long seine net with a 1-m drop and 2-mm mesh so that smaller-bodied fish were captured. To ensure that fish assemblages were sampled with adequate accuracy and precision, sampling effort, i.e. number of seine-net drags, varied at each site depending on pool size and habitat complexity. Large pools with high habitat complexity required a greater sampling effort to maximise capture probability. All fish collected were identified to species level and released at the approximate point of capture.
Laboratory methods
In the laboratory, zooplankton samples were stained with rose bengal, filtered through a 100-μm mesh, rinsed and transferred to a container that was filled to 50 mL with deionised water. Samples were stirred to homogenise and lift zooplankton into suspension, then five 1-mL aliquots were taken from each sample and transferred to a Bogorov chamber for analysis under a Leica S91 stereo microscope equipped with the ICC50 W camera kit and Leica LAS software (ver. 4.12, see https://www.leica-microsystems.com/products/microscope-software/p/leica-application-suite/). Each zooplankter was counted, measured and identified to family level. Samples were predominantly composed of Copepoda (89%), Cladocera (10%) and Ostrocoda (1%). Body measurements of Copepoda were made from the head to the base of the caudal setae (inclusive of rami in adults), Cladocera from the head to the base of the body excluding the tail spine and Ostracoda along the long axis of the carapace. To determine the dry mass (μg) of each individual, we employed ‘pooled’ length–weight regression equations from Bottrell et al. (1976) (for Copepoda and Cladocera) and Shmeleva (1965) (for Ostracoda). Zooplankton dry mass for each site was calculated as the sum of the dry mass of all individuals in each 5-mL aliquot from all three site replicates. This value was then converted to dry mass per volume (μg m−3) for statistical analysis.
Zooplankton do not feature in the diet of all fish species (post-larval stage); therefore, only species that are known to consume zooplankton were included in the analysis of top–down and bottom–up processes. These were bony bream (Nematalosa erebi), barred grunter (Amniataba percoides), Prince Regent hardyhead (Craterocephalus lentiginosus), western rainbowfish (Melanotaenia australis), spangled perch (Leiopotherapon unicolor) and Fitzroy River glassfish (Ambassis spp.) (Pusey et al. 2004; Medeiros and Arthington 2008). We derived the total abundance of zooplanktivorous fish at each site, which was expressed as catch per unit effort.
Data analysis
All data analysis was conducted using a combination of specific and base packages within program R (ver. 4.1.2, R Foundation for Statistical Computing, Vienna, Austria, see https://www.r-project.org/). We investigated the diversity of physical and chemical parameters and biological assemblages across sites by using a variety of techniques. Physical and chemical differences among macro-habitat and meso-habitat types were visually assessed using box plots and principal-component analysis (PCA). Data used in PCA analysis were first natural-log or power transformed prior to normalisation (subtract the mean and divide by the standard deviation) to account for skewed data (Wold et al. 1987).
We examined spatial and temporal variation in ecological processes with four linear mixed-effects models. The first model (Model i) assessed top–down and bottom–up forces shaping phytoplankton biomass in floodplain pools. Chl-a was the dependant variable and covariates were included as independent variables to examine the influence of nutrient availability (nitrogen and phosphorus), grazing pressure (zooplankton biomass), meso-habitat (pond form, channel form) and season (early or late dry season). The second model (Model ii) was used to investigate top–down and bottom–up forces influencing a primary consumer in floodplain pools. Zooplankton biomass (dependent variable) was examined as a function of food availability (bottom–up), predation (top–down) and season. Main effects included phytoplankton biomass (Chl-a), zooplanktivore fish abundance and sampling period (early or late dry season). The third model (Model iii) assessed top–down and bottom–up forces acting on phytoplankton in main-channel pools by using Chl-a as the dependent variable. Main effects included nutrient availability (nitrogen and phosphorus) and grazing pressure (zooplankton biomass). Model iv examined bottom–up forces acting on zooplankton in the main channel by using zooplankton biomass as the dependant variable and Chl-a biomass as the independent variable. All main-channel pools were sampled in the late dry season, so no ‘season’ covariate was included in Models iii or iv. In all models, ‘site’ was included as a random effect in recognition of the non-independence of zooplankton samples, i.e. three samples at each site. To meet parametric assumptions, dependant variables were either natural-log transformed or power transformed and continuous independent variables were either natural-log or power transformed prior to analysis. After transformation, continuous covariates were centred on zero and scaled to one standard deviation. Correlation between covariates was <0.7 (Pearson correlation coefficients).
All models investigating ecological processes were fitted in a Bayesian framework using the Gibbs sampler JAGS (ver. 4.3.0, see https://sourceforge.net/projects/mcmc-jags/files/JAGS/4.x/Windows/; Plummer 2003) called within program R (ver. 4.1.2, R Foundation for Statistical Computing, see https://www.r-project.org/) using package R2jags (ver. 0.7-1, Y.-S. Su and M. Yajima, see https://CRAN.R-project.org/package=R2jags). Two MCMC chains were run for 10,000 iterations with a thin rate of 1. The first 5000 iterations were discarded, leaving a posterior sample of 10,000 for inference. Chains were considered converged when (Gelman et al. 2004). Model fit was assessed using a combination of residual and Q–Q plots. We used Student’s t-distribution priors with σ = 1.8 and υ = 2 for the standard deviation of all random effects, as per Beesley et al. (2023). To avoid model overfitting, we conducted model regularisation using stochastic search variable selection (SSVS) (George and McCulloch 1993; O’Hara and Sillanpää 2009). This procedure performs parameter shrinkage on effect parameters with conditional priors of:
where θ is the covariate effect parameter and w is an inclusion parameter that can take the value of 0 or 1. When w = 1 the variance of the prior σ2 = 100, which gives a standard normal uninformative prior for θ. When w = 0, the variance σ2 = 0.01, which specifies a region of high probability at θ = 0, effectively excluding the parameter from the model. Bernoulli priors with a probability of 0.5 were specified for w. This assigned equal probability of inclusion or exclusion for each covariate in our models. The posterior mean of w can be interpreted as support for the inclusion or exclusion of each covariate from the best predictive model with values of 0 = low support and 1 = strong support (Barbieri and Berger 2004). The advantage of SSVS is that the effect estimates and model predictions are automatically model averaged, thus optimising the predictive capacity of the model (George and McCulloch 1993; O’Hara and Sillanpää 2009). Covariate effects were considered statistically significant from zero when 95% Bayesian credible intervals of the model-averaged posterior samples did not include zero, approximating α ≤ 0.05.
Results
In total, 16,497 individual zooplankton were counted, measured and identified from 20 floodplain pools and 10 main-channel pools. In total, 25,827 individual fishes comprising 17 species were caught from the 20 floodplain pools. From this total, 25,393 individuals belonged to the six species identified as zooplanktivores, which were used in analysis of top–down and bottom–up processes in floodplain habitats.
Physical and chemical characterisation
Physical and chemical conditions varied substantially among sites. The greatest differences were observed between the main-channel and pond-form floodplain pools, primarily being related to differences in water depth, habitat complexity, turbidity, dissolved nitrogen content and Chl-a biomass. These parameters were strongly loaded along PC1, which accounted for 45.83% of variation (Fig. 2). Main-channel pools tended to be deep with clear water and high habitat complexity, whereas pond-form floodplain pools were shallow and turbid with greater nutrient loading, Chl-a biomass and homogenous habitat. Channel-form floodplain pools often represented a mid-point between the extremes of pond-form floodplain pools and the main channel (Fig. 2). Water depth and habitat complexity tended to be greater in channel-form pools than pond-form pools, and turbidity and Chl-a were generally lower. However, channel-form floodplain pools were still typically shallower, more turbid and with higher Chl-a concentrations than in the main-channel pools (Table 1, Fig. 3, 4). Differences in habitat complexity between macro-habitat and meso-habitats were primarily driven by variable water depths and substrate types (Fig. 3). Physical and chemical conditions were substantially more variable in floodplain habitats than in the main channel, with pond-form floodplain pools displaying the greatest variability (Table 1, Fig. 3).
Principal component analysis and ordination bi-plot of physical, chemical and chlorophyll-a biomass data collected in pond-form (n = 14) and channel-form (n = 6) floodplain pools and main-channel pools (n = 6).
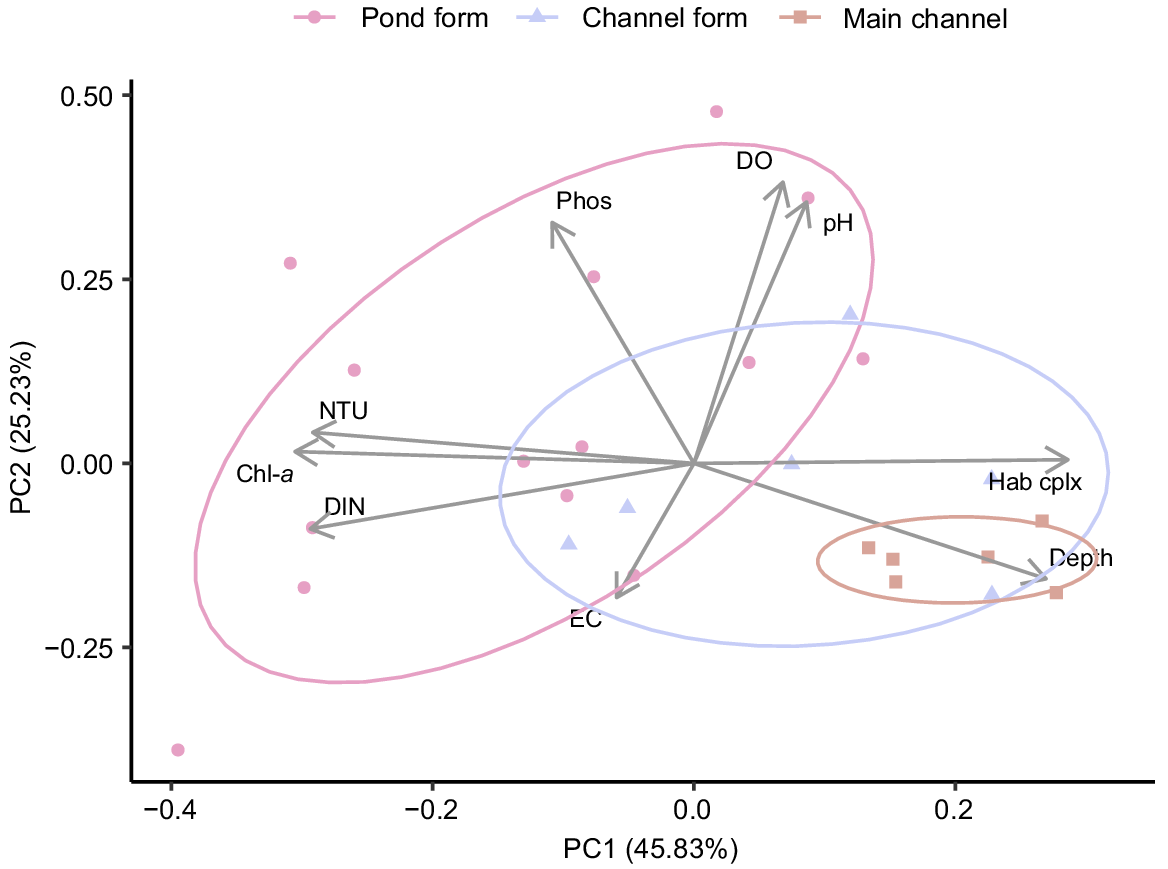
Physio-chemical parameter | Floodplain | Main channel | ||
---|---|---|---|---|
Pond form | Channel form | |||
Average water depth (m) | 0.39 ± 0.19 | 0.74 ± 0.32 | 4.68 ± 3.02 | |
Ammonia (μg L−1) | 80.09 ± 187.01 | 16.19 ± 5.04 | 15.57 ± 4.56 | |
Nitrate and nitrite (μg L−1) | 17.96 ± 24.01 | 4.57 ± 1.22 | 4.35 ± 0.84 | |
Total dissolved inorganic N (μg L−1) | 98.04 ± 187.96 | 20.7 ± 6.08 | 19.91 ± 5.8 | |
Phosphorus (μg L−1) | 10.31 ± 12.71 | 3.98 ± 2.67 | 2.66 ± 0.35 | |
Dissolved oxygen (mg L−1) | 10.06 ± 4.20 | 8.07 ± 1.11 | 8.14 ± 0.85 | |
pH | 9.12 ± 1.09 | 8.72 ± 0.47 | 8.79 ± 0.10 | |
Electrical conductivity (μS cm−1) | 250.93 ± 135.00 | 217.31 ± 96.94 | 350.37 ± 30.40 | |
Salinity (ppt) | 0.12 ± 0.07 | 0.10 ± 0.05 | 0.16 ± 0.01 | |
Turbidity (NTU) | 160.44 ± 244.73 | 24.92 ± 25.65 | 2.43 ± 1.64 | |
Habitat-complexity score | 4 ± 2.25 | 7.33 ± 1.63 | 7.17 ± 0.41 | |
Large woody debris (%) | 0.29 ± 0.83 | 0.75 ± 0.61 | 0.32 ± 0.38 | |
Small woody debris (%) | 5.0 ± 13.09 | 2.92 ± 3.58 | 0.32 ± 0.38 | |
Mud substrate (%) | 89.93 ± 26.27 | 82.50 ± 17.82 | 22.17 ± 19.70 | |
Sand substrate (%) | 6.07 ± 13.37 | 11.33 ± 15.32 | 57.0 ± 17.61 | |
Gravel substrate (%) | 0.36 ± 0.63 | 2.83 ± 4.02 | 20.0 ± 22.80 | |
Cobble substrate (%) | 1.36 ± 4.80 | 3.33 ± 6.06 | 0.83 ± 2.04 | |
Macrophytes (%) | 26.0 ± 36.73 | 18.67 ± 30.54 | 4.33 ± 5.71 |
Values show means ± s.d.
Box plots of raw physical and chemical data collected in pond-form (n = 14) and channel-form (n = 6) floodplain pools and main-channel pools (n = 6). Bold line indicates the median value, the two hinges are the 25th (lower) and 75th (upper) quartiles, whiskers represent the inter-quartile range and black circles are outlying points.
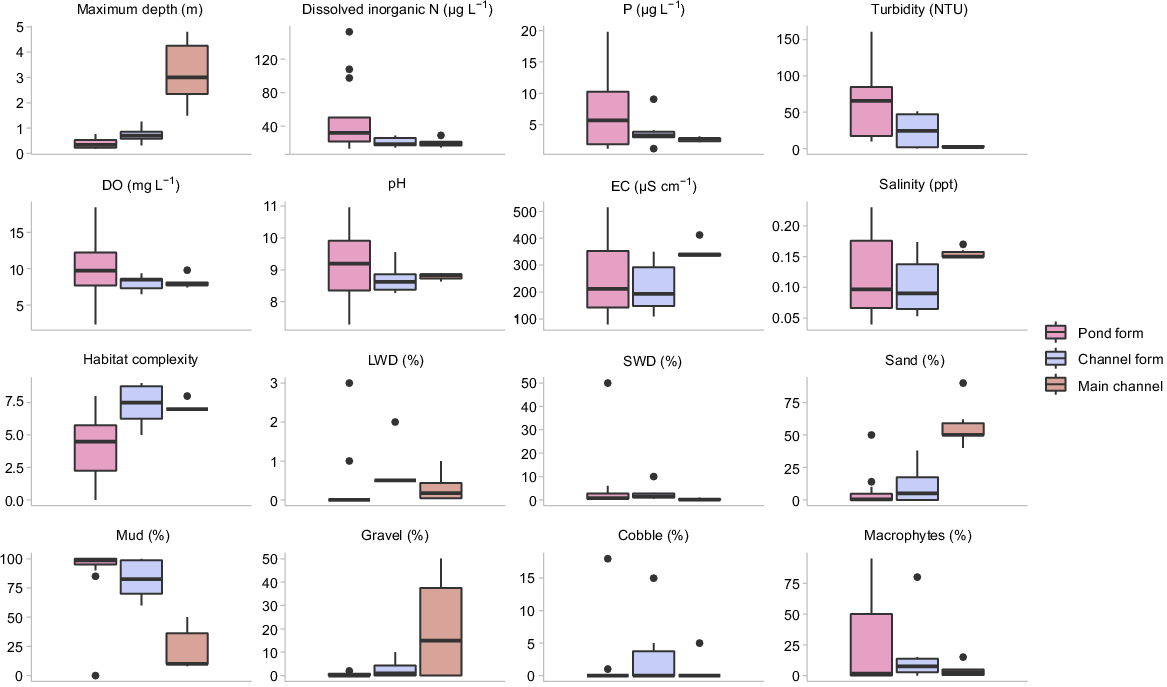
Box plot showing (a) log-transformed chlorophyll-a biomass and (b) log-transformed zooplankton biomass in pond-form (n = 14) and channel-form (n = 6) floodplain pools and main-channel pools (n = 10). Bold line indicates the median value, the two hinges are the 25th (lower) and 75th (upper) quartiles, whiskers represent the inter-quartile range and black circles are outlying points.
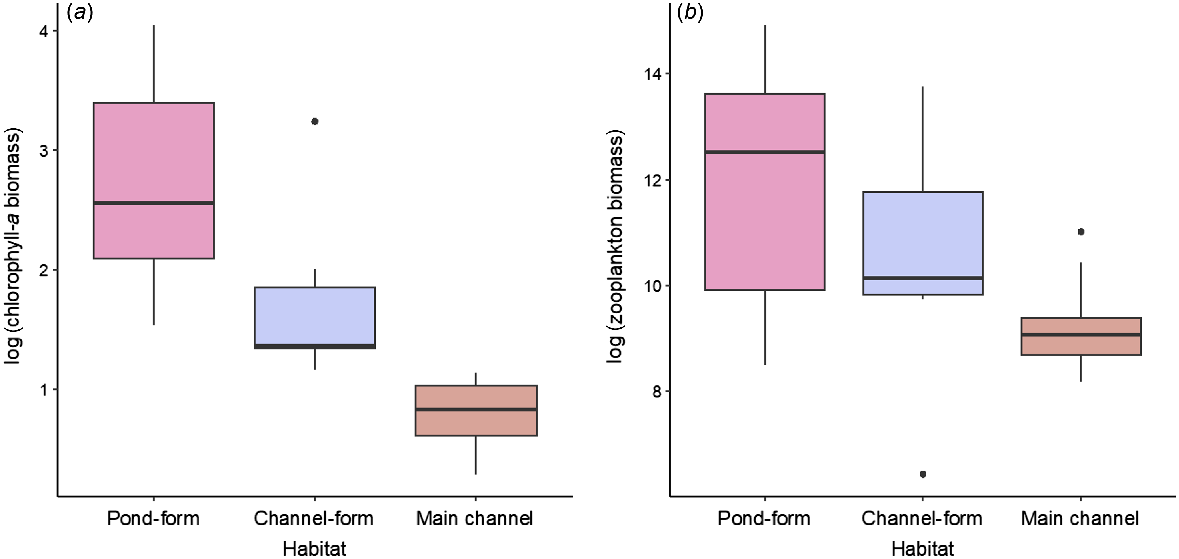
Biological characterisation
There were considerable differences in the biological characterisation of floodplain habitats and the main channel. Chl-a biomass varied from 4.63 to 56.87 mg m−3 (mean 20.74 mg m−3) in pond-form floodplain pools, from 3.20 to 25.54 mg m−3 (mean 7.97 mg m−3) in channel-form floodplain pools and from 1.82 to 6.98 mg m−3 (mean 3.28 mg m−3) in main-channel pools (Fig. 4a). Estimates of zooplankton biomass showed a similar pattern and varied substantially between macro-habitat and meso-habitat types (Fig. 4b). For example, mean zooplankton biomass was 663.47 mg m−3 in pond-form floodplain pools, 205.13 mg m−3 in channel-form floodplain pools and 18.84 mg m−3 in main-channel pools. Zooplankton biomass was considerably more variable in floodplain pools than in the main-channel, which displayed minimal variability (Fig. 4b, Table 1).
Ecological processes
Top–down and bottom–up processes varied in strength spatially between macro-habitats (i.e. main-channel and floodplain), but no changes were detected over time. In floodplain pools, bottom–up processes controlled phytoplankton and zooplankton biomass. Chl-a was strongly positively influenced by dissolved inorganic nitrogen (Table 2, Model i; Fig. 5) and zooplankton biomass was positively influenced by Chl-a concentration (Table 2, Model ii; Fig. 6). No top–down effects were observed in either floodplain model, with both zooplankton biomass and zooplanktivorous fish abundances having a negligible effect on Chl-a biomass and zooplankton biomass respectively (Table 2). Contrary to the strong bottom–up processes observed on the floodplain, in the main channel we found no evidence of bottom–up or top–down effects and none of the covariates were good predictors of Chl-a or zooplankton biomass (Table 2, Models iii and iv). We found no evidence of temporal variation in top–down and bottom–up processes. On the floodplain, the strength of top–down and bottom–up forces remained consistent between the early dry season and late dry season (Table 2). Additionally, we observed no change in the strength of top–down and bottom–up forces between floodplain meso-habitats, i.e. pond-form pools or channel-form pools (Table 2).
Model | Variable | Effect size | s.d. | LCI | UCI | w | |
---|---|---|---|---|---|---|---|
(i) Phytoplankton in floodplain pools | Dissolved inorganic nitrogen A | 0.724 | 0.135 | 0.416 | 0.954 | 1.00 | |
Phosphorus | 0.097 | 0.135 | −0.037 | 0.408 | 0.52 | ||
Zooplankton biomass | 0.064 | 0.134 | −0.183 | 0.395 | 0.48 | ||
Meso-habitat | 0.061 | 0.194 | −0.336 | 0.545 | 0.36 | ||
Season | −0.268 | 0.328 | −0.991 | 0.073 | 0.59 | ||
(ii) Zooplankton in floodplain pools | Chlorophyll-a biomass A | 1.418 | 0.422 | 0.613 | 2.237 | 0.99 | |
Zooplanktivorous fish CPUE | 0.193 | 0.355 | −0.255 | 1.080 | 0.45 | ||
Meso-habitat | −0.019 | 0.373 | −1.090 | 0.740 | 0.39 | ||
Season | −0.619 | 0.630 | −1.714 | 0.273 | 0.67 | ||
(iii) Phytoplankton in main-channel pools | Dissolved inorganic nitrogen | 0.013 | 0.058 | −0.069 | 0.200 | 0.20 | |
Phosphorus | −0.009 | 0.056 | −0.183 | 0.069 | 0.17 | ||
Zooplankton biomass | 0.037 | 0.086 | −0.027 | 0.274 | 0.30 | ||
(iv) Zooplankton in main-channel pools | Chlorophyll-a biomass | 0.161 | 0.381 | −0.624 | 0.946 | 0.50 |
Credible intervals that do not overlap zero indicate significance at α = 0.05. w is an indication of the support for the inclusion of a given variable in the best predictive model, with 0 = low support and 1 = strong support. s.d., standard deviation; LCI, 95% lower credible interval; UCI, 95% upper credible interval; w, inclusion probability.
Model-predicted chlorophyll-a biomass v. dissolved inorganic nitrogen concentration in floodplain pools (n = 20). Area of shading surrounding the central line represents 95% Bayesian credible intervals. Circles and triangles are transformed data representing pond-form (n = 14) and channel-form (n = 6) pools respectively.
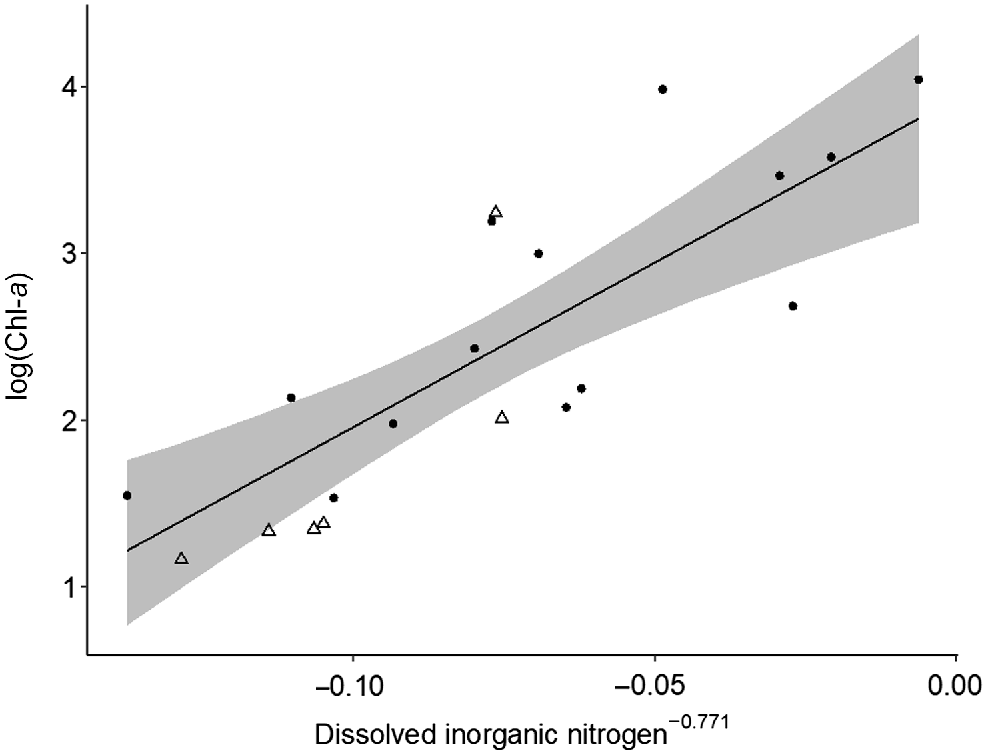
Model-predicted zooplankton biomass v. chlorophyll-a biomass in floodplain pools (n = 20). Area of shading surrounding the central line represents 95% Bayesian credible intervals. Circles and triangles are transformed data representing pond-form (n = 14) and channel-form (n = 6) pools respectively.
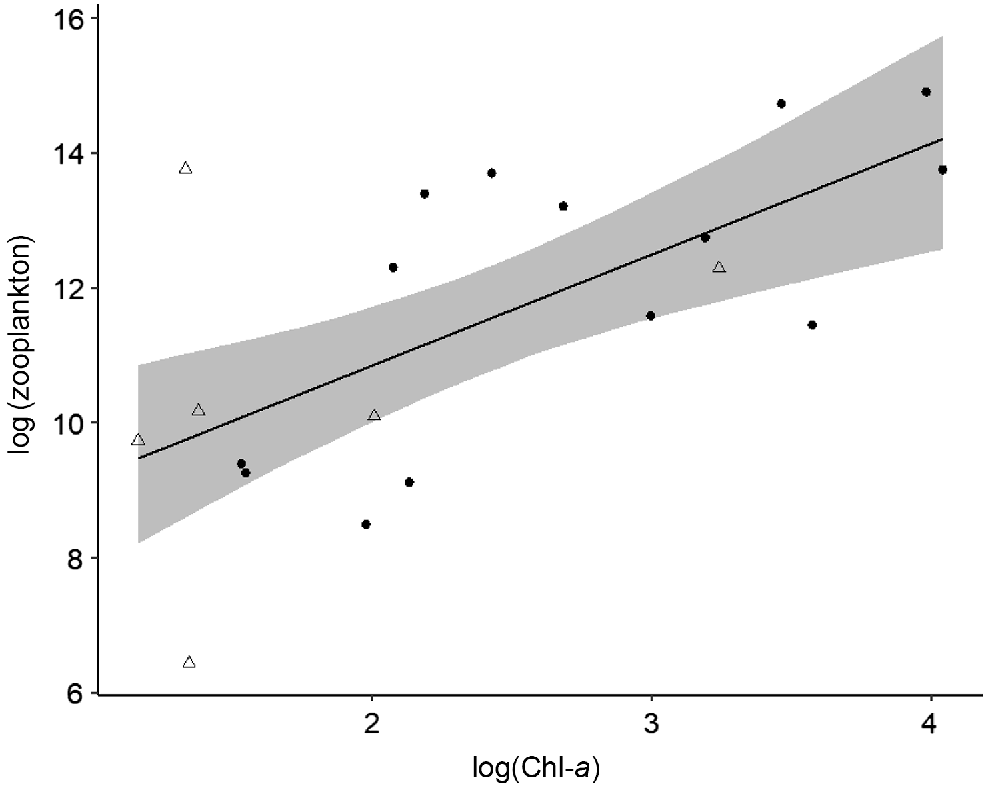
Discussion
On the floodplain, bottom–up processes drove water-column productivity to a degree not observed in the main channel, providing a valuable resource pathway supporting consumer populations locally and catchment wide. In floodplain pools, high nutrient concentrations increased phytoplankton biomass, providing an abundant food resource, which boosted zooplankton populations. The strength of bottom–up forces on the floodplain was consistent in the early and late dry season for primary producers and primary consumers alike. No significant top–down effects were detected in either meso-habitat. Floodplain pools showed considerable physical, chemical and biological heterogeneity, which contrasted with the relatively uniform main channel. Floodplain pools were shallow and more turbid with higher nutrient loads than the main-channel, which was deep, clear and nutrient-limited. Phytoplankton and zooplankton biomass were considerably greater and more variable in floodplain pools than in the main-channel, where both were largely absent. In the face of water-resource development, maintaining aspects of the natural flow regime that promote habitat heterogeneity in the lower catchment is crucial for preserving local and landscape-scale ecosystem functioning.
Hydrological variability through space and time promotes habitat heterogeneity (Junk et al. 1989; Poff and Ward 1990; Poff et al. 1997; Amoros and Bornette 2002). The physical and chemical characteristics of main-channel and floodplain habitats in the Fitzroy River were markedly different. For instance, main-channel sites tended to be deep with clear water and high habitat complexity, whereas pond-form floodplain pools were shallow and turbid with greater nutrient loading and homogenous habitat. Pond-form pools typically displayed the greatest heterogeneity, which was in stark contrast to the main-channel, which displayed little variation in physical and chemical conditions among pools. Channel-form floodplain pools often represented a mid-point between the extremes of the main-channel and pond-form pools. Biotic assemblages displayed a pattern concurrent with the different physical and chemical conditions observed across macro- and meso-habitats. Phytoplankton and zooplankton biomasses were greater and more variable in pond-form floodplain pools compared to channel-form floodplain pools and substantially greater than in the main channel.
Local physical and chemical conditions, along with biological communities, influence ecosystem structure and function (Thorp et al. 2006; Warfe et al. 2011; Rolls et al. 2012). In this study, we have shown that the substantial differences in physical and chemical conditions and biological assemblages between floodplain and main-channel habitats in the Fitzroy River drive differences in the strength of top–down and bottom–up processes. Bottom–up forces in floodplain pools were greater than those observed in the main channel, where statistically significant bottom–up or top–down effects were not detected. Bottom–up effects on the floodplain were driven by nutrient availability, which increased phytoplankton biomass, boosting zooplankton populations. Elevated nutrient concentrations in floodplain habitats have been observed elsewhere in the wet–dry tropics and have been shown to stimulate water-column Chl-a concentrations (Pettit et al. 2012; Molinari et al. 2021). There are likely to be several mechanisms that contribute to increased nutrient loads in shallow floodplain pools, including mobilisation of latent terrestrial energy sources during flooding (Baldwin and Mitchell 2000; Balzer et al. 2023), wind-derived re-suspension of nutrient-rich sediments (Søndergaard et al. 1992) and substrate disturbance or waste inputs from large herbivore activity, e.g. cattle (Dixon et al. 2011; Pettit et al. 2012). Deep water and dense riparian vegetation at main-channel sites are likely to buffer against wind-driven sediment re-suspension, and annual wet-season flows scour sandy benthic substrate, transporting nutrients downstream to the estuary (Townsend and Padovan 2005). Steep banks probably restrict cattle access to the river along large parts of the main-channel, limiting direct nutrient inputs to local ‘hotspots’ of cattle activity. Although field observations of pugging and cattle presence suggested increased activity at some floodplain pools v. the main channel, cattle activity was not quantified as part of this study.
Our finding that bottom–up forces were dominant is consistent with studies of other tropical floodplain systems globally (Trevisan and Forsberg 2007; Rejas and Muylaert 2010; Frau et al. 2019; Toruan et al. 2022). In the Australian wet–dry tropics, few studies have examined phytoplankton on the floodplain and none have simultaneously assessed the influence of top–down and bottom–up processes. However, pertinent research from the Cloncurry (Faggotter et al. 2013) and Mitchell (Pettit et al. 2012) rivers confirmed that nitrogen is a key driver of phytoplankton production on northern Australian floodplains. Resource limitation of phytoplankton has also been observed in the main channel of several rivers in the wet–dry tropics (Townsend and Edwards 2003; Webster et al. 2005; Townsend et al. 2012; Faggotter et al. 2013); however, the present study found no direct evidence for bottom–up control of phytoplankton in the Fitzroy River main channel. Light availability and high flow velocity can also constrain phytoplankton growth (Burford et al. 2012), although these factors are likely to have had little effect because water conditions were clear, shading from riparian vegetation was minimal and limited to channel margins and flow velocity was low. It is probable that phytoplankton production was constrained by nutrient availability; however, this relationship could not be demonstrated because of the low and uniform dissolved inorganic nitrogen, phosphorus and Chl-a concentrations in the main-channel. We found no evidence of consumer regulation of phytoplankton or zooplankton in floodplain or main-channel habitats, a finding consistent with a study of the Cloncurry River, Queensland (Faggotter et al. 2013). Across South America and temperate North America, consumer regulation of zooplankton by fish and phytoplankton by zooplankton has been observed (Schaus et al. 2002; Frau 2022). However, for significant top–down effects to occur, the intensity of predation needs to be sufficiently high, which even in aquatic systems is likely to be limited in time and space (Polis and Strong 1996). The impact of top–down forces in freshwater habitats can also be influenced indirectly by consumer effects on nutrient cycling. For example, fish and zooplankton, by excretory processes, can supply nitrogen and phosphorus at rates capable of supporting the nutrient demands of primary producers (Vanni 2002). Indeed, this indirect bottom–up process can override any top–down effect of consumers on phytoplankton or zooplankton biomass (Rejas et al. 2005).
The patterns and strength of top–down and bottom–up forces in floodplain habitats in the Fitzroy River were similar in the early and late dry season. Early in the dry season post-flooding, bottom–up forces are typically strong owing to increased availability of nutrients, which boosts primary production (Bunn et al. 2006; Pettit et al. 2012). Other studies have demonstrated that consumer regulation of resources can vary in strength through time as a function of seasonal hydrological variability, where low-water conditions during the dry season facilitate an increase in top–down forces as a result of increased consumer density (Winemiller et al. 2014; Frau et al. 2015). In central Australia, fish biomass in isolated waterholes has been shown to decrease significantly as the dry season progresses (Arthington et al. 2005; Burford et al. 2008), potentially reducing the intensity of top–down forces on zooplankton. Moreover, the release of nutrients into waterholes due to consumer mortality can replenish the available nutrient pool, which otherwise declines over time, stimulating primary production and maintaining the strength of bottom–up forces (Burford et al. 2008). Fish mortality during the late dry season may therefore directly reduce the strength of top–down forces at a time when low-water conditions would usually facilitate an increase in predation, and indirectly increase the strength of bottom–up forces during a period of diminishing nutrient availability for primary producers. This process may stabilise top–down and bottom–up processes over the dry season and contribute to the absence of a seasonal pattern observed on the floodplain in the Fitzroy River. However, repeat sampling of pools throughout the dry season is required to verify this.
In freshwater ecosystems around the world, the phytoplankton resource pathway provides an important contribution to consumer biomass (Schaus et al. 2002; Frau 2022). However, in the wet–dry tropics and dryland systems of northern and central Australia, benthic algae is considered to be the primary resource pathway sustaining both main-channel and floodplain heterotroph populations (Bunn et al. 2003; Douglas et al. 2005; Pettit et al. 2017). Nonetheless, there is mounting evidence that the phytoplankton pathway, from zooplankton, may provide an important contribution to consumer diets as well as influence broader ecosystem functioning (Medeiros and Arthington 2008; Pusey et al. 2021; Pratt et al. 2023). Indeed, Pratt et al. (2023) demonstrated that zooplankton biomass was the primary mechanism driving the growth rate of young-of-year bony bream in the Fitzroy River (WA), where high zooplankton biomass was exclusive to the floodplain. Faster growth in floodplain habitats led to an increase in modelled survival, suggesting that the floodplain has the potential to provide a disproportionally large contribution of this ecologically important species to the riverine meta-population. Similar benefits may apply to other fish species given that zooplankton are the primary food resource of most larval fishes at the onset of exogenous feeding, regardless of adult dietary guild (Barlow et al. 1993; Nunn et al. 2012). Zooplankton also contribute to the diet of many waterbirds, which are capable of transporting energy across catchments (Kingsford et al. 2010). Thus, the floodplain and the abundant zooplankton, which are exclusive to this habitat, are not just important for local food webs but may also contribute to main-channel and landscape-scale ecosystem functioning.
This study is one of few in northern Australia to extensively characterise floodplain wetlands, providing an important contribution to baseline data of the physical, chemical and biological conditions in these habitats. With the exception of Pettit et al. (2012), the majority of studies have focussed on the main channel and only occasionally included a floodplain pool. Pertinent information from this body of research has been synthesised and compared with the present study to establish the transferability of our results. The physical and chemical conditions of pools sampled were consistent with those that have been reported in other studies of the Fitzroy River (Kay et al. 1999; Fellman et al. 2011; Burrows et al. 2020; Gleiss et al. 2021) and elsewhere in the wet–dry tropics (Dixon et al. 2011; Pettit et al. 2012; Townsend et al. 2012; Faggotter et al. 2013). Main-channel Chl-a concentration was in line with results reported by Gleiss et al. (2021) in two dry-season refuge pools in the Fitzroy River main stem, and is consistent with what has been reported in the main channel of other river systems across northern Australia (Webster et al. 2005; Rayner et al. 2009; Townsend et al. 2012; Jardine et al. 2015). Elevated concentrations of water-column Chl-a observed in floodplain pools compared with the main channel were similar to those reported in several other floodplain rivers in the wet–dry tropics (Pettit et al. 2012; Molinari et al. 2021) and elsewhere in Australia (Ning and Nielsen 2011). Zooplankton biomass is consistent with that reported by Pratt et al. (2023) in both main-channel and floodplain sites in the Fitzroy River. Zooplankton densities are in the same range as that reported by Faggotter et al. (2013) for main-channel and floodplain pools in the Edith River, Northern Territory, and for ephemeral floodplain wetlands in south-eastern Australia (individuals per litre) (James et al. 2008), but were considerably higher in both main-channel and floodplain habitats than that reported in the slack waters of the Mulgrave River, tropical Queensland (Godfrey et al. 2021). Overall, the results from the present study are consistent with what has been reported elsewhere in the literature, suggesting the characterisation of floodplain pools presented herein may have high transferability to other systems across northern Australia.
In this study, we have demonstrated that floodplain pools fill a unique role in the wider riverine ecosystem, even when floodplain inundation is brief, a finding that has implications for local and landscape-scale ecosystem functioning. This information can inform the management of the Fitzroy River as well as other systems throughout northern Australia and elsewhere. In the Fitzroy River, the natural flow regime is relatively unmodified compared with that of other large rivers globally. However, along with several other rivers in the region, there is interest in developing its water resources to support irrigated agriculture (Petheram et al. 2018b), a process which will likely alter the natural flow regime. Indeed, modelling suggests that development has the potential to reduce hydrological connectivity between the river and its floodplain, decreasing the area, depth and duration of seasonal floodplain inundation (Karim et al. 2018). Floodplain habitats in the Fitzroy River display substantial physical, chemical and biological heterogeneity, and support water-column productivity at a level well above that which is observed in the main channel. Pond-form floodplain pools displayed the highest nutrient concentrations and supported the greatest phytoplankton and zooplankton biomass. For this subhabitat type to fill, larger overbank flood events that inundate the floodplain proper are required. Channel-form floodplain habitats are likely to back-fill more frequently and during periods of lower main-channel discharge than pond-form pools. Water managers should account for the different inundation requirements needed to maintain both floodplain meso-habitats when designing water planning policy. Floodplain habitats are not just important for local biological communities, they also contribute to landscape-scale ecosystem functioning. Floodplain contributions to the main channel have been observed in other river systems globally (Tockner et al. 1999; Jardine et al. 2012b; Furst et al. 2014; Sturrock et al. 2022). Thus, any modification of the natural flow regime that might negatively affect floodplain habitats has the potential to alter ecosystem functioning at a local and landscape scale. It is therefore vital that water planning policy for the lower Fitzroy River catchment protects the aspects of the natural flow regime that promote habitat heterogeneity, to preserve ecosystem functioning in both floodplain and main-channel habitats.
Data availability
All data associated with this study are available through the University of Western Australia’s research repository (https://research-repository.uwa.edu.au/en/datasets/).
Conflicts of interest
The authors declare that they have no conflicts of interest. Recommendations regarding water planning policy are the opinions of the authors and not the State Government of Western Australia.
Declaration of funding
This project was supported by the Australian Government’s National Environmental Science Program through the Northern Australia Environmental Resources Hub Project 1.3.3.
Author contributions
Oliver P. Pratt, Leah S. Beesley, Samantha A. Setterfield and Michael M. Douglas provided the conceptualisation and developed the methods. Oliver P. Pratt and Leah S. Beesley conducted the research and provided data analysis, data interpretation, and preparation of figures and tables. All authors contributed to the writing of this paper.
Acknowledgements
We gratefully acknowledge the Traditional Owners of the Country on which this work was undertaken; the Nyikina-Mangala people, Yanunijarra and Gooniyandi people, and recognise their continuing connection to land, waters and culture. We pay our respects to their Elders, past, present and emerging. We thank the Prescribed Body Corporates of the different native title groups, and representatives from Yungngora Community, for providing us with permission to undertake research on Country. Thanks go to the Indigenous Rangers and Ranger Co-ordinators who assisted with field work and we acknowledge the assistance of the Kimberley Land Council in project facilitation, particularly Karen Dayman. We thank the pastoral managers of Jubilee Downs, Myroodah, Mount Anderson, Millijidee, Noonkanbah and Gogo Stations for their cooperation.
References
Amoros C, Bornette G (2002) Connectivity and biocomplexity in waterbodies of riverine floodplains. Freshwater Biology 47, 761-776.
| Crossref | Google Scholar |
Arthington AH, Balcombe SR, Wilson GA, Thoms MC, Marshall J (2005) Spatial and temporal variation in fish-asselemblage structure in isolated waterholes during the 2001 dry season of an arid-zone floodplain river, Cooper Creek, Australia. Marine and Freshwater Research 56, 25-35.
| Crossref | Google Scholar |
Baldwin DS, Mitchell AM (2000) The effects of drying and re-flooding on the sediment and soil nutrient dynamics of lowland river–floodplain systems: a synthesis. Regulated Rivers: Research & Management 16, 457-467.
| Crossref | Google Scholar |
Balzer MJ, Hitchcock JN, Hadwen WL, Kobayashi T, Westhorpe DP, Boys C, Mitrovic SM (2023) Experimental additions of allochthonous dissolved organic matter reveal multiple trophic pathways to stimulate planktonic food webs. Freshwater Biology 68, 821-836.
| Crossref | Google Scholar |
Barbieri MM, Berger JO (2004) Optimal predictive model selection. The Annals of Statistics 32, 870-879.
| Crossref | Google Scholar |
Barlow CG, Rodgers LJ, Palmer PJ, Longhurst CJ (1993) Feeding habits of hatchery-reared barramundi Lates calcarifer (Bloch) fry. Aquaculture 109, 131-144.
| Crossref | Google Scholar |
Beesley LS, Pusey BJ, Douglas MM, Gwinn DC, Canham CA, Keogh CS, Pratt OP, Kennard MJ, Setterfield SA (2020) New insights into the food web of an Australian tropical river to inform water resource management. Scientific Reports 10, 14294.
| Crossref | Google Scholar | PubMed |
Beesley LS, Killerby-Smith S, Gwinn DC, Pusey BJ, Douglas MM, Novak PA, Tayer TC, Keogh CS, Kennard MJ, Canham CA, Setterfield SA (2023) Modelling the longitudinal distribution, abundance, and habitat use of the giant freshwater shrimp (Macrobrachium spinipes) in a large intermittent, tropical Australian river to inform water resource policy. Freshwater Biology 68, 61-76.
| Crossref | Google Scholar |
Bottrell HH, Duncan A, Gliwicz ZM, Grygierek E, Herzig A, Hillbricht-Ilkowska A, Kurosawa H, Larsson P, Weglenska T (1976) A review of some problems in zooplankton production studies. Norwegian Journal of Zoology 24, 419-456.
| Google Scholar |
Bunn SE, Davies PM, Winning M (2003) Sources of organic carbon supporting the food web of an arid zone floodplain river. Freshwater Biology 48, 619-635.
| Crossref | Google Scholar |
Bunn SE, Thoms MC, Hamilton SK, Capon SJ (2006) Flow variability in dryland rivers: boom, bust and the bits in between. River Research and Applications 22, 179-186.
| Crossref | Google Scholar |
Bureau of Meteorology (2024) Climate statistics for Australian locations. Available at http://www.bom.gov.au/climate/averages/tables/cw_003006.shtml [Verified 13 February 2024]
Burford MA, Cook AJ, Fellows CS, Balcombe SR, Bunn SE (2008) Sources of carbon fuelling production in an arid floodplain river. Marine and Freshwater Research 59, 224-234.
| Crossref | Google Scholar |
Burford MA, Webster IT, Revill AT, Kenyon RA, Whittle M, Curwen G (2012) Controls on phytoplankton productivity in a wet–dry tropical estuary. Estuarine, Coastal and Shelf Science 113, 141-151.
| Crossref | Google Scholar |
Burrows RM, Beesley L, Douglas MM, Pusey BJ, Kennard MJ (2020) Water velocity and groundwater upwelling influence benthic algal biomass in a sandy tropical river: implications for water-resource development. Hydrobiologia 847, 1207-1219.
| Crossref | Google Scholar |
Burrows RM, Douglas MM, Bunn SE, Pusey BJ, Setterfield SA, Garcia EA, Kennard MJ (2022) 4. Case study: Tropical freshwater food webs- spatial and conceptual gaps in our understanding of their ecology and management. In ‘Environmental flows synthesis to support uptake of environmental flows research in northern Australia’. (Eds MJ Kennard, LS Beesley, SE Bunn, MA Burford, RM Burrows, CA Canham, D Crook, MM Douglas, EA Garcia, A King, SA Setterfield, K O’Mara, J Marshall, BJ Pusey, B Stewart-Koster, M Venarsky) pp. 26–49. (Griffith University: Brisbane, Qld, Australia)
das Candeias DA, Moi DA, Simões NR, Azevedo F, Meerhoff M, Bonecker CC (2022) High temperature, predation, nutrient, and food quality drive dominance of small-sized zooplankton in Neotropical lakes. Aquatic Sciences 84, 49.
| Crossref | Google Scholar |
Dixon I, Dobbs R, Townsend SA, Close P, Ligtermoet E, Dostine P, Duncan R, Kennard MJ, Tunbridge D (2011) Trial of the Framework for the Assessment of River and Wetland Health (FARWH) in the wet–dry tropics for the Daly and Fitzroy Rivers. Tropical Rivers and Coastal Knowledge (TRaCK) research consortium, Charles Darwin University, Darwin, NT, Australia.
Douglas MM, Bunn SE, Davies PM (2005) River and wetland food webs in Australia’s wet–dry tropics: general principles and implications for management. Marine and Freshwater Research 56, 329-342.
| Crossref | Google Scholar |
Faggotter SJ, Webster IT, Burford MA (2013) Factors controlling primary productivity in a wet–dry tropical river. Marine and Freshwater Research 64, 585-598.
| Crossref | Google Scholar |
Feitosa IB, Huszar VLM, Domingues CD, Appel E, Paranhos R, Almeida RM, Branco CWC, Bastos WR, Sarmento H (2019) Plankton community interactions in an Amazonian floodplain lake, from bacteria to zooplankton. Hydrobiologia 831, 55-70.
| Crossref | Google Scholar |
Fellman JB, Dogramaci S, Skrzypek G, Dodson W, Grierson PF (2011) Hydrologic control of dissolved organic matter biogeochemistry in pools of a subtropical dryland river. Water Resources Research 47, W06501.
| Crossref | Google Scholar |
Frau D (2022) Grazing impacts on phytoplankton in South American water ecosystems: a synthesis. Hydrobiologia 849, 833-860.
| Crossref | Google Scholar |
Frau D, Devercelli M, José De Paggi S, Scarabotti P, Mayora G, Battauz Y, Senn M (2015) Can top–down and bottom–up forces explain phytoplankton structure in a subtropical and shallow groundwater-connected lake? Marine and Freshwater Research 66, 1106-1115.
| Crossref | Google Scholar |
Frau D, Battauz Y, Alvarenga PF, Scarabotti PA, Mayora G, Sinistro R (2019) Assessing the relevance of top–down and bottom–up effects as phytoplankton structure drivers in a subtropical hypereutrophic shallow lake. Aquatic Ecology 53, 265-280.
| Crossref | Google Scholar |
Furst DJ, Aldridge KT, Shiel RJ, Ganf GG, Mills S, Brookes JD (2014) Floodplain connectivity facilitates significant export of zooplankton to the main River Murray channel during a flood event. Inland Waters 4, 413-424.
| Crossref | Google Scholar |
Garcia EA, Townsend SA, Douglas MM (2015) Context dependency of top–down and bottom–up effects in a northern Australian tropical river. Freshwater Science 34, 679-690.
| Crossref | Google Scholar |
George EI, McCulloch RE (1993) Variable selection via Gibbs Sampling. Journal of the American Statistical Association 88, 881-889.
| Crossref | Google Scholar |
Godfrey PC, Pearson RG, Pusey BJ, Arthington AH (2021) Drivers of zooplankton dynamics in a small tropical lowland river. Marine and Freshwater Research 72, 173-185.
| Crossref | Google Scholar |
James CS, Thoms MC, Quinn GP (2008) Zooplankton dynamics from inundation to drying in a complex ephemeral floodplain wetland. Aquatic Sciences 70, 259-271.
| Crossref | Google Scholar |
Jardine TD, Pettit NE, Warfe DM, Pusey BJ, Ward DP, Douglas MM, Davies PM, Bunn SE (2012a) Consumer-resource coupling in wet–dry tropical rivers. Journal of Animal Ecology 81, 310-322.
| Crossref | Google Scholar | PubMed |
Jardine TD, Pusey BJ, Hamilton SK, Pettit NE, Davies PM, Douglas MM, Sinnamon V, Halliday IA, Bunn SE (2012b) Fish mediate high food web connectivity in the lower reaches of a tropical floodplain river. Oecologia 168, 829-838.
| Crossref | Google Scholar | PubMed |
Jardine TD, Woods R, Marshall J, Fawcett J, Lobegeiger J, Valdez D, Kainz MJ (2015) Reconciling the role of organic matter pathways in aquatic food webs by measuring multiple tracers in individuals. Ecology 96, 3257-3269.
| Crossref | Google Scholar | PubMed |
Junk WJ, Furch K (1993) A general review of tropical South American floodplains. Wetlands Ecology and Management 2, 231-238.
| Crossref | Google Scholar |
Junk WJ, Bayley PB, Sparks RE (1989) The flood pulse concept in river–floodplain systems. In ‘Proceedings of the International Large River Symposium (LARS)’, 14–21 September 1986, Honey Harbour, ON, Canada. (Ed. DP Dodge) Special Publication of Fish and Aquatic Sciences 106, pp. 110–127. (Department of Fisheries and Oceans: Ottawa, ON, Canada) Available at https://ftp.cs.ru.nl/toinesmits/Recommended_readings_IWRM_2009/Water_Ecomorphological_principles/1989JunkThe%20flood%20pulse%20concept%20in.pdf
Karim F, Peña-Arancibia J, Ticehurst C, Marvanek S, Gallant J, Hughes JM, Dutta D, Vaze J, Pethernam C, Seo L, Kitson S (2018) Floodplain inundation mapping and modelling for the Fitzroy, Darwin and Mitchell catchments. A technical report to the Australian Government from the CSIRO Northern Australia Water Resource Assessment, part of the National Water Infrastructure Development Fund: Water Resource Assesments. CSIRO, Australia.
Kay WR, Smith MJ, Pinder AM, Mcrae JM, Davis JA, Halse SA (1999) Patterns of distribution of macroinvertebrate families in rivers of north-western Australia. Freshwater Biology 41, 299-316.
| Crossref | Google Scholar |
Kennard MJ, Pusey BJ, Olden JD, Mackay SJ, Stein JL, Marsh N (2010) Classification of natural flow regimes in Australia to support environmental flow management. Freshwater Biology 55, 171-193.
| Crossref | Google Scholar |
Kingsford RT, Roshier DA, Porter JL (2010) Australian waterbirds: time and space travellers in dynamic desert landscapes. Marine and Freshwater Research 61, 875-884.
| Crossref | Google Scholar |
Koning AA, McIntyre PB (2021) Grassroots reserves rescue a river food web from cascading impacts of overharvest. Frontiers in Ecology and the Environment 19, 152-158.
| Crossref | Google Scholar |
Lear KO, Ebner BC, Fazeldean T, Whitty J, Morgan DL (2023) Inter-decadal variation in diadromous and potamodromous fish assemblages in a near pristine tropical dryland river. Ecology of Freshwater Fish 32, 444-463.
| Crossref | Google Scholar |
Lewis WM, Hamilton SK, Lasi MA, Rodríguez M, Saunders JF (2000) Ecological Determinism on the Orinoco Floodplain: a 15-year study of the Orinoco floodplain shows that this productive and biotically diverse ecosystem is functionally less complex than it appears. Hydrographic and geomorphic controls induce a high degree of determinism in biogeochemical and biotic processes. BioScience 50, 681-692.
| Crossref | Google Scholar |
Medeiros ESF, Arthington AH (2008) The importance of zooplankton in the diets of three native fish species in floodplain waterholes of a dryland river, the Macintyre River, Australia. Hydrobiologia 614, 19-31.
| Crossref | Google Scholar |
Molinari B, Stewart-Koster B, Adame MF, Campbell MD, McGregor G, Schulz C, Malthus TJ, Bunn S (2021) Relationships between algal primary productivity and environmental variables in tropical floodplain wetlands. Inland Waters 11, 180-190.
| Crossref | Google Scholar |
Mosepele K, Kolding J, Bokhutlo T, Mosepele BQ, Molefe M (2022) The Okavango Delta: fisheries in a fluctuating floodplain system. Frontiers in Environmental Science 10, 854835.
| Crossref | Google Scholar |
Ning NSP, Nielsen DL (2011) Community structure and composition of microfaunal egg bank assemblages in riverine and floodplain sediments. Hydrobiologia 661, 211-221.
| Crossref | Google Scholar |
Nunn AD, Tewson LH, Cowx IG (2012) The foraging ecology of larval and juvenile fishes. Reviews in Fish Biology and Fisheries 22, 377-408.
| Crossref | Google Scholar |
O’Hara RB, Sillanpää MJ (2009) A review of Bayesian variable selection methods: what, how and which. Bayesian Analysis 4, 85-117.
| Crossref | Google Scholar |
Petheram C, Hughes J, Stokes C, Watson I, Irvin S, Musson D, Philip S, Turnadge C, Poulton P, Rogers L, Wilson P, Seo L, Pollino C, Ash A, Webster T, Yeates S, Chilcott C, Bruce C, Stratford D, Taylor A, Davies P, Higgins A (2018b) Case Studies for the Northern Australia Water Resource Assessment. A technical report to the Australian Government from the CSIRO Northern Australia Water Resource Assessment, part of the National Water Infrastructure Development Fund: Water Resource Assessments. CSIRO, Canberra, ACT, Australia.
Pettit NE, Bayliss P, Davies PM, Hamilton SK, Warfe DM, Bunn SE, Douglas MM (2011) Seasonal contrasts in carbon resources and ecological processes on a tropical floodplain. Freshwater Biology 56, 1047-1064.
| Crossref | Google Scholar |
Pettit NE, Jardine TD, Hamilton SK, Sinnamon V, Valdez D, Davies PM, Douglas MM, Bunn SE (2012) Seasonal changes in water quality and macrophytes and the impact of cattle on tropical floodplain waterholes. Marine and Freshwater Research 63, 788-800.
| Crossref | Google Scholar |
Pettit NE, Naiman RJ, Warfe DM, Jardine TD, Douglas MM, Bunn SE, Davies PM (2017) Productivity and connectivity in tropical riverscapes of Northern Australia: ecological insights for management. Ecosystems 20, 492-514.
| Crossref | Google Scholar |
Plummer M (2003) JAGS: a program for analysis of Bayesian graphical models using Gibbs sampling. In ‘Proceedings of the 3rd International Workshop on Distributed Statistical Computing (DSC 2003)’, 20–22 March 2003, Vienna, Austria. (Eds K Hornik, F Leisch, A Zeileis) pp. 1–10. (DSC: Vienna, Austria)
Poff NL, Ward JV (1990) Physical habitat template of lotic systems: recovery in the context of historical pattern of spatiotemporal heterogeneity. Environmental Management 14, 629-645.
| Crossref | Google Scholar |
Poff NL, Allan JD, Bain MB, Karr JR, Prestegaard KL, Richter BD, Sparks RE, Stromberg JC (1997) The natural flow regime- a paradigm for river conservation and restoration. BioScience 47, 769-784.
| Crossref | Google Scholar |
Polis GA, Strong DR (1996) Food web complexity and community dynamics. The American Naturalist 147, 813-846.
| Crossref | Google Scholar |
Power ME (1990) Resource enhancement by indirect effects of grazers: armored catfish, algae, and sediment. Ecology 71, 897-904.
| Crossref | Google Scholar |
Pratt OP, Beesley LS, Pusey BJ, Gwinn DC, Keogh CS, Douglas MM (2023) Brief floodplain inundation provides growth and survival benefits to a young-of-year fish in an intermittent river threatened by water development. Scientific Reports 13, 17725.
| Crossref | Google Scholar | PubMed |
Pusey BJ, Jardine TD, Beesley LS, Kennard MJ, Ho TW, Bunn SE, Douglas MM (2021) Carbon sources supporting Australia’s most widely distributed freshwater fish, Nematalosa erebi (Günther) (Clupeidae: Dorosomatinae). Marine and Freshwater Research 72, 288-298.
| Crossref | Google Scholar |
Rayner TS, Pusey BJ, Pearson RG (2009) Spatio-temporal dynamics of fish feeding in the lower Mulgrave River, north-eastern Queensland: the influence of seasonal flooding, instream productivity and invertebrate abundance. Marine and Freshwater Research 60, 97-111.
| Crossref | Google Scholar |
Rejas D, Muylaert K (2010) Bottom–up and top–down control of phytoplankton growth in an Amazonian varzea lake. Fundamental and Applied Limnology 176, 225-234.
| Crossref | Google Scholar |
Rejas D, Declerck S, Auwerkerken J, Tak P, Meester LD (2005) Plankton dynamics in a tropical floodplain lake: fish, nutrients, and the relative importance of bottom–up and top–down control. Freshwater Biology 50, 52-69.
| Crossref | Google Scholar |
Rolls RJ, Leigh C, Sheldon F (2012) Mechanistic effects of low-flow hydrology on riverine ecosystems: ecological principles and consequences of alteration. Freshwater Science 31, 1163-1186.
| Crossref | Google Scholar |
Schaus MH, Vanni MJ, Wissing TE (2002) Biomass-dependent diet shifts in omnivorous gizzard shad: implications for growth, food web, and ecosystem effects. Transactions of the American Fisheries Society 131, 40-54.
| Crossref | Google Scholar |
Shmeleva AA (1965) Weight characteristics of the zooplankton of the Adriatic Sea. Bulletin de L’institut Océanographique de Monaco 65, 1-24.
| Google Scholar |
Sparks RE (1995) Need for ecosystem management of large rivers and their floodplains. BioScience 45, 168-182.
| Crossref | Google Scholar |
Sturrock AM, Ogaz M, Neal K, Corline NJ, Peek R, Myers D, Schluep S, Levinson M, Johnson RC, Jeffres CA (2022) Floodplain trophic subsidies in a modified river network: managed foodscapes of the future? Landscape Ecology 37, 2991-3009.
| Crossref | Google Scholar |
Søndergaard M, Kristensen P, Jeppesen E (1992) Phosphorus release from resuspended sediment in the shallow and wind-exposed Lake Arresø, Denmark. Hydrobiologia 228, 91-99.
| Crossref | Google Scholar |
Thomaz SM, Bini LM, Bozelli RL (2007) Floods increase similarity among aquatic habitats in river–floodplain systems. Hydrobiologia 579, 1-13.
| Crossref | Google Scholar |
Thorp JH, Thoms MC, Delong MD (2006) The riverine ecosystem synthesis: biocomplexity in river networks across space and time. River Research and Applications 22, 123-147.
| Crossref | Google Scholar |
Tockner K, Pennetzdorfer D, Reiner N, Schiemer F, Ward JV (1999) Hydrological connectivity, and the exchange of organic matter and nutrients in a dynamic river–floodplain system (Danube, Austria). Freshwater Biology 41, 521-535.
| Crossref | Google Scholar |
Toruan RL, Dina R, Coggins LX, Ghadouani A (2022) Hydrological regime and fish predation regulate the zooplankton community size structure in a tropical floodplain lake. Water 14, 2518.
| Crossref | Google Scholar |
Townsend SA, Edwards CA (2003) A fish kill event, hypoxia and other limnological impacts associated with early wet season flow into a lake on the Mary River floodplain, tropical northern Australia. Lakes & Reservoirs: Science, Policy and Management for Sustainable Use 8, 169-176.
| Crossref | Google Scholar |
Townsend SA, Padovan AV (2005) The seasonal accrual and loss of benthic algae (Spirogyra) in the Daly River, an oligotrophic river in tropical Australia. Marine and Freshwater Research 56, 317-327.
| Crossref | Google Scholar |
Townsend SA, Przybylska M, Miloshis M (2012) Phytoplankton composition and constraints to biomass in the middle reaches of an Australian tropical river during base flow. Marine and Freshwater Research 63, 48-59.
| Crossref | Google Scholar |
Trevisan GV, Forsberg BR (2007) Relationships among nitrogen and total phosphorus, algal biomass and zooplankton density in the central Amazonia lakes. Hydrobiologia 586, 357-365.
| Crossref | Google Scholar |
Turschwell MP, Stewart-Koster B, King AJ, Pusey B, Crook D, Boone E, Douglas M, Allsop Q, Jackson S, Kennard MJ (2019) Flow-mediated predator–prey dynamics influence fish populations in a tropical river. Freshwater Biology 64, 1453-1466.
| Crossref | Google Scholar |
Vanni MJ (2002) Nutrient cycling by animals in freshwater ecosystems. Annual Review of Ecology and Systematics 33, 341-370.
| Crossref | Google Scholar |
Warfe DM, Pettit NE, Davies PM, Pusey BJ, Hamilton SK, Kennard MJ, Townsend SA, Bayliss P, Ward DP, Douglas MM, Burford MA, Finn M, Bunn SE, Halliday IA (2011) The ‘wet–dry’ in the wet–dry tropics drives river ecosystem structure and processes in northern Australia. Freshwater Biology 56, 2169-2195.
| Crossref | Google Scholar |
Webster IT, Rea N, Padovan AV, Dostine P, Townsend SA, Cook S (2005) An analysis of primary production in the Daly River, a relatively unimpacted tropical river in northern Australia. Marine and Freshwater Research 56, 303-316.
| Crossref | Google Scholar |
Winemiller KO, Montoya JV, Roelke DL, Layman CA, Cotner JB (2006) Seasonally varying impact of detritivorous fishes on the benthic ecology of a tropical floodplain river. Journal of the North American Benthological Society 25, 250-262.
| Crossref | Google Scholar |
Winemiller KO, Montaña CG, Roelke DL, Cotner JB, Montoya JV, Sanchez L, Castillo MM, Layman CA (2014) Pulsing hydrology determines top-down control of basal resources in a tropical river–floodplain ecosystem. Ecological Monographs 84, 621-635.
| Crossref | Google Scholar |
Wold S, Esbensen K, Geladi P (1987) Principal component analysis. Chemometrics and Intelligent Laboratory Systems 2, 37-52.
| Crossref | Google Scholar |