Abundance, size and biomass of long-spined sea urchins (Centrostephanus rodgersii) and red sea urchins (Heliocidaris tuberculata) in New South Wales, Australia
C. Blount


A
B
C
D
E
F
Abstract
Detailed demographic information for the commercially harvested long-spined sea urchins (Centrostephanus rodgersii) and red sea urchins (Heliocidaris tuberculata) on nearshore reefs of New South Wales is unavailable.
This is the first detailed study of abundances and sizes of these sea urchin species on shallow reefs of New South Wales where the fishery operates.
Divers counted sea urchins and measured their sizes in transects in the nearshore mosaic habitat and in extensive barrens habitat at 51 sites along ~800 km of coast.
Centrostephanus was found at most sites in both habitats, whereas Heliocidaris was found at lower densities and at fewer sites. Heliocidaris was rare in the far south and in barrens habitat. Centrostephanus was, on average, smaller in barrens habitat than in the nearshore mosaic habitat, and size structures shifted towards smaller individuals from north to south.
Centrostephanus occurred at higher densities than did Heliocidaris and had a broader distribution along the coast and across the nearshore reefs where the fishery operates.
This study provides a historical baseline for these commercially and ecologically important species. The small biomass of Heliocidaris (~3% that of Centrostephanus) and its restricted distribution suggest it is vulnerable to overfishing.
Keywords: abundance, barrens, biomass, Centrostephanus rodgersii, density, distribution, Heliocidaris tuberculata, macroalgae, size structure.
Introduction
Globally, sea urchins can play a large role in determining the structure of habitats and the local abundance of a broad range of species in both temperate and tropical ecosystems (see Lawrence 2020 for a recent compilation of reviews). The impact of sea urchin herbivory is apparent at seascape scales, where a mosaic of different subtidal habitats can be formed as species respond to grazing pressure. An enormous literature, both experimental and observational, documents the ecological role of sea urchins (Norderhaug and Christie 2009; Filbee Dexter and Scheibling 2014; Lawrence 2020; Steneck 2020). Such is the ecological significance of sea urchin herbivory that there is increasing interest in understanding the extent of habitats created and maintained by them (Filbee-Dexter and Scheibling 2014).
In many places, more is known about the causes of variability in distribution and abundance at local scales than about patterns at larger, biogeographic scales. Historically, on nearshore subtidal reefs, for example, descriptions of patterns and experiments to test hypotheses about those patterns were constrained by the limits of what was possible by scientific divers. At these scales, the focus has been on the patchiness in habitat structure and these patterns have influenced the questions asked by researchers (e.g. Underwood et al. 1991; Andrew 1993; Curley et al. 2003; Connell and Irving 2008).
In recent decades, advances in technology such as towed video and autonomous underwater vehicles have enabled studies of patterns at ever larger scales, often without the loss of granularity usually associated with describing nature at an increasing scale (e.g. Jordan et al. 2010; Marzinelli et al. 2015; Perkins et al. 2015; Edgar et al. 2020; Davis et al. 2020, 2021, 2023). These methods have made it possible to understand general patterns in species abundances across ranges and provide further insights into the potential effects of climate change, fishing and other anthropogenic impacts on species, communities and ecosystems (Vye et al. 2020).
In south-eastern Australia, there have been significant re-distributions of marine species enabled by the climate-driven strengthening of the East Australia Current system (Gervais et al. 2021; Ridgway and Ling 2023). The most consequential of these redistributions has been the southern-range extension of the long-spined sea urchin (Centrostephanus rodgersii, hereafter Centrostephanus), from its historical range in New South Wales into eastern Victoria and Tasmania (Johnson et al. 2005; Ling 2008; Ling et al. 2015; Ling and Keane 2024). Centrostephanus is the dominant herbivore on rocky reefs in temperate south-eastern Australia (Jones and Andrew 1990), creating and maintaining the barrens habitat, i.e. areas with high cover of crustose coralline algae and no foliose algae (see Byrne and Andrew 2020 for review). Where the species has extended into eastern Victoria and Tasmania, it has had profound consequences for seascapes and fisheries for other species as it has radically changed nearshore reefs and negatively affected abalone fisheries (Ling et al. 2015). In New South Wales, by contrast, Glasby and Gibson (2020) estimated that over the past 30 years, on average, there had been small increases in extensive barrens (~20 m2 ha−1 year−1) and, at some sites, there was little change or a reduction in areal extent.
Centrostephanus is most abundant in the barrens, but is also found in large, although more variable, numbers in other areas of nearshore reef (Underwood et al. 1991; Jones and Andrew 1993). As for many species of sea urchin (e.g. Levitan 1991; Pert et al. 2018; Yatsuya and Nakahara 2004), Centrostephanus in areas of barrens grow more slowly and reach smaller sizes than do those in macroalgal habitat (Blount 2004; Ling and Johnson 2009). Further, the size structure of local populations of Centrostephanus in barrens is often, but not always, quite unimodal and often dominated by larger individuals, consistent with rapid early growth and limited recent recruitment of juveniles (Andrew and Underwood 1989, Andrew and O’Neill 2000; Blount 2004; Ling and Johnson 2009). These observations point to important demographic differences between sea urchins in barrens and macroalgal habitats, that may be due to differences in the density of individuals. In shallow water, reefs in New South Wales are a complex mosaic of patches of large brown algae, turfing algae, and areas of crustose coralline algae maintained by Centrostephanus (the ‘mini barrens’ of Shepherd and Edgar 2014). In contrast to the ‘incipient barrens’ described in the transition of reefs to extensive barrens in Tasmania (Ling and Keane 2024), these patches of mini barrens appear stable; their areal extent is limited by sea urchin vulnerability to wave action and the need for daytime shelter from predators (Andrew 1993).
The co-occurring red sea urchin (Heliocidaris tuberculata, hereafter Heliocidaris) has been the subject of reasonably little ecological research. Research has focused on the early life-history stages and the implications of larval attributes on climate-change impacts (Byrne et al. 2013, 2022). Little is known of patterns in the distribution and abundance of the species and the processes that control these patterns (McLaren et al. 2024). Heliocidaris is mostly found in the immediate subtidal zone in rock depressions and crevices, sometimes co-occurring with Centrostephanus (Underwood et al. 1991), but rarely occurring in barrens (Underwood et al. 1991).
Complicating the ecological understanding of how sea urchins affect the seascape and its management is that some sea urchin species are harvested for their roe. Sea urchin fisheries have historically been difficult to manage and have been overfished in many jurisdictions (Andrew et al. 2002). As sedentary invertebrates, they are not well-suited to fisheries management regimes based on broad-scale and model-based assessments, and better results are predicted from fine-scale management based on a good understanding of their spatial structure and the productivity of stocks (Orensanz et al. 2005; Defeo et al. 2016; Aguión et al. 2022).
As interest in large-scale patterns in the distribution and abundance of sea urchins has grown, so too have questions about the persistence and historical trends of Centrostephanus populations (Davis et al. 2023; Kingsford and Byrne 2023). Here, we present a detailed study of the distribution, abundance and population size structure of Centrostephanus and Heliocidaris on shallow, nearshore reefs along ~800 km of the New South Wales coast. Our surveys were structured to be at the shallow depths of the inner margin of nearshore reef, a small portion of the entire reefscape that exists off the coast of New South Wales (e.g. Jordan et al. 2010; Perkins et al. 2015), but where the fishery operates. They were also structured with regard to the five regions along the New South Wales that are used to manage the sea urchin fishery. The surveys we describe were completed 23 years ago and so provide an important historical baseline not only for the ecology of these species and places, but also for assessment of their current stocks and management of their fisheries. Fisheries for both species of sea urchin complicate ecological understanding of how sea urchins interact with the broader seascape and its management in New South Wales (Blount and Worthington 2002; Byrne and Andrew 2020; Chick 2020, 2023). Patterns in the abundance of Heliocidaris provide a counterpoint to the ecological dominance and expanding range of Centrostephanus. Unlike Centrostephanus, Heliocidaris is not known to be a habitat former and does not occur beyond the southern border of New South Wales (Byrne et al. 2022).
Materials and methods
Study sites and sampling methods
Sea urchins were sampled at 51 rocky reef sites in New South Wales between March 2000 and April 2001 (Fig. 1). The potential for short-term changes in density within the sampling period to confound spatial patterns were considered to be unlikely, given prior indications of stable populations of Centrostephanus in New South Wales among months in an analogous sampling regime (Andrew and Underwood 1989). Ten sites were randomly selected and surveyed in each of five regions (commercial sea urchin fishing management regions), except in Region 2 where there were 11 sites. Following Underwood et al. (1991), at each site, on calm days, divers laid two parallel transects, 20 m apart, perpendicular to the shoreward edge of subtidal reefs for a maximum of 50 m, or for a shorter distance if the reef abutted on sand or if the reef dropped away to more than 15-m depth or into extensive barrens (see below). Transects traversed a range of identifiable habitats (we use the term ‘habitat’ throughout the paper to refer to a ‘type’ rather than a ‘place’). Transects began as close to the intertidal zone as prevailing sea conditions allowed (see Supplementary Fig. S1). These transects ran through the various habitats at each site. The shallowest areas of reefs on open coasts in New South Wales are usually covered by a mosaic of foliose and turfing algal habitats, interspersed with small patches of crustose coralline algal barrens (Underwood et al. 1991). Among this mosaic, several taxa can be locally dominant, notably, solitary ascidians (Pyura spp.), the canopy-forming algae Phyllospora comosa, Ecklonia radiata, Sargassum spp. and Durvillaea spp., and turf-forming geniculate coralline algae (Underwood et al. 1991; Jones and Andrew 1993; Andrew and O’Neill 2000; Dye and Blount 2011). We use the term ‘nearshore mosaic’ to describe this composite habitat. This term captures the complexity and scale dependence of descriptions of habitats within this seascape. In all but one site, the areal extent of the nearshore mosaic habitat was sufficient to allow estimates of the abundance of sea urchins to be estimated without a high probability of repeated sampling (Fig. 1). All sites with nearshore mosaic habitat had small patches of barrens habitat at the bases of boulders and in cracks and crevices in the rocky reef.
Map of New South Wales, Australia, showing regions and sites where sea urchins were sampled in the nearshore mosaic and barrens habitats.
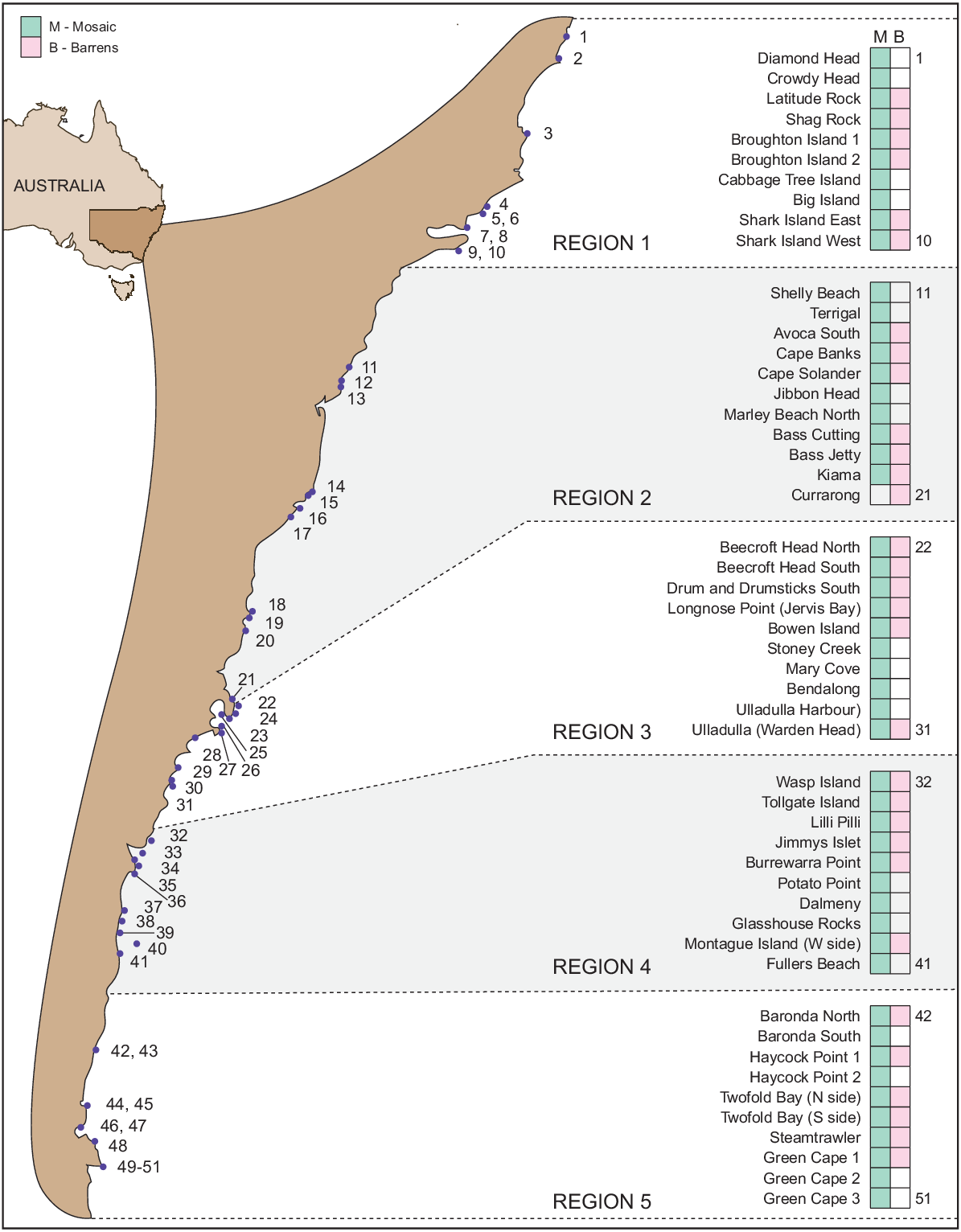
At sites where each main transect did not run into extensive barrens, it ended at 50 m. Alternatively, if the nearshore mosaic extended less than 50 m before it ran into sand, then it ended at the sand edge and the length was recorded. At two-thirds of the 51 sites (Fig. 1), where the nearshore mosaic habitat was bordered by extensive barrens (Underwood et al. 1991; Andrew and O’Neill 2000; Glasby and Gibson 2020), two additional 15-m transects were sampled in this second habitat type. These transects were started within 3 m of the end of the nearshore mosaic and extended in the same direction (see Supplementary Fig. S1). The extensive barrens habitat is a more homogenous and easily defined habitat than is the nearshore mosaic.
Within the nearshore mosaic and barrens habitats, densities of Centrostephanus and Heliocidaris were estimated visually by carefully searching the reef, including in crevices without disturbing the reef. Sea urchins in two size classes (≤50 mm, <2 years old; and >50-mm test diameter) were counted in 12 replicate, 5 × 1-m belt transects perpendicular to the main transects in the nearshore mosaic and in six replicate belt transects in the barrens, if present. Suitable levels of replication in the nearshore mosaic and barrens were determined from pilot studies. The 5 × 1-m belt transects were randomly assigned to one of two divers and sampled at equally spaced positions along the main transects in both the nearshore mosaic and barrens, if present. These belt transects covered a total of 60 m2 in the nearshore mosaic and 30 m2 in the barrens, if present.
At sites where Centrostephanus and Heliocidaris were present, divers measured the test diameter of 100 randomly chosen individuals of both species in the nearshore mosaic habitat and 100 Centrostephanus in barrens habitat. Test diameters were measured by placing Vernier callipers between the spines to reach the test at its widest point.
Data analysis
Densities of total and small (≤50 mm) Centrostephanus or Heliocidaris in 5 × 1-m belts were analysed separately using a four-way (Factor 1, region, fixed, five levels: regions 1–5; Factor 2, habitat, fixed, two levels: nearshore mosaic and barrens; Factor 3, site, random, nested in region, 11 levels; Factor 4, transect, random, nested in site, two levels) univariate permutational ANOVA (PERMANOVA; Anderson 2001), with a type 3 model. Analyses were run using 4999 permutations with Euclidean distance measures (Dorman et al. 2012). PERMANOVA main test was used to test for significant factors and pairwise a posteriori tests were used to compare levels within significant factors. PERMANOVA does not assume normality of errors, but is sensitive to deviations from homoscedasticity. As such, where a key term of interest was significant and where pairwise tests had determined significant differences between one or more groups within a factor, PERMDISP was performed on these to test for homogeneity of multivariate dispersions (Anderson et al. 2006). Where homoscedasticity could not be achieved through transformation, a more conservative significance level of P ≤ 0.01 was considered in the PERMANOVA pairwise tests to account for heteroscedasticity (Zar 1998).
Frequency distributions of sizes for Centrostephanus and Heliocidaris were compared among regions and, for Centrostephanus only, between habitats using Kolmogorov–Smirnov tests with an appropriate level of protection against Type I error for non-orthogonal tests by adjusting α (0.05) using the Dunn–Sidak method.
The relationship between densities of Centrostephanus and Heliocidaris within each region and distance from the shore in the nearshore mosaic and distance from the shoreward edge of barrens was investigated using simple linear regression. Homoscedasticity was confirmed for all regressions by visual inspection of the residuals. Jarque–Bera tests (Jacque and Bera 1987) were performed on the residuals of all regressions and normal distributions were confirmed only for Centrostephanus in barrens.
Biomass of sea urchins in each region was estimated by multiplying the area of habitat with the average number of individuals and the average weight of individuals within each region. Estimates of the area of reef available to Centrostephanus and Heliocidaris within 65 m of nearshore areas and within depths of 20 m in regions were obtained from a publicly available database (NSW Department of Planning and Environment 2012). Although this does not account for the entire reef area occupied by Centrostephanus in New South Wales, which is known to occur as deep as 30 m (Jordan et al. 2010), it covers the area where the fishery operates. The proportion of reef within each region that was nearshore mosaic or barrens was estimated from mapping undertaken by Andrew and O’Neill (2000). The average number of individuals within each region was calculated by multiplying the average density of sea urchins for that region by the appropriate reef area. The average weight of individuals in each region was estimated using global relationships between test diameter (mm) and total weight (g) of individuals for each species. For Heliocidaris, the relationship was as follows:
where R2 = 0.84 and n = 577.
For Centrostephanus, the relationship was as follows:
where R2 = 086 and n = 45.
The standard error (s.e.) of the biomass was estimated using the following equation:
where c is the area of habitat (i.e. m2, and assumed to be known without error), U is the average density of individuals (m−2), V is the average weight of individuals (g), and and are the corresponding variances of U and V. That is, no covariance was assumed between U and V. The area of habitat was assumed to be known without error because we had no formal quantitative estimate of error, but it is likely to be less than 10%.
Results
Density
Centrostephanus was found at all but 1 the 51 sites at densities that ranged between 0.3 and 34.6 individuals per 5 m2 in the nearshore mosaic and between 6.4 and 56.0 individuals per 5 m2 in barrens. There was no significant difference in the density of Centrostephanus among regions, and this was consistent for both habitats (Table 1a, Fig. 2a). The density of total Centrostephanus differed significantly between habitats at some of the sites within regions (as shown by the significant Habitat × Site interaction; Table 1a). Within all regions, barrens generally had higher densities of Centrostephanus than did the nearshore mosaic. Across all five regions, pairwise comparisons of the two habitats at sites where both occurred (Fig. 1) showed that densities in barrens were generally greater than were those in the nearshore mosaic at most sites, but this was not significant in all cases (Fig. 3).
Source of variation | d.f. | MS | Pseudo-F | P(perm) | |
---|---|---|---|---|---|
(a) Centrostephanus | |||||
Region, Re | 4 | 2623.100 | 1.856 | 0.139 | |
Habitat, Ha | 1 | 47,705.000 | 46.023 | <0.001 | |
Site (Re) | 46 | 2012.100 | 12.606 | <0.001 | |
Re × Ha | 4 | 665.140 | 0.642 | 0.637 | |
Transect (Site (Re)) | 51 | 160.680 | 1.969 | <0.001 | |
Ha × Site (Re) | 25 | 1036.500 | 8.569 | <0.001 | |
Ha × Transect (Site (Re)) | 30 | 120.970 | 1.482 | 0.054 | |
Residual | 1410 | 81.606 | |||
Total | 1571 | ||||
(b) Heliocidaris | |||||
Region, Re | 4 | 78.855 | 2.605 | 0.034 | |
Habitat, Ha | 1 | 342.410 | 16.146 | <0.001 | |
Site (Re) | 46 | 42.244 | 23.652 | <0.001 | |
Re × Ha | 4 | 27.125 | 1.279 | 0.303 | |
Transect (Site (Re)) | 51 | 1.761 | 0.485 | 0.999 | |
Ha × Site (Re) | 25 | 21.207 | 13.923 | <0.001 | |
Ha × Transect (Site (Re)) | 30 | 1.523 | 0.420 | 0.998 | |
Residual | 1410 | 3.628 | |||
Total | 1571 |
Significant terms in the PERMANOVA are in bold, P ≤ 0.05.
Mean density (±s.e.) in regions of (a) total Centrostephanus and (b) total Heliocidaris in the nearshore mosaic habitat (green) and barrens (blue). Letters above bars indicate significant differences among regions.
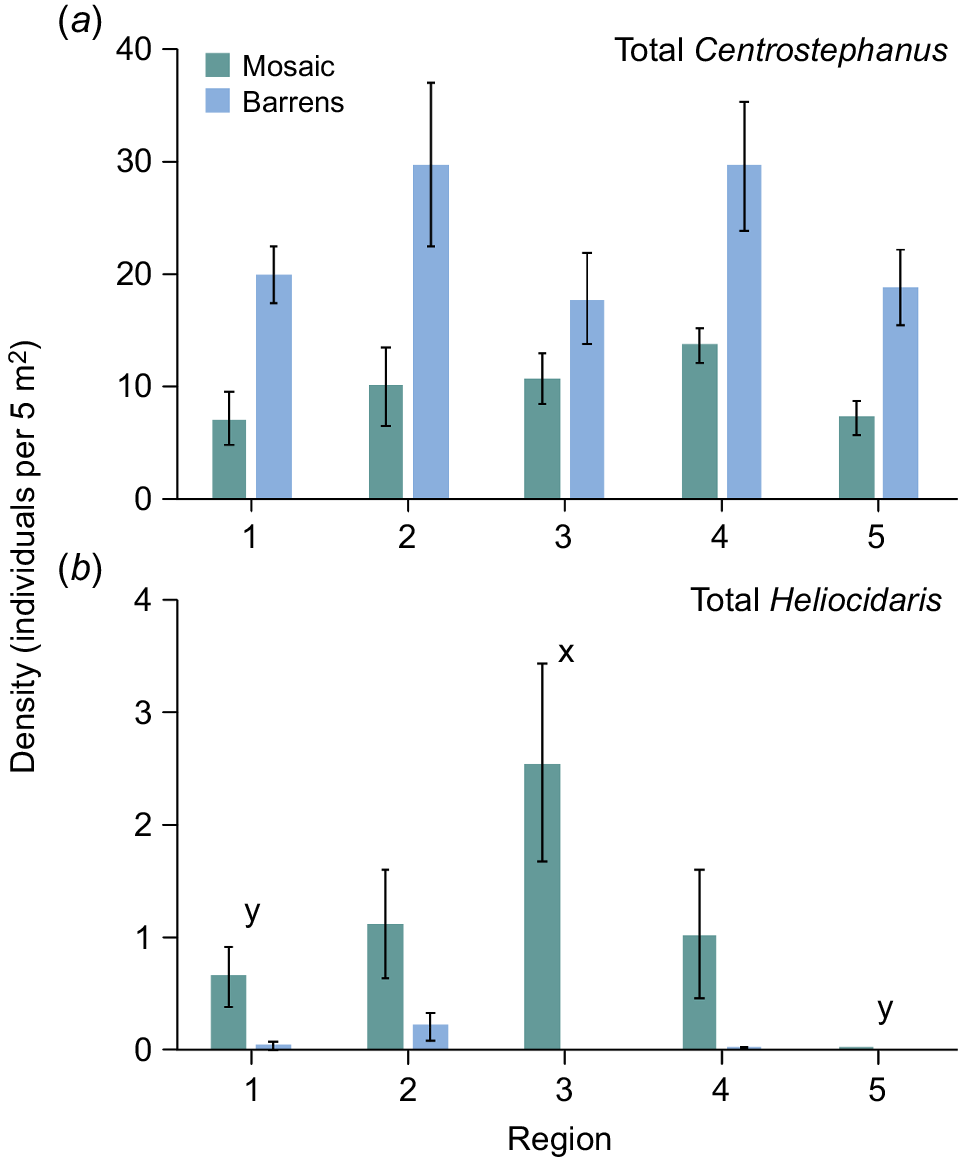
Mean density (±s.e.) at sites of total Centrostephanus in the nearshore mosaic habitat (green) and barrens (blue). Asterisks (*) above columns indicate significant differences between habitats at a site. Note: only sites where both habitats occurred are shown.
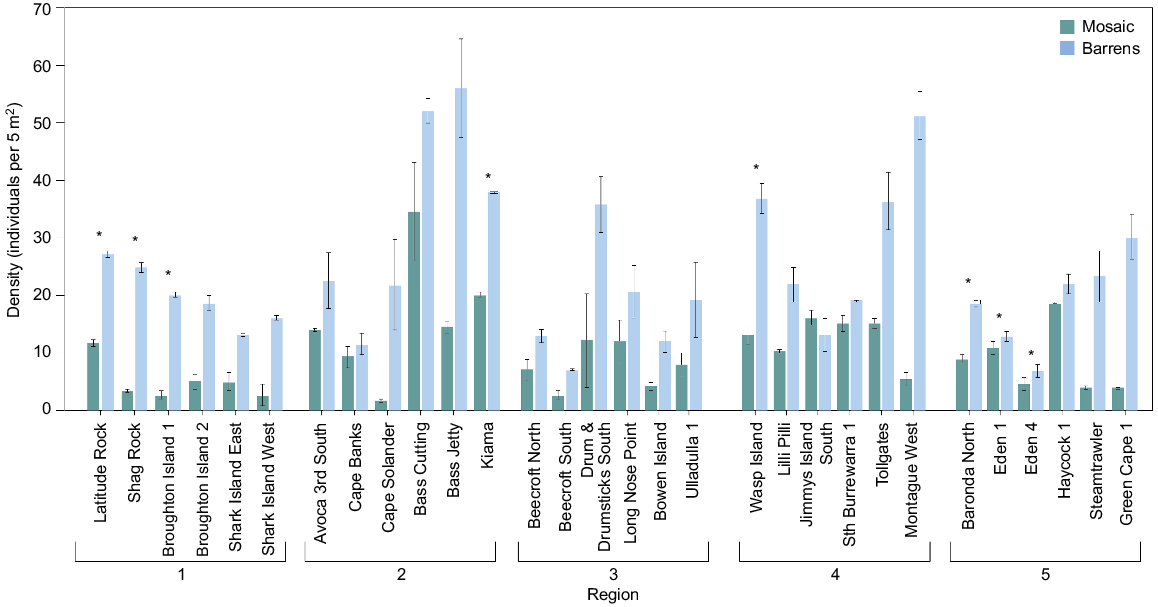
Within Regions 1–4, Heliocidaris was found in the nearshore mosaic habitat at 88% of the sites, with a maximum density of 8.3 individuals per 5 m2, but was present at only 20% of the sites sampled in Region 5, and at very low densities (<0.1 individuals per 5 m2). Heliocidaris was rarely found in barrens (i.e. at only six sites in total) and when found, only at low densities (<1 individual per 5 m2). There were significant differences in the density of total Heliocidaris among regions and this was consistent between habitats (Table 1b, Fig. 2b). Average densities of total Heliocidaris in Region 3 (habitats combined) were significantly greater than in Regions 1 and 5 (Table 1b, Fig. 2b). As for Centrostephanus, the density of total Heliocidaris differed significantly between habitats at some sites within regions (as shown by the significant Habitat × Site interaction) (Table 1b). In all regions, densities in the nearshore mosaic were significantly greater than in barrens at many sites (Fig. 4), although pairwise comparisons were unable to resolve which sites differed significantly between habitats. Within all regions, pairwise comparisons indicated significant differences in densities of Heliocidaris within barrens or the nearshore mosaic among many sites.
Mean density (±s.e.) at sites of total Heliocidaris in the nearshore mosaic habitat (green) and barrens (blue).
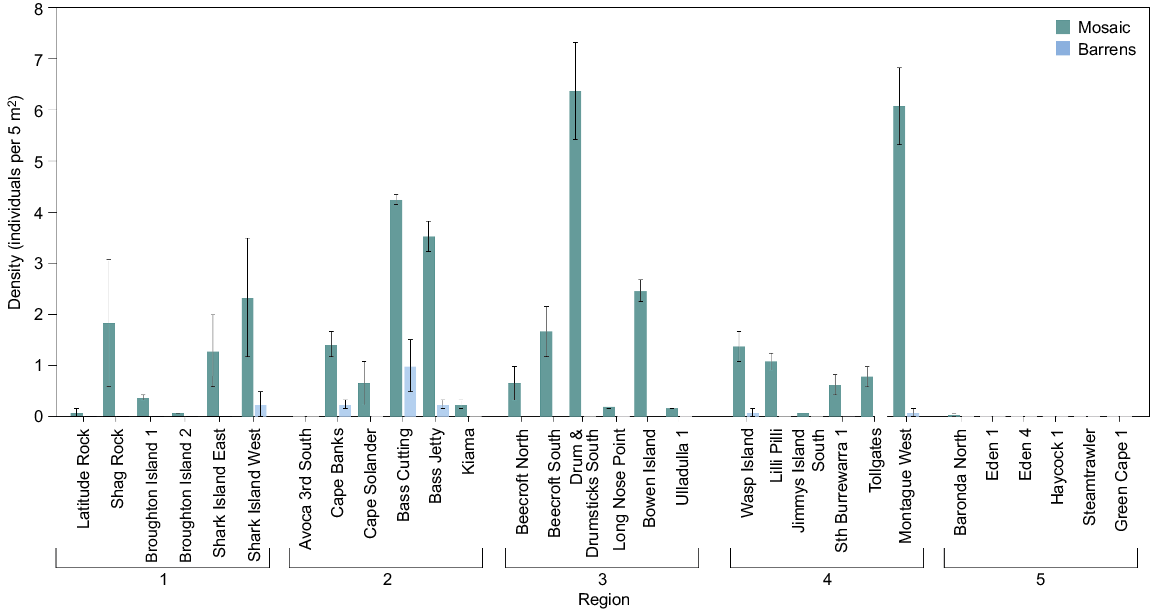
Densities of small (≤50 mm) individuals ranged between 0.03 and 4.9 per 5 m2 at sites for Centrostephanus and between 0.03 and 0.5 per 5 m2 for Heliocidaris. Small Centrostephanus were observed at all but three sites and there were significant differences between habitats for some sites and vice versa, among some sites within the mosaic or barrens (Table 2a, Fig. 5a). By contrast, small Heliocidaris were not observed at 26 sites (including all 10 sites in Region 5) and densities of small Heliocidaris differed between habitats for some regions and vice versa (Table 2b, Fig. 5b). Pairwise tests indicated that there were significantly greater densities of small Heliocidaris in the nearshore mosaic than in barrens in Regions 1–4 (Fig. 5b). Further, in the nearshore mosaic, pairwise tests showed that densities of small Heliocidaris in Region 3 were greater than for all other regions (Fig. 5b). The density of small Heliocidaris differed significantly between habitats at some sites (as shown by the significant Habitat × Site interaction) (Table 2b). Pairwise tests were unable to resolve where differences occurred.
Source of variation | d.f. | MS | Pseudo-F | P(perm) | |
---|---|---|---|---|---|
(a) Centrostephanus | |||||
Region, Re | 4 | 33.149 | 1.473 | 0.210 | |
Habitat, Ha | 1 | 6.446 | 0.330 | 0.595 | |
Site (Re) | 46 | 30.922 | 4.679 | <0.001 | |
Re × Ha | 4 | 4.611 | 0.236 | 0.926 | |
Transect (Site (Re)) | 51 | 6.647 | 1.751 | <0.001 | |
Ha × Site (Re) | 25 | 19.553 | 2.503 | 0.011 | |
Ha × Transect (Site (Re)) | 30 | 7.813 | 2.058 | 0.011 | |
Residual | 1410 | 3.797 | |||
Total | 1571 | ||||
(b) Heliocidaris | |||||
Region, Re | 4 | 0.264 | 1.791 | 0.136 | |
Habitat, Ha | 1 | 2.604 | 27.547 | <0.001 | |
Site (Re) | 46 | 0.175 | 5.080 | <0.001 | |
Re × Ha | 4 | 0.407 | 4.310 | <0.001 | |
Transect (Site (Re)) | 51 | 0.034 | 0.394 | 1.0000 | |
Ha × Site (Re) | 25 | 0.095 | 2.876 | <0.001 | |
Ha × Transect (Site (Re)) | 30 | 0.033 | 0.383 | 0.998 | |
Residual | 1410 | 0.086 | |||
Total | 1571 |
Significant terms in the PERMANOVA are in bold, P ≤ 0.05.
Mean density (±s.e.) in regions of (a) small Centrostephanus and (b) small Heliocidaris in the nearshore mosaic habitat (green) and barrens (blue). Letters above bars indicate significant differences among regions (within the mosaic) or between habitats at a region.
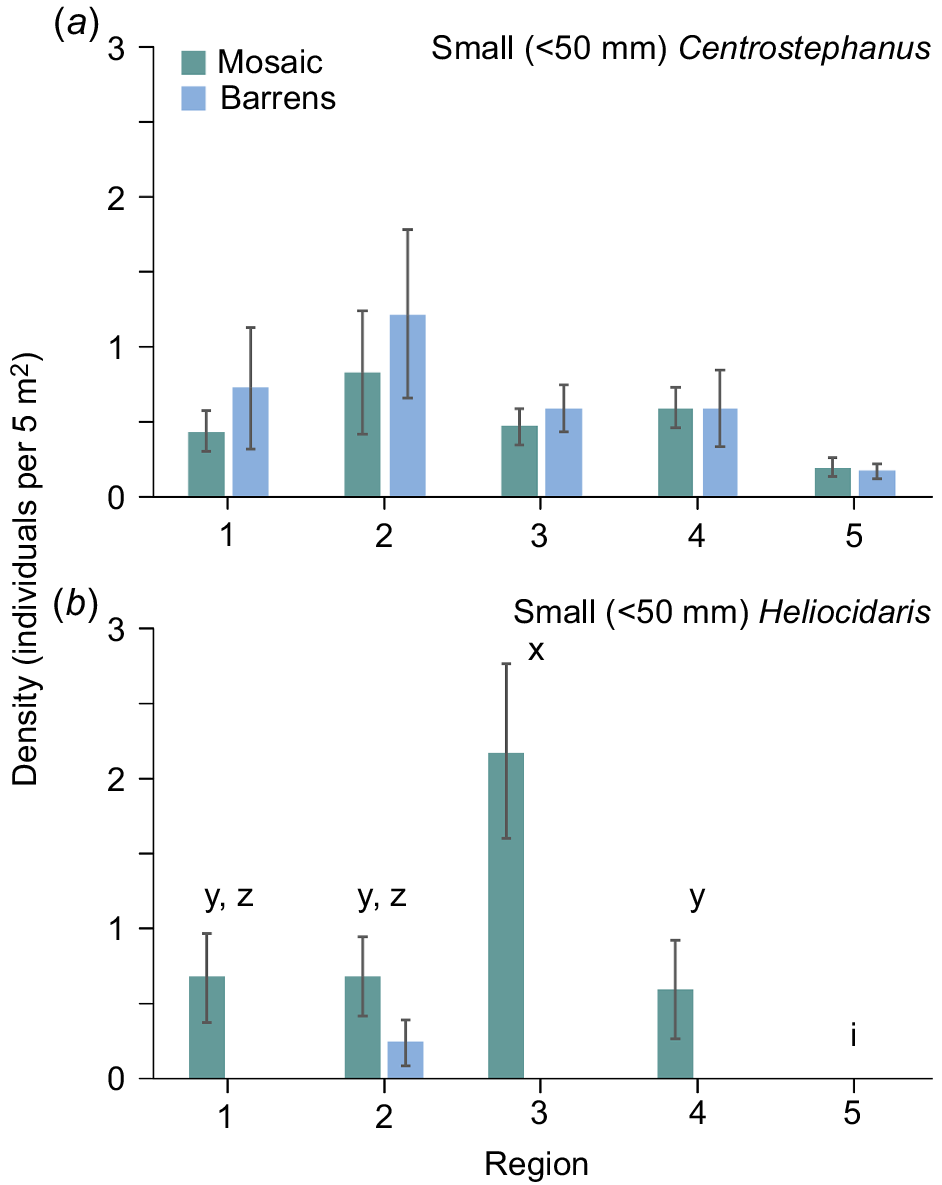
In the nearshore mosaic, there were significant relationships between density and distance from the shore for Centrostephanus in Regions 1 and 3 and for Heliocidaris in all regions, with the density of Centrostephanus increasing away from shore and the density of Heliocidaris decreasing (Table 3). However, although statistically significant linear relationships existed, in all instances the small R2 (range of 0.03–0.06 for Centrostephanus and 0.01–0.14 for Heliocidaris) indicated that only a very small amount of the overall variation in density can be explained by the distance from the shore. In barrens, there was no relationship between the density of Centrostephanus and distance from the shoreward edge (Table 3).
Region | Centrostephanus | Heliocidaris | |||||||||
---|---|---|---|---|---|---|---|---|---|---|---|
d.f. | F | P | R2 | Slope | d.f. | F | P | R2 | Slope | ||
Nearshore mosaic | |||||||||||
R1 | 1, 214 | 23.12 | <0.001 | 0.06 | + | 1, 214 | 23.12 | <0.001 | 0.10 | − | |
R2 | 1, 239 | 1.96 | 0.163 | 0.01 | na | 1, 239 | 21.70 | <0.001 | 0.08 | − | |
R3 | 1, 239 | 3.20 | 0.075 | 0.01 | na | 1, 239 | 31.14 | <0.001 | 0.12 | − | |
R4 | 1, 239 | 6.58 | 0.011 | 0.03 | + | 1, 239 | 37.15 | <0.001 | 0.14 | − | |
R5 | 1, 239 | 0.28 | 0.597 | <0.01 | na | 1, 239 | 2.48 | 0.11 | 0.01 | na | |
Barrens | |||||||||||
R1 | 1, 70 | 0.88 | 0.352 | 0.01 | na | nd | nd | nd | nd | nd | |
R2 | 1, 70 | 0.10 | 0.748 | <0.01 | na | nd | nd | nd | nd | nd | |
R3 | 1, 70 | 0.05 | 0.833 | <0.01 | na | nd | nd | nd | nd | nd | |
R4 | 1, 70 | <0.01 | 0.949 | <0.01 | na | nd | nd | nd | nd | nd | |
R5 | 1, 70 | 0.10 | 0.754 | <0.01 | na | nd | nd | nd | nd | nd |
Significant terms in the regression are in bold, P ≤ 0.05. na, not applicable; nd, no data.
Size–frequency distributions
Within all regions, the size–frequency distributions of Centrostephanus in the nearshore mosaic and barrens were generally unimodal (Fig. 6). In all regions, the distributions for Centrostephanus were significantly different between habitats (K–S test, D > Dcrit). This is seen in the smaller modal size of Centrostephanus in barrens than in the nearshore mosaic, as well as the greater proportion of larger animals in the nearshore mosaic across all regions (Fig. 6). There were also significant differences in size–frequency distributions for Centrostephanus in nearshore mosaic and barrens habitats among regions. For the nearshore mosaic, distributions among regions were all significantly different, apart from Regions 1 and 4, Regions 2 and 3, and Regions 4 and 5; for barrens, they were significantly different from one another apart from Regions 1 and 3, and Regions 4 and 5 (K–S test, D > Dcrit, n-adjusted, Fig. 6). These differences were apparent as a general shift in the distributions to smaller individuals (in each habitat) from north to south. The exception was the distribution of Centrostephanus in barrens in Region 2, which included larger proportions of smaller-sized animals than for any other region.
Percentage frequency distributions of sizes of (a) Centrostephanus and (b) Heliocidaris in the nearshore mosaic habitat (green) and barrens (blue) by region.
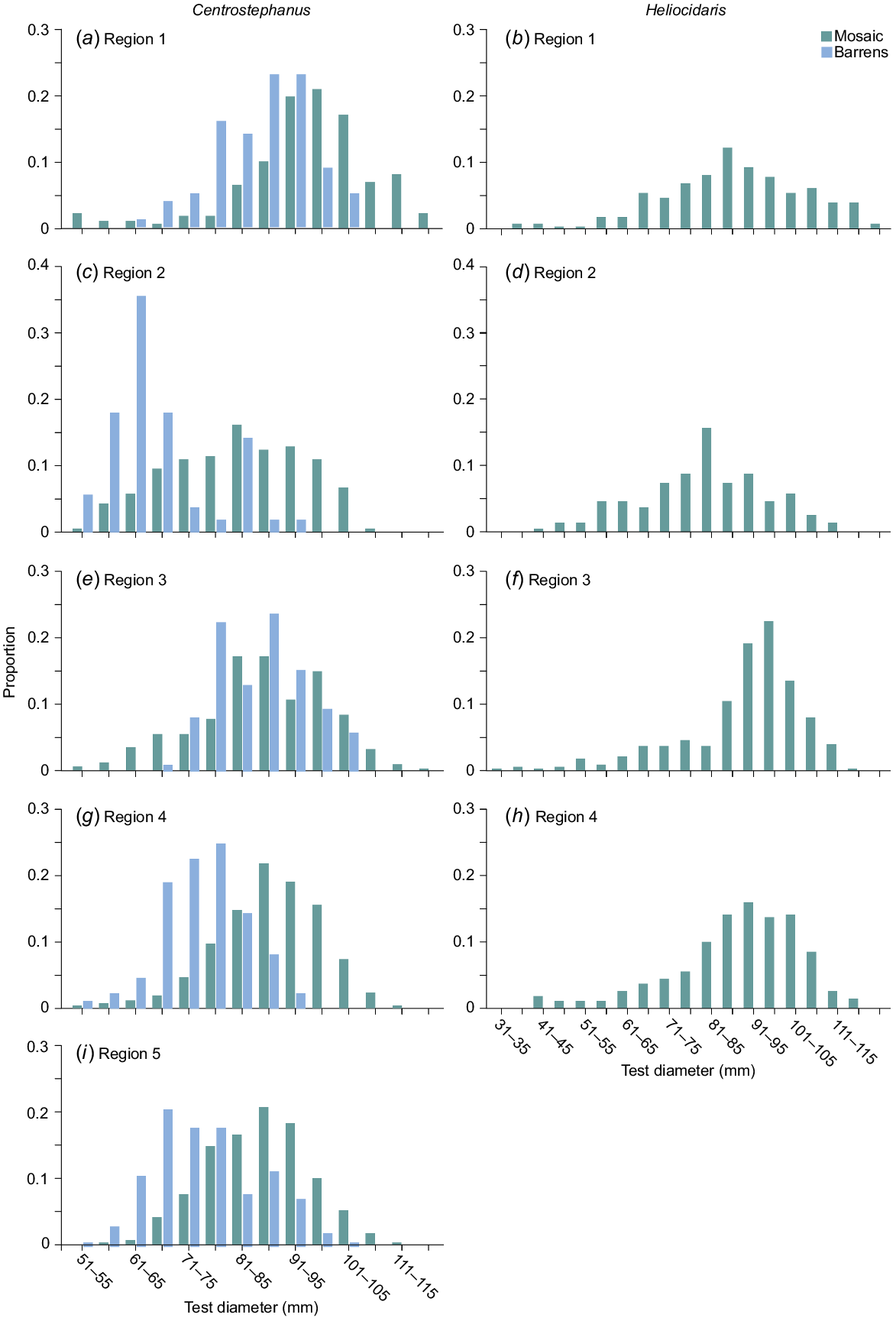
For Heliocidaris, the size–frequency distributions in the nearshore mosaic were significantly different from one another, apart from Regions 1 and 4, and Regions 2 and 4 (K–S test, D > Dcrit, n-adjusted), but there was no obvious trend from north to south (Fig. 6).
Biomass
The areas of the nearshore mosaic and barrens were generally similar in Regions 3 and 5, whereas the nearshore mosaic represented a greater proportion of total reef than did barrens in Region 1 and barrens represented a greater proportion than the nearshore mosaic in Regions 2 and 4. Within 65 m of shore, the total biomass for Centrostephanus was 22,118 tonnes (Mg) in the nearshore mosaic and 25,974 Mg in barrens (Table 4). Biomass was greatest in both habitats in Region 2, as was the reef area. In the nearshore mosaic, biomass and reef area were lowest in Region 3 and in barrens, these were least in Region 1. The total biomass for Heliocidaris in the nearshore mosaic was 1781 Mg (Table 4). As for Centrostephanus, biomass of Heliocidaris in the nearshore mosaic was greatest in Region 2, approximately double that of Region 3 and triple that of Regions 1 and 4.
Item | Symbol | Centrostephanus | Heliocidaris | |||||||||
---|---|---|---|---|---|---|---|---|---|---|---|---|
R1 | R2 | R3 | R4 | R5 | R1 | R2 | R3 | R4 | R5 | |||
Mosaic | ||||||||||||
Density (individuals m−2) | A | 1.45 (0.49) | 2.02 (0.70) | 2.16 (0.46) | 2.76 (0.30) | 1.47 (0.32) | 0.13 (0.05) | 0.22 (0.10) | 0.51 (0.18) | 0.21 (0.12) | 0.01 (0.01) | |
Mean weight (kg) | B | 0.44 (0.02) | 0.35 (0.03) | 0.37 (0.02) | 0.39 (0.01) | 0.39 (0.01) | 0.37 (0.03) | 0.27 (0.04) | 0.32 (0.03) | 0.32 (0.03) | nd | |
Reef area (ha) | C | 428 | 1505 | 259 | 353 | 484 | 428 | 1505 | 259 | 353 | 484 | |
Biomass (Mg) | A × B × C | 2708 (2933) | 10,725 (12,024) | 2063 (1426) | 3815 (1354) | 2756 (1912) | 207 (178)) | 917 (308)) | 421 (242) | 236 (218) | 3 (9) A | |
Barrens | ||||||||||||
Density (individuals m−2) | E | 4.01 (0.53) | 5.95 (1.46) | 3.57 (0.82) | 5.96 (1.16) | 3.80 (0.67) | 0.01 (0.01) | 0.04 (0.07) | 0 | 0.01 (0.19) | 0 | |
Mean weight (kg) | F | 0.33 (0.02) | 0.23 (0.04) | 0.33 (0.02) | 0.27 (0.02) | 0.28 (0.02) | 0.37 (0.03) | 0.27 (0.04) | 0.32 (0.03) | 0.32 (0.03) | 0.32 (0.03) | |
Reef area (ha) | G | 183 | 632 | 248 | 442 | 442 | 183 | 632 | 248 | 442 | 442 | |
Biomass (Mg) | E × F × G | 2420 (770) | 8721 (6568) | 2899 (1681) | 7217 (3655) | 4781 (2371) | 6 (76) B | 74 (117) B | 0 | 8 (272) B | 0 |
Discussion
Patterns in density and size of sea urchins
The reasonably consistent densities of Centrostephanus observed along ~800 km of the New South Wales coast differ from those in a recent study where density generally decreased with latitude (Davis et al. 2020). A number of reasons may underlie these differences, including different methods used (diver-based surveys versus towed-camera surveys), changes in the density of Centrostephanus between sampling periods or different areas sampled across the reef (see below).
In this study, we observed average densities of Centrostephanus in the nearshore mosaic to be approximately half of the densities in barrens; this pattern is consistent with other observations for this species in Tasmania where the nearshore reef is dominated by macroalgae (Johnson et al. 2005; Ling and Johnson 2009) and for other species of sea urchins (Comely and Ansell 1988; Fagerli et al. 2015). The similar density of small individuals (equivalent to ≤2 year olds, Blount 2004) we observed in barrens and the nearshore mosaic in most regions suggests that recruitment is not the cause of this pattern.
Heliocidaris, in contrast to Centrostephanus, was restricted to the nearshore mosaic habitat, with very few being encountered in barrens (Fig. 2b). The greatest densities of Heliocidaris occurred from Jervis Bay to Ulladulla (Region 3), where the mean density was significantly greater (approximately double) than for the adjacent regions to the north and south. Densities of Heliocidaris in Regions 2 to 4 were significantly greater than for the northern-most (Region 1) and southern-most (Region 5) regions. In some regions, there were significantly greater densities of small Heliocidaris in the nearshore mosaic than in barrens, suggesting differences in recruitment between the habitats. Our data appear to represent the range limits of this species’ general distribution, as supported by recent Reef Life Survey data, although we note that Heliocidaris has been recorded at some offshore island sites further north than our sampling extent (Reef Life Survey data, 2023, see https://reeflifesurvey.com/survey-data/, accessed 1 May 2023).
A recent study of eight sea urchin species showed a close match between larval thermal tolerance and realised thermal niche (Collin et al. 2021), indicating that the thermal tolerance of the pelagic phase may be more important in determining distributions than is thermal tolerance of adults. Indeed, in the laboratory, Byrne et al. (2022) matched the optimal temperature range for survival of embryos and larvae of Heliocidaris with the realised thermal niche of its adult distribution (i.e. sea-surface temperature range of 13.8–26.5°C). The small latitudinal range of Heliocidaris, when coupled with its narrow nearshore distribution within the shallow nearshore mosaic habitat (i.e. generally <5-m water depths), means that this species probably has one of the smallest latitudinal distributions of sea urchins with planktonic-feeding larvae.
At the smaller scale of sites within regions, estimates of densities of Centrostephanus in barrens ranged between 6.4 and 56.0 individuals per 5 m2 and were comparable to previous estimates for this species in barrens (Andrew and Underwood 1989; Andrew and O’Neill 2000). Consistent with regional patterns, at sites, mean densities of Centrostephanus in barrens (ranging between 6.4 and 56.0 individuals per 5 m2) were generally higher than for the nearshore mosaic (ranging between 0.29 and 34.6 individuals per 5 m2), but this was significant only at some of the sites. Interestingly, average densities of Centrostephanus in the nearshore mosaic at two sites (4.0 individuals m−2 at Bass Cutting and 6.9 individuals m−2 at Kiama), both in Region 2, were at or above the threshold (~4 individuals m−2) at which Ling et al. (2009) considered that a phase shift is triggered from macroalgal habitat to barrens. Although our sampling may have coincided with the beginnings of such a phase shift, a review of recent aerial imagery of these sites did not find evidence that a phase shift had occurred (C. Blount, unpubl. data). It is more likely that complex topography at some sites supports densities >4 individuals m−2 without triggering such a shift. In no instance were densities in barrens below the threshold (~0.5 individuals m−2) at which Ling et al. (2009) considered that a reverse phase shift from barrens to macroalgal habitat would occur.
Differences in availability of shelter may explain why densities of Centrostephanus in barrens were approximately double those in the nearshore mosaic. In New South Wales, barrens habitat occurs down to 27-m water depth, but is most common in the 7–15-m depth range, reflecting the cross-shelf or depth distribution of Centrostephanus (Jordan et al. 2010; Perkins et al. 2015). Apart from Ecklonia forests that may occur at depth, most of the macroalgal communities within the nearshore mosaic of the shallow reefs of New South Wales are on shallower, wave-exposed reefs rather than barrens (Underwood et al. 1991; Andrew and O’Neill 2000).
For Heliocidaris, the pattern was opposite to that observed for Centrostephanus, with larger total densities in the nearshore mosaic than in barrens. There was also a wide range of densities in the nearshore mosaic at sites within regions. Heliocidaris was more common near the centre of its distribution and absent at most sites in the far south of New South Wales (Region 5) and at many sites in the most northern region, sharply contrasting with Centrostephanus. Even in Region 3, in the centre of its distribution, although large accumulations of Heliocidaris were observed at some sites, there were few individuals at many others.
Peaks in the size structures of Centrostephanus in barrens were consistently at smaller test diameters than for the nearshore mosaic. This trend of smaller peaks of Centrostephanus in barrens than in other habitats is also found in Tasmania where it is attributed to slower somatic growth due to lower food (macroalgae) supply (Ling and Johnson 2009). As seen in other species, small size and slower growth may also be due to density-dependent competition among individuals for food (e.g. Himmelmann 1978; Sivertsen and Hopkins 1995). Despite differences in densities and size structures in adjacent habitats, a similar age structure of Centrostephanus was observed in Tasmania, where it was suggested to indicate greater rates of settlement or post-settlement survivorship in barrens (Ling and Johnson 2009). Blount et al. (2024) found the same patterns for age structures down to 10-m water depths for Centrostephanus in New South Wales, and, in this study, down to 15-m water depths, we did not find any evidence of different rates of settlement or post-settlement survivorship between habitats, at least in analysis of abundances of small individuals (≤50 mm, i.e. ≤2 year olds, see Andrew 1991).
The peaks in size structures of Centrostephanus and the distributions tended to shift towards smaller animals with an increasing latitude, except for Region 2, which appears to be an anomaly. In general, with further separation among regions, the distributions tended to differ significantly from one another. Slower somatic growth of Centrostephanus associated with generally colder water temperatures with an increasing latitude may be responsible for the shift in distributions (C. Blount et al., unpubl. data). Although the size structure of populations of Heliocidaris also tended to differ significantly from one another, there was no latitudinal gradient.
Developing models for distributions and population dynamics of sea urchins in New South Wales
Although not designed to test hypotheses concerning species’ distribution patterns within their range, the contrasting patterns we observed in the distributions of Centrostephanus and Heliocidaris offer the basis for some commentary. Centrostephanus is abundant and widely distributed across an expansive geographic range, whereas Heliocidaris has a much more restricted latitudinal distribution within the centre of its geographic range (see Byrne et al. 2022) and is relatively uncommon along the coast. In addition, Heliocidaris is found within a narrow depth range in the immediate subtidal zone. The larval stages of the two species also differ markedly, with the larva of Centrostephanus being the 2-armed echinopluteus characteristic of diadematids, which appears designed for long-distance dispersal, whereas the larva of Heliocidaris is the more typical eight-armed echinopluteus (Soars et al. 2009; Soars and Byrne 2015). Centrostephanus has a longer larval life (1–5 months) than does Heliocidaris (~3–5 weeks) (Byrne et al. 2001; Huggett et al. 2005; Mos et al. 2020). Moreover, the contrasting latitudinal poleward range of the two species is also influenced by the difference in the larval thermal tolerance, with the greater cool tolerance of Centrostephanus facilitating larval success poleward and range expansion under climate change (Byrne et al. 2022).
Given these patterns, Heliocidaris distribution is broadly consistent with the ‘abundant centre’ hypothesis (Whittaker 1956; Brown 1984), which holds that species will be most abundant at the centre of their distribution, where presumably environmental conditions are best suited to its niche requirements, among other causal hypotheses (Sagarin and Gaines 2002). By contrast, Centrostephanus was consistently abundant across the sites sampled here and might be considered a generalist species (Byrne and Andrew 2020). There is little evidence of greater abundance at the centre of its historical range. Further, its poleward range extension provides little support for alternative models of distribution that predict, for example, that its abundance would become more patchy at the margins of its range. More generally, the strong and changing environmental gradient along the 800 km of coastline sampled in this study offers a useful and largely underutilised opportunity to test hypotheses concerning the distribution and abundance of species (Sagarin and Gaines 2002).
The size structures of Centrostephanus recorded in the present study are similar to those reported elsewhere in New South Wales (Andrew and Underwood 1989; Andrew and O’Neill 2000). Large Centrostephanus in these populations are likely to be up to 27 years old (Blount et al. 2024). At a regional level, we found little evidence of the bimodality in population structures that has been found in several species of echinoid, which can be due to either ontogenetic shifts in microhabitat and associated changes in vulnerability to predation (e.g. Strongylocentrotus franciscanus, Tegner and Dayton 1981; Evechinus chloroticus, Andrew and Choat 1982) or contrasting periods of very high and very low recruitment (e.g. Strongylocentrotus droebachiensis, Fagerli et al. 2015). Although we did find evidence of significant small-scale variation in recruitment among sites, as did Andrew and Underwood (1989), it did not appear to be substantial enough to affect size structures. Others have suggested that a long reproductive lifespan and abundant gamete production with occasional successful recruitment events are sufficient to maintain populations that are dominated by large, old individuals (Ebert 1982, 1983; Sivertsen 1997; Vadas et al. 2002).
The shallow boundaries between extensive barrens and nearshore mosaic habitats within New South Wales often align with discontinuities in reef substratum type (Jordan et al. 2010). The wave energy and sweeping motion of large brown seaweeds that can create an abrasive ‘whiplash’ effect on the benthos appear to restrict Centrostephanus from reaching densities in the shallows that would be sufficient to create extensive barrens there (Andrew 1993; Andrew and Byrne 2001; see also Konar 2000). This study was not designed to estimate whether the fine-scale distribution of barrens has expanded or contracted within New South Wales. We cannot, therefore, confirm anecdotal evidence that the species has spread within or among reefs in the State in recent decades and since Glasby and Gibson’s (2020) investigation that compared historical estimates with those in the 2010s. Within the broadscale picture of relative stability described by Glasby and Gibson (2020), there was considerable variability in changes in local extent of barrens in nearshore shallow reefs. Further, the cumulative effect of small annual percentage increases in extent of barrens over decades may result in significant expansion in area in some areas of the coast. Such increases would go largely unnoticed in deeper areas of reef, below the reach of aerial imagery and often into more turbulent shallow areas.
Given the management implications of changes in the extent of barrens, there is an urgent need to take advantage of new survey technologies to establish baselines on the areal extent of barrens. Such baselines should extend historical comparisons (Andrew and O’Neill 2000; Perkins et al. 2015; Glasby and Gibson 2020) and establish new baselines for deeper reefs. We speculate that the period of El Niño years observed in 2014–16, and the associated unusually large storm (Mortlock et al. 2017; J. Smythe, pers. comm.) marked a significant event in the recruitment, and consequent local expansion on Centrostephanus in New South Wales.
A key finding of this study was that, regardless of spatial scale, Heliocidaris exists at much lower densities than does Centrostephanus. Although both species grow to similar sizes and, therefore, probably have similar requirements for food and shelter, it is unlikely that interspecific competition with Centrostephanus is the cause of Heliocidaris’ lower densities, at least in the nearshore mosaic. At sites where they co-exist in the nearshore mosaic and where densities of Heliocidaris were greatest, we found no evidence of lower densities of Centrostephanus that may indicate reduced competition at these places. Notwithstanding this, there may be competition occurring in barrens, given that the densities of Centrostephanus are greater and Heliocidaris rarely occurs. Alternatively, there could also be differences in predation levels of Heliocidaris between the nearshore mosaic and barrens habitats or there may be a preference for Heliocidaris to recruit to shallows or the nearshore mosaic.
Recruitment to mainly shallow waters (≤4 m) has been observed for other sea urchins, for example, Anthocidaris crassispina (Lau et al. 2011) and Paracentrotus lividus (Ouréns et al. 2013). Ontogenetic migration is another explanation and, although this has been observed for juveniles of A. crassispina (Lau et al. 2011), P. lividus (Ouréns et al. 2013) and Loxechinus albus (Molinet et al. 2013), migration is generally always from shallow to deeper areas rather than vice versa. Notwithstanding this, we did find some evidence, although very limited, to support a shoreward migration for Heliocidaris, given densities decreased with distance from the shore in the nearshore mosaic in Regions 1, 3 and 4. An alternative explanation for the generally lower density of Heliocidaris relative to Centrostephanus could be differences between species in their recruitment potential, source–sink relationships, or larval or early post-settlement mortality. However, very little is known about any of these processes aside from their larval biology and planktonic larval duration (see above).
Implications for fisheries
The limited distribution and low abundance of Heliocidaris provides an important and cautionary baseline for the fishery for this species. The fishery expanded rapidly in the early years of the 2000s, leading to significant depletions of populations on local scales and probable serial depletion in patterns of fishing (Worthington and Blount 2003; Chick 2020). The fishery is managed with an annual total allowable commercial catch, introduced in 2002, of 60 Mg and reduced to 30 Mg in 2023, spatially managed catch caps, as size limit (Chick 2020), and a series of spatial closures across the domain of the fishery. The total allowable commercial catch has never been harvested (Chick 2023). Aside from the surveys reported here, and descriptions of its reproductive and larval ecology (e.g. Byrne et al. 2022), there is inadequate information available for managers to proceed with anything other than highly precautionary management (NSW Total Allowable Fishing Committee 2021). Notably missing are time series of abundance, estimates of growth, longevity and recruitment dynamics that would enable recommendations concerning the productivity of stocks and the sustainability of harvests. Globally, the history of over-exploitation of sea urchin fisheries with such large deficits in information is poor (Andrew et al. 2002). The precautionary approach, as currently used by managers of the fishery, is to maintain a small total catch partitioned by regions according to historical local biomass, and retain multiple closed areas within regions that are understood to maintain pre-fishing densities in many places. The surveys reported here provide a unique 24-year-old baseline for further empirical assessments of the fishery.
By contrast, the Centrostephanus harvest in New South Wales, although gradually increasing from ~50 Mg liveweight in 2002 to ~254 Mg in 2023 (Chick 2023), represents a very small percentage of estimated stocks (i.e. <1%, noting that our surveys were conducted only in the fished area and not the entire reefscape), and there is considerably more information available about the biology and ecology of the species (Worthington and Blount 2003, see Byrne and Andrew 2020 for recent review). Further, a large proportion, possibly the majority, of available stocks are found in habitats with poor roe recovery, and are not fished (Blount and Worthington 2002; Blount et al. 2017). This circumstance offers great scope for low-risk experimentation and expansion of the fishery. It is reasonable to conclude that neither sustainability risk, consistent with the NSW Fisheries Management Act 1994, nor availability of information are impediments to innovation. The rate-limiting step in the management cycle is far more likely to revolve around the social dimensions of the fishery, including the appetite for true collective action, resolution of access and property right issues, capacity to implement innovative experimental fishery regimes, and the profitability of fishing enterprises (Blount et al. 2017; Byrne and Andrew 2020; Andrew 2022).
Both fisheries share risks external to the dynamics of the fishery, notably climate change-induced change in the dynamics of populations. Environmental changes in the oceanography of the New South Wales coast are already evident and predicted to become more severe (Davis et al. 2023). Such systemic changes further reduce the applicability of centralised management regimes implemented over broad geographic scales, and reinforce the importance of empirical surveys of patterns, such as those described in the present study, in understanding ecological processes influenced by fishing.
Data availability
Data used in the analyses presented here are available from the NSW Department of Primary Industries and Regional Development (Fisheries) upon reasonable request by a data licencing agreement.
Declaration of funding
This research was primarily funded by the Australian Government Fisheries Research and Development Corporation (projects 1999/128 and 2021/060). The New South Wales Department of Primary Industries and Regional Development (Fisheries) provided financial support to projects 1999/128 and 2021/060.
Acknowledgements
The authors thank Ben Stewart, Ron Avery and Peter Gibson for assisting with field work, and Eleanor McNeill for preparing the graphics.
References
Aguión A, Ojea E, García-Flórez L, Cruz T, Garmendia JM, Davoult D, Queiroga H, Rivera A, Acuña-Fernández JL, Macho G (2022) Establishing a governance threshold in small-scale fisheries to achieve sustainability. Ambio 51(3), 652-665.
| Crossref | Google Scholar | PubMed |
Anderson MJ (2001) A new method for non-parametric multivariate analysis of variance. Austral Ecology 26, 32-46.
| Crossref | Google Scholar |
Anderson MJ, Ellingsen KE, McArdle BH (2006) Multivariate dispersion as a measure of beta diversity. Ecology Letters 9, 683-693.
| Crossref | Google Scholar | PubMed |
Andrew NL (1991) Changes in subtidal habitat following mass mortality of sea urchins in Botany Bay, New South Wales. Australian Journal of Ecology 16, 353-362.
| Crossref | Google Scholar |
Andrew NL (1993) Spatial heterogeneity, sea urchin grazing and habitat structure on reefs in temperate Australia. Ecology 74, 292-302.
| Crossref | Google Scholar |
Andrew NL (2022) Sea urchins have invaded Tasmania and Victoria, but we can’t work out what to do with them. December 2022. In The Conversation, 6 December 2022. Available at https://theconversation.com/sea-urchins-have-invaded-tasmania-and-victoria-but-we-cant-work-out-what-to-do-with-them-194534
Andrew NL, Choat JH (1982) The influence of predation and conspecific adults on the abundance of juvenile Evechinus chloroticus (Echinoidea:Echinometridae). Oecologia 54, 80-87.
| Crossref | Google Scholar | PubMed |
Andrew NL, O’Neill AL (2000) Large-scale patterns in habitat structure on subtidal rocky reefs in New South Wales. Marine and Freshwater Research 51, 255-263.
| Crossref | Google Scholar |
Andrew NL, Underwood AJ (1989) Patterns of abundance of the sea urchin Centrostephanus rodgersii (Agassiz) on the central coast of New South Wales, Australia. Journal of Experimental Marine Biology and Ecology 131, 61-80.
| Crossref | Google Scholar |
Andrew NL, Agatsuma Y, Ballesteros E, Bazhin AG, Creaser EP, Barnes DKA, Botsford LW, Bradbury A, Campbell A, Dixon JD, Einarsson S, Gerring PK, Hebert K, Hunter M, Hur SB, Johnson CR, Juinio-Menez MA, Kalvass P, Miller RJ, Moreno CA, Palleiro JS, Rivas D, Robinson SML, Schroeter SC, Steneck RS, Vadas RL, Woodby DA, Xiaoqi Z (2002) Status and management of world sea urchin fisheries. Oceanography and Marine Biology: An Annual Review 40, 343-425.
| Google Scholar |
Blount C, Worthington DG (2002) Identifying individuals of the sea urchin Centrostephanus rodgersii with high-quality roe in New South Wales, Australia. Fisheries Research 58, 341-348.
| Crossref | Google Scholar |
Blount C, Chick R, Worthington DG (2017) Enhancement of an underexploited fishery – improving the yield and colour of roe in the sea urchin Centrostephanus rodgersii by reducing density or transplanting individuals. Fisheries Research 186, 586-597.
| Crossref | Google Scholar |
Blount C, Worthington DG, Byrne M, Chick RC, Andrew NL (2024) Aging of the sea urchin Centrostephanus rodgersii using demi-pyramid microstructure. Fisheries Research 270, 106899.
| Crossref | Google Scholar |
Brown JH (1984) On the relationship between abundance and distribution of species. The American Naturalist 124(2), 255-279.
| Crossref | Google Scholar |
Byrne M, Emlet RB, Cerra A (2001) Ciliated band structure in planktotrophic and lecithotrophic larvae of Heliocidaris species (Echinodermata: Echinoidea): a demonstration of conservation and change. Acta Zoologica 82, 189-199.
| Crossref | Google Scholar |
Byrne M, Foo S, Soars NA, Wolfe KDL, Nguyen HD, Hardy N, Dworjanyn SA (2013) Ocean warming will mitigate the effects of acidification on calcifying sea urchin larvae (Heliocidaris tuberculata) from the Australian global warming hot spot. Journal of Experimental Marine Biology and Ecology 448, 250-257.
| Crossref | Google Scholar |
Byrne M, Gall ML, Campbell H, Lamare MD, Holmes SP (2022) Staying in place and moving in space: contrasting larval thermal sensitivity explains distributional changes of sympatric sea urchin species to habitat warming. Global Change Biology 28(9), 3040-3053.
| Crossref | Google Scholar | PubMed |
Chick RC (2020) Stock assessment report 2020 – sea urchin and turban shell fishery: red sea urchin (Heliocidaris tuberculata). (NSW Department of Primary Industries, Fisheries NSW: Port Stephens, NSW, Australia) Available at https://www.dpi.nsw.gov.au/__data/assets/pdf_file/0007/1347334/NSW-Stock-Assessment-2020-SUTS-Fishery-Red-Urchin.pdf
Chick RC (2023) Fishery statistics summary 2023 – sea urchin and turban shell fishery: red sea urchin (Heliocidaris tuberculata). (NSW Department of Primary Industries, Fisheries NSW: Port Stephens, NSW, Australia) Available at https://www.dpi.nsw.gov.au/__data/assets/pdf_file/0004/1487254/Fishery-Statistics-Summary-2023-24-Red-Sea-Urchin.pdf
Collin R, Rebolledo AP, Smith E, Chan KYK (2021) Thermal tolerance of early development predicts the realised thermal niche in marine ectotherms. Functional Ecology 35, 1679-1692.
| Crossref | Google Scholar |
Comely CA, Ansell AD (1988) Population density and growth of Echinus esculentus L. on the Scottish west coast. Estuarine Coastal and Shelf Science 27, 311-334.
| Crossref | Google Scholar |
Connell SD, Irving AD (2008) Integrating ecology with biogeography using landscape characteristics: a case study of subtidal habitat across continental Australia. Journal of Biogeography 35, 1608-1621.
| Crossref | Google Scholar |
Curley BG, Kingsford MJ, Gillanders BM (2003) Spatial and habitat-related patterns of temperate reef fish assemblages: implications for the design of Marine Protected Areas. Marine and Freshwater Research 53, 1197-1210.
| Crossref | Google Scholar |
Davis TR, Cadiou G, Champion C, Coleman MA (2020) Environmental drivers and indicators of change in habitat and fish assemblages within a climate change hotspot. Regional Studies in Marine Science 36, 101295.
| Crossref | Google Scholar |
Davis TR, Champion C, Coleman MA (2021) Climate refugia for kelp within an ocean warming hotspot revealed by stacked species distribution modelling. Marine Environmental Research 166, 105267.
| Crossref | Google Scholar | PubMed |
Davis TR, Knott NA, Champion C, Przeslawski R (2023) Impacts of climate change on densities of the urchin Centrostephanus rodgersii vary among marine regions in eastern Australia. Diversity 15, 419.
| Crossref | Google Scholar |
Defeo OM, Castrejon M, Perez-Castaneda R, Castilla JC, Gutiérrez NL, Essington TE, Folke C (2016) Co-management in Latin American small-scale shellfisheries: assessment from long-term case studies. Fish and Fisheries 17, 176-192.
| Crossref | Google Scholar |
Dorman SR, Harvey ES, Newman SJ (2012) Bait effects in sampling coral reef fish assemblages with Stereo-BRUVs. PLoS ONE 7, e41538.
| Crossref | Google Scholar | PubMed |
Ebert TA (1982) Longevity, life-history, and relative body wall size in sea urchins. Ecological Monographs 52, 353-394.
| Crossref | Google Scholar |
Edgar GJ, Cooper C, Baker SC, Barker W, Barrett NS, Becerro MA, Bates AE, Brock D, Ceccarelli DM, Clausius E, Davey M, Davis TR, Day PB, Green A, Griffiths SR, Hicks J, Hinojosa IA, Jones BK, Kininmonth S, Larkin MF, Lazzari N, Lefcheck JS, Ling SD, Mooney P, Oh E, Pérez-Matus A, Pocklington JB, Riera R, Sanabria-Fernandez JA, Seroussi Y, Shaw I, Shields D, Shields J, Smith M, Soler GA, Stuart-Smith J, Turnbull J, Stuart-Smith RD (2020) Reef Life Survey: establishing the ecological basis for conservation of shallow marine life. Biological Conservation 252, 108855.
| Crossref | Google Scholar |
Fagerli CW, Stadniczeñko SG, Pedersen MF, Christie H, Fredriksen S, Norderhaug KM (2015) Population dynamics of Strongylocentrotus droebachiensis in kelp forests and barren grounds in Norway. Marine Biology 162, 1215-1226.
| Crossref | Google Scholar |
Filbee-Dexter K, Scheibling RE (2014) Sea urchin barrens as alternative stable states of collapsed kelp ecosystems. Marine Ecology Progress Series 495, 1-25.
| Crossref | Google Scholar |
Gervais CR, Champion C, Pecl GT (2021) Species on the move around the Australian coastline: a continental-scale review of climate-driven species redistribution in marine systems. Global Change Biology 27(411), 202127.
| Crossref | Google Scholar |
Glasby TM, Gibson PT (2020) Decadal dynamics of subtidal barrens habitat. Marine Environmental Research 154, 104869.
| Crossref | Google Scholar | PubMed |
Himmelmann JH (1978) Reproductive cycle of the green sea urchin, Strongylocentrotus droebachiensis. Canadian Journal of Zoology 56, 1828-1836.
| Crossref | Google Scholar |
Huggett MJ, King CK, Williamson JE, Steinberg PD (2005) Larval development and metamorphosis of the Australian diadematid sea urchin Centrostephanus rodgersii. Invertebrate Reproduction and Development 47, 197-204.
| Crossref | Google Scholar |
Jarque CM, Bera AK (1987) A test for normality of observations and regression residuals. International Statistical Review 55, 163-172.
| Crossref | Google Scholar |
Johnson CR, Ling SD, Ross J, Shepherd S, Miller K (2005) Establishment of the long-spined sea urchin (Centrostephanus rodgersii) in Tasmania: first assessment of potential threats to fisheries. Fisheries Research and Development Corporation Project Number 2001/044. University of Tasmania, Hobart, Tas., Australia.
Jones GP, Andrew NL (1990) Herbivory and patch dynamics on rocky reefs in temperate Australasia: the roles of fish and sea urchins. Australian Journal of Ecology 15, 505-520.
| Crossref | Google Scholar |
Jones GP, Andrew NL (1993) Temperate reefs and the scope of seascape ecology. In ‘Proceedings of the second international temperate reef symposium’, 7–10 January 1992, Auckland, New Zealand. (Eds CN Battershill, DR Schiel, GP Jones, RG Creese, AB MacDiarmid) p. 252. (NIWA Marine: Wellington, New Zealand)
Kingsford MJ, Byrne M (2023) New South Wales rocky reefs are under threat. Marine and Freshwater Research 74(2), 95-98.
| Crossref | Google Scholar |
Konar B (2000) Seasonal inhibitory effects of marine plants on sea urchins: structuring communities the algal way. Oecologia 125, 208-217.
| Crossref | Google Scholar | PubMed |
Lau DCC, Dumont CP, Lui GCS, Qiu JW (2011) Effectiveness of a small marine reserve in southern China in protecting the harvested sea urchin Anthocidaris crassispina: a mark-and-recapture study. Biological Conservation 144, 2674-2683.
| Crossref | Google Scholar |
Levitan DR (1991) Skeletal changes in the test and jaws of the sea urchin Diadema antillarum in response to food limitation. Marine Biology 111, 431-435.
| Crossref | Google Scholar |
Ling SD (2008) Range expansion of a habitat-modifying species leads to loss of taxonomic diversity: a new and impoverished reef state. Oecologia 156, 883-894.
| Crossref | Google Scholar | PubMed |
Ling SD, Johnson CR (2009) Population dynamics of an ecologically important range-extender: kelp beds versus sea urchin barrens. Marine Ecology Progress Series 374, 113-125.
| Crossref | Google Scholar |
Ling SD, Keane JP (2024) Climate-driven invasion and incipient warnings of kelp ecosystem collapse. Nature Communications 15, 400.
| Crossref | Google Scholar | PubMed |
Ling SD, Johnson CR, Frusher SD, Ridgway KR (2009) Overfishing reduces resilience of kelp beds to climate-driven catastrophic phase shift. Proceedings of the National Academy of Sciences 106(52), 22341-22345.
| Crossref | Google Scholar | PubMed |
Ling SD, Scheibling RE, Rassweiler A, Johnson CR, Shears N, Connell SD, Salomon AK, Norderhaug KM, Pérez-Matus A, Hernández JC, Clemente S, Blamey LK, Hereu B, Ballesteros E, Sala E, Garrabou J, Cebrian E, Zabala M, Fujita D, Johnson LE (2015) Global regime shift dynamics of catastrophic sea urchin overgrazing. Philosophical Transactions of the Royal Society – B. Biological Sciences 370, 20130269.
| Crossref | Google Scholar |
McLaren E, Sommer B, Pandolfi JM, Beger M, Byrne M (2024) Taxa-dependent temporal trends in the abundance and size of sea urchins in subtropical eastern Australia. Ecology and Evolution 14, e11412.
| Crossref | Google Scholar | PubMed |
Marzinelli EM, Williams SB, Babcock RC, Barrett NS, Johnson CR, Jordan A, Kendrick GA, Pizarro OR, Smale DA, Steinberg PD (2015) Large-scale geographic variation in distribution and abundance of Australian deep-water kelp forests. PLoS ONE 10(2), e0118390.
| Crossref | Google Scholar | PubMed |
Molinet C, Balboa CA, Moreno CA, Diaz M, Gebauer P, Niklitschek EJ, Barahona N (2013) Variability in the growth patterns of Loxechinus albus along a bathymetric gradient associated with a fishing ground. Bulletin of Marine Science 89, 699-716.
| Crossref | Google Scholar |
Mortlock TR, Goodwin ID, McAneney JK, Roche K (2017) The June 2016 Australian east coast low: importance of wave direction for coastal erosion assessment. Water 9, 121.
| Crossref | Google Scholar |
Mos B, Byrne M, Dworjanyn SA (2020) Effects of low and high pH on sea urchin settlement, implications for the use of alkali to counter the impacts of acidification. Aquaculture 528, 735618.
| Crossref | Google Scholar |
Norderhaug KM, Christie HC (2009) Sea urchin grazing and kelp re-vegetation in the NE Atlantic. Marine Biology Research 5(6), 515-528.
| Crossref | Google Scholar |
NSW Department of Planning and Environment (2012) Nearshore subtidal marine reef systems and soft sediment mapping, New South Wales. (NSW DPE) Available at https://datasets.seed.nsw.gov.au/dataset/nearshore-subtidal-marine-reef-systems-and-soft-sediment-mapping-new-south-walesffd80 [Dataset, verified 1 May 2023]
NSW Total Allowable Fishing Committee (2021) Sea urchin and turban shell restricted fishery: red urchin. Determination for the 2022 fishing period. 12 November 2021. Report prepared by the New South Wales Total Allowable Fishing Committee. (NSW Department of Primary Industries and Regional Development: Sydney, NSW, Australia) Available at https://www.dpi.nsw.gov.au/__data/assets/pdf_file/0003/1441551/INW21-30904-TAF-Committee-Red-Urchin-Determination-2022-FINAL.pdf
Orensanz JM, Parma AM, Jerez G, Barahona N, Montecinos M, Elias I (2005) What are the key elements for the sustainability of ‘S-fisheries’? Insights from South America. Bulletin of Marine Science 76, 527-556.
| Google Scholar |
Ouréns R, Flores L, Fernández L, Freire J (2013) Habitat and density-dependent growth of the sea urchin Paracentrotus lividus in Galicia (NW Spain). Journal of Sea Research 76, 50-60.
| Crossref | Google Scholar |
Perkins NR, Hill NA, Foster SD, Barret NS (2015) Altered niche of an ecologically significant urchin species, Centrostephanus rodgersii, in its extended range revealed using an autonomous underwater vehicle. Estuarine, Coastal and Shelf Science 155, 56-65.
| Crossref | Google Scholar |
Pert CG, Swearer SE, Dworjanyn S, Kriegisch N, Turchini GM, Francis DS, Dempster T (2018) Barrens of gold: gonad conditioning of an overabundant sea urchin. Aquaculture Environment Interactions 10, 345-361.
| Crossref | Google Scholar |
Ridgway KR, Ling SD (2023) Three decades of variability and warming of nearshore waters around Tasmania. Progress in Oceanography 215, 103046.
| Crossref | Google Scholar |
Sagarin RD, Gaines SD (2002) The ‘abundant centre’ distribution: to what extent is it a biogeographical rule? Ecology Letters 5(1), 137-147.
| Crossref | Google Scholar |
Sivertsen K (1997) Geographic and environmental factors affecting the distribution of kelp beds and barren grounds and changes in biota associated with kelp reduction at sites along the Norwegian coast. Canadian Journal of Fisheries and Aquatic Sciences 54, 2872-2887.
| Crossref | Google Scholar |
Soars NA, Byrne M (2015) Contrasting arm elevation angles of multi- and two-armed sea urchin echinoplutei, support Grünbaum and Strathmann’s hydromechanical model. Marine Biology 162, 607-616.
| Crossref | Google Scholar |
Soars NA, Prowse TAA, Byrne M (2009) Overview of phenotypic plasticity in echinoid larvae, ‘Echinopluteus transversus’ type vs typical echinoplutei. Marine Ecology Progress Series 383, 113-125.
| Crossref | Google Scholar |
Tegner MJ, Dayton PK (1981) Population structure, recruitment and mortality of two sea urchins (Strongylocentrotus franciscanus and S. purpuratus) in a kelp forest. Marine Ecology Progress Series 5, 255-268.
| Crossref | Google Scholar |
Underwood AJ, Kingsford MJ, Andrew NL (1991) Patterns in shallow subtidal marine assemblages along the coast of New South Wales. Australian Journal of Ecology 16, 231-249.
| Crossref | Google Scholar |
Vadas RL, Smith BD, Beal B, Dowling T (2002) Sympatric growth morphs and size bimodality in the green sea urchin (Strongylocentrotus droebachiensis). Ecological Monographs 72, 113-132.
| Crossref | Google Scholar |
Vye SR, Dickens S, Adams L, Bohn K, Chenery J, Dobson N, Dunn RE, Earp HS, Evans M, Foster C, Grist H, Holt B, Hull S, Jenkins SR, Lamont P, Long S, Mieszkowska N, Millard J, Morrall Z, Pack K, Parry-Wilson H, Pocklington J, Pottas J, Richardson L, Scott A, Sugden H, Watson G, West V, Winton D, Delany J, Burrows MT, Blakeslee A (2020) Patterns of abundance across geographical ranges as a predictor for responses to climate change: evidence from UK rocky shores. Diversity and Distributions 26, 1357-1365.
| Crossref | Google Scholar |
Whittaker RH (1956) Vegetation of the Great Smoky Mountains. Ecological Monographs 26, 1-80.
| Crossref | Google Scholar |
Worthington DG, Blount C (2003) Research to develop and manage the sea urchin fisheries of NSW and eastern Victoria. NSW Fisheries Final Report Series Number 56, Fisheries Research and Development Corporation Project Number 1999/128. (NSW Fisheries: Sydney, NSW, Australia) Available at https://www.frdc.com.au/sites/default/files/products/1999-128-DLD.pdf
Yatsuya K, Nakahara H (2004) Density, growth and reproduction of the sea urchin Anthocidaris crassispina (A. Agassiz) in two different adjacent habitats, the Sargassum area and Corallina area. Fisheries Science 70(2), 233-240.
| Crossref | Google Scholar |