Tidal migrations of juvenile Sillago spp. in a subtropical intertidal nursery seascape
Max L. Giaroli A , Craig A. Chargulaf


A School of Biological Sciences, University of Queensland, Saint Lucia, Qld 4067, Australia.
B Arup, Level 4, 108 Wickham Street, Fortitude Valley, Qld 4006, Australia.
C School of Science, Technology and Engineering, University of the Sunshine Coast, Petrie, Qld 4502, Australia.
Marine and Freshwater Research 74(14) 1193-1210 https://doi.org/10.1071/MF23033
Submitted: 15 February 2023 Accepted: 19 July 2023 Published: 29 August 2023
Abstract
Subtropical intertidal pools on depositional shores are important nursery habitats for smaller juveniles (10- to ~25-mm total length, TL) of commercially important smelt whiting (Sillago spp.), whereas larger juveniles >25 mm TL occupy shallow subtidal habitats at low tide.
We investigated the connectivity between lower and upper shore habitats in Moreton Bay.
We used funnel camera traps to assess tidal movements of juvenile whiting and compared harpacticoid copepod genera in small juvenile whiting guts caught post-foraging with those sampled from nearby sediments to infer patterns of foraging.
Smaller juveniles transited sandy upper-shore habitat at depths from 1.5 to <10 cm and avoided vegetated habitats, whereas larger juveniles moved into mangroves at depths of 15–30 cm on the rising tide. Coullana spp. harpacticoids were found in greater proportions in whiting guts of small juveniles than in the sampled habitats, but were abundant in intertidal pool and mangrove sediments.
Intertidal sandy habitats are seemingly an important high-tide habitat for smaller juvenile whiting, where they preferentially forage on Coullana spp.
Given the broad distribution of smelt whiting in the Indo-Pacific, the protection of such habitats must be addressed by fishery and habitat management agencies for species with similar early nursery requirements.
Keywords: diet, harpacticoid, intertidal, mangrove, nursery, sandflat, seagrass, video, whiting.
Introduction
Intertidal flats are an important interface between sublittoral marine and terrestrial systems (Nagelkerken et al. 2008). Mud and sandflats, mangrove forests and intertidal seagrass meadows are linked by intertidal pools and streams to form a complex mosaic of habitat patches that support diverse and abundant fish and invertebrate communities (Bucher and Saenger 1994; Hindell and Jenkins 2004; Adkins et al. 2016). However, living in the intertidal zone is challenging because regular inundation by the sea drives rapid changes in biophysical parameters such as salinity, oxygen availability, temperature, and desiccation risk, that induce considerable physiological demands on its inhabitants (Broekhuysen 1940; Morris and Taylor 1983; Somero 2002). However, there are important ecological advantages to being able to exploit intertidal habitats as the tides provide access to additional food resources, through nutrient and meiofauna-rich sediments (Weisberg et al. 1981; Madon et al. 2001). Occupying the shallow water at the leading edge of the incoming tide, known as the tidal excursion front (Birt and Tibbetts 2007), may also allow smaller species to avoid larger predators that by virtue of their size must occupy deeper water (Rypel et al. 2007; Boswell et al. 2019). Moreover, juvenile nekton may experience accelerated development because of the elevated temperature of the shallow intertidal pools (Krück et al. 2009) and the tidal excursion front (Birt and Tibbetts 2007). Intertidal habitats are therefore important nurseries for a diversity of fish and crustaceans (Kneib 1993; Laegdsgaard and Johnson 1995; Seitz et al. 2014) and have a fundamental value to some important fisheries (Martinho et al. 2012; Kimirei et al. 2013; Sundblad et al. 2014).
The nursery seascape approach, in which nursery areas are considered mosaics of interconnected intertidal habitats, is increasingly being used to describe habitat usage by juvenile fishes (Nagelkerken et al. 2015; Litvin et al. 2018). Such an approach incorporates factors such as tidal and ontogenetic migrations, hydrodynamics, and interspecific interactions including behaviours such as foraging and sheltering (Nagelkerken et al. 2015; Litvin et al. 2018). For example, juvenile rock fish (Sebastes spp.), a commercially important species in the north-eastern Pacific, was thought to use only seagrass meadows as a nursery habitat. However, isotope analysis of gut contents showed that it receives additional food resources from adjacent kelp forests that improve the condition of juvenile fish (Olson et al. 2019). This information was used to advocate for incorporating kelp–seagrass connectivity in conservation planning (Olson et al. 2019). The nursery seascape concept is highly applicable to intertidal areas where there is strong zonation and partitioning of habitats (Mattone et al. 2022). However, identifying the nursery seascapes and their use can be quite complex, owing to the simultaneous impact of several variables, including human disturbances, prevailing conditions of weather and tide, and movement dynamics of fishes; thus, an integrated approach is required to address knowledge gaps (Crook et al. 2015).
Moreton Bay is a subtropical embayment in Queensland, Australia, flanked to the east by vegetated sand islands and to the west by a heavily urbanised mainland (Gibbes et al. 2013). The coastline of Moreton Bay, and its islands, comprise extensive intertidal flats that sustain mangroves, intertidal seagrass, saltmarsh and unvegetated mud and sandflats (Lovelock et al. 2019). These habitats combine to form a diverse seascape of habitat patches, connected by intertidal streams, depressions and sometimes seagrass corridors used by fishes to move among habitat patches (Davis et al. 2017; Espadero et al. 2020). Moreton Bay’s depositional shores are also characterised by soft-sediment pools formed by foraging stingrays that provide refugia for juvenile fish and prawns at low tide (Meager et al. 2005; Krück et al. 2009; Chargulaf et al. 2011), including many species captured in local fisheries (Laegdsgaard and Johnson 1995; Thomas and Connolly 2001).
Smelt-whiting (family Sillaginidae) is a demersal fish that is commonly harvested across much of the Indo-west Pacific (McKay 1992). In Queensland, over 1.2 million individuals were caught by recreational fishers in 2019, making whiting the second-most harvested recreational species in the state (Misson et al. 2020). This strong recreational catch, along with a state-wide commercial harvest of ~1200 tonnes (Mg) results in considerable pressure on the stock (Leigh et al. 2019; Wortmann 2020). The most recent stock assessment in south-eastrn Queensland estimated sand whiting (Sillago ciliata) biomass to be at <29% of unfished biomass, well below the 60% target given in Queensland’s Sustainable Fishing Strategy 2017–2017 (Leigh et al. 2019). Given their current low stock level and their recreational and commercial importance, improving our understanding of their nursery habitat use will allow interventions that could protect existing stocks and accelerate their recovery.
Moreton Bay hosts three common sillaginids, namely, sand whiting (Sillago ciliata), winter whiting (S. maculata), and golden-line whiting (S. analis), which are all harvested by commercial and recreational fishers (Gray and Kennelly 2003). Whiting species are notoriously difficult to distinguish visually as juveniles (Weng 1983) and so most studies involving juvenile whiting have been conducted at the level of genus (e.g. Krück et al. 2009; Chargulaf et al. 2011). Current understanding of their ecology indicates that larval whiting first settle onto sandflats, with high numbers of small juveniles being observed in intertidal soft-sediment pools in spring through to autumn (Krück et al. 2009; Chargulaf et al. 2011). The pools are thought to act as a low-tide refuge from predation for small juvenile whiting (<25-mm total length, TL), where they feed on benthic harpacticoid copepods and nematodes (Coull et al. 1995; Krück et al. 2009). However, it is not known whether they remain in these depressions as the tide inundates the shore, or whether they migrate up the shore to other refugia. If they are tidal migrants, then understanding their migration pathways as they ingress and egress upper shores is important to characterise to manage and conserve those corridors. Larger juvenile whiting (>25 mm TL) undertake significant ontogenetic habitat shift and transition to a diet comprised mostly of decapods and polychaetes (Krück et al. 2009). These larger juvenile whiting migrate up the shore with the tide to access mangroves, and are particularly common along the leading edge of the forest among mangrove pneumatophores (Laegdsgaard and Johnson 1995; Thomas and Connolly 2001). Owing to their larger size, it is possible that they move into these habitats in deeper water than do smaller individuals, which could have implications on the probability of interactions with potential predators.
Despite patterns in whiting abundance within the sandflat–seagrass–mangrove fringe ecotone being studied previously, the specific routes over which these movements take place, and reasons for those movements, remain poorly understood. This is typically related to challenges in monitoring movement of these small and often highly camouflaged fish throughout tidal cycles. Recent developments in underwater-video techniques have allowed for better description of the movement of intertidal fishes (Ellis and Bell 2008; Kimball and Able 2012; Davis et al. 2017) and offer an approach to understand the tidal migrations of juvenile whiting in Moreton Bay. In addition to these visual approaches, dietary information can elucidate patterns of fish movement across seascapes (Davis et al. 2015; Thomas et al. 2020). Harpacticoid copepods are a major dietary component of juvenile whiting. They were present in 85% of juvenile whiting guts caught from intertidal pools, and account for 45% of prey volume in those guts (Krück et al. 2009). Assemblages of harpacticoids display habitat specificity (Findlay 1981; Coull 1999; Azovskii and Chertoprud 2002; Stringer et al. 2012; Ghosh and Mandal 2019), meaning that comparisons of harpacticoid assemblages consumed by whiting with those from sediment samples offers a potential method for understanding the importance of different microhabitats to foraging juvenile whiting.
In this study, we combined video and diet data to test three complimentary hypotheses about the movement of whiting. First, we hypothesised that small and large juvenile whiting individuals would access different upper-shore habitats and differ in the pathways used to access these habitats. Second, we hypothesised that these differences would translate to differences in the water depth in which these fish egress and ingress habitats because of accessibility. Finally, we hypothesised that the harpacticoid copepods in the guts of smaller juvenile whiting individuals caught after high tide will resemble the harpacticoid communities in their high-tide foraging grounds.
Materials and methods
Study sites
This study was conducted on the intertidal sandflats of eastern Moreton Bay (Quandamooka), around the township of Dunwich (Goompi) on North Stradbroke Island (Minjerribah). These low-energy intertidal sandflats experience semi-diurnal tides that have a mean spring tidal range of ~1.5 m and mean neap tidal range of ~0.8 m. They are characterised by extensive intertidal pools and intertidal habitat patches including Avicennia and Rhizophora mangroves and intertidal Zostera and Halophila seagrass beds. Some of these shores have been anthropogenically altered and now include other habitats such as sandy beaches and sand breaks, which are sections of shore ~10 m wide with no mangroves. Both sandy beaches and sand breaks do not normally persist on the western side of North Stradbroke Island. These habitats could be used as a proxy for low-energy depositional shores that lack mangroves elsewhere. The following three shores were investigated in this study: Myora (27°28′15″S, 153°25′19″E), One Mile Beach (27°29′23″S, 153°24′22″E) and Adam’s Beach (27°30′28″S, 153°24′36″E) (Fig. 1). Each of these shores displayed a variety of habitat patches and allowed comparisons to be made among mangrove, mangrove–seagrass, and sand break habitats. Extensive Avicennia marina and Rhizophora stylosa mangrove forests extend ~30–70 m at Myora and Adam’s Beach, and a narrow band of A. marina is present at One Mile beach. Intertidal Zostera muelleri and Halophila ovalis seagrass beds were also present on the intertidal flat at Adam’s Beach and were patchy at One Mile Beach, occurring only at the mangrove edges. Sand breaks were present at both Adam’s Beach and One Mile Beach, but not at Myora.
Funnel traps
Digital video recordings of funnel-trap apertures were used to observe movement of juvenile whiting individuals between sandflats and high-tide habitat patches, and the use of other habitat features such as channels. Here, tidally migrating fish were funnelled past two GoPro cameras that recorded videos that were post-processed to quantify fish movement. Funnels consisted of four 1.5 m long, 0.2 m high metal flyscreen ‘nets’ with 1-mm mesh, and wings were set in a X-shape orientation, with a 0.1-m opening at the apex (Fig. 2). One GoPro (either a Hero 5: field of view, FOV, medium; resolution, 1080 pixels; frames per second, FPS, 60, with a 10× magnifying lens attached; or a Hero 8: FOV, wide; resolution, 1080 pixels; FPS, 120) was set up on the benthos, facing the apex, against the tide to retrieve high-resolution images for fish identification. Two different GoPro models were used because of camera availability and the specifications of the two GoPro models varied as a result of the settings available on both cameras. Both models were set up so that the camera remained between 10 and 15 cm from the apex, with the point of focus being located in the middle of the apex, and both funnel walls being in frame. The other GoPro (Hero 8: FOV, super view; resolution 2700 pixels; FPS, 90) was set up on the other side of the apex to observe the entire water column and ensure that whiting were not swimming over the funnel trap when the funnel walls were fully submerged. The same camera configuration was used at all sites. The funnel walls and cameras were held in place by 0.3-m metal tent pegs. Having two GoPros also made this setup more robust to recording errors and obstructions such as leaf-litter blocking cameras.
Diagram of the X-shaped funnel trap, showing the dimensions of the flyscreen netting, general position of both GoPro cameras and the flyscreen netting. GoPros and flyscreen netting were held in place by using 0.3-m tent pegs. This funnel trap was used as a trial, the gap at the apex was reduced to 0.1 m following this trial.
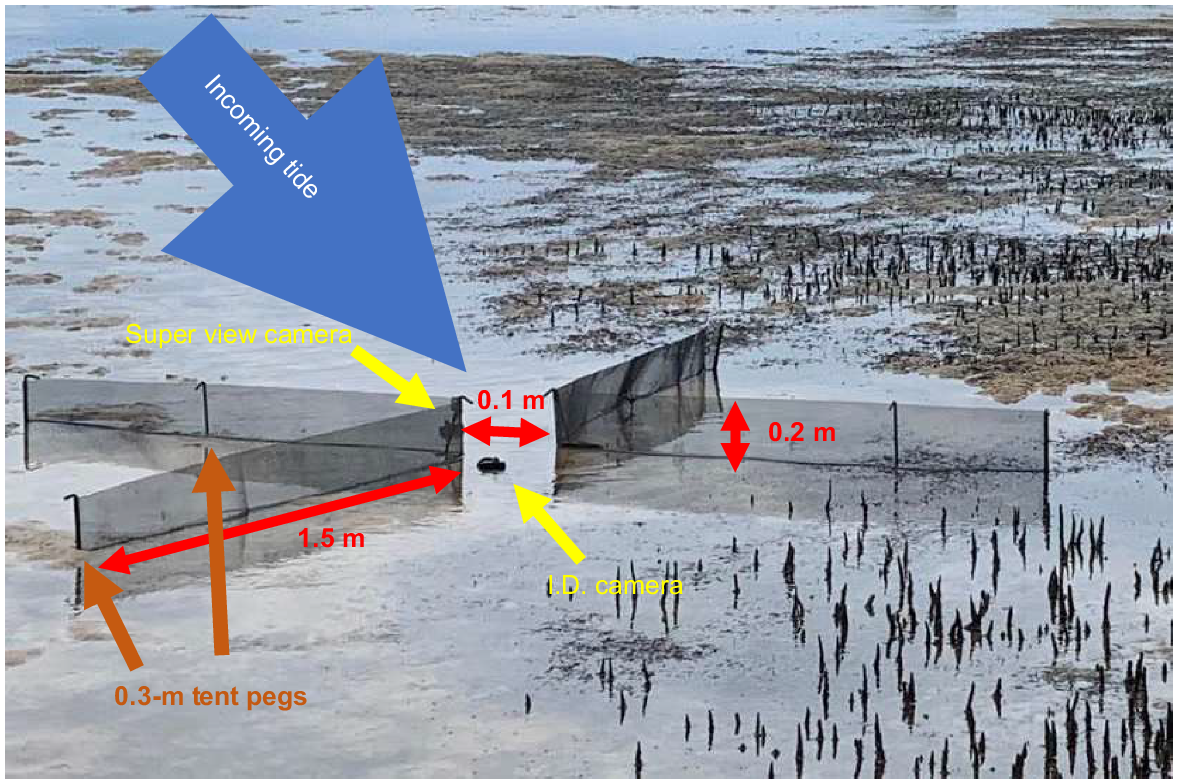
Video data collection
Sampling was conducted between October and November 2021 and January and February 2022 on spring tides when the tidal range was greater than 1.3 m and outside of crepuscular periods (i.e. 1 h either side of sunrise or sunset) to standardise for potential temporal and tidal effects. The first and last hours of water passing through the funnel-video trap, corresponding to 0–300-mm and 300–0-mm water depth, would have the highest probable movement of juvenile whiting between low-tide and high-tide habitat patches. For this reason, and because of the battery-life constraints of GoPro cameras, recordings were made only for the first and last hours of inundation. Cameras were removed after the first hour of inundation to charge batteries, with the funnel traps remaining in place. Cameras with fully charged batteries were returned to the same funnel trap for the last hour of inundation.
Funnel traps were set up at the following five habitat-patch interfaces: (1) channels and (2) no channels, the latter hereafter being referred to as flats, between intertidal seagrass and mangrove, (3) channels and (4) flats between sandflat and mangrove, and (5) channels between sandflat and sand break (Fig. 3). Eight replicate funnel traps were set up at each habitat interface on both incoming and outgoing tides. All footage from some replicates was excluded from analysis because of poor visibility, which was defined as either the apex of the funnel trap not being visible for more than 2 min owing to high turbidity, or floating particulate debris obscuring more than 10% of the field of view. This reduced the level of replication available for analysis to n = 6 for incoming mangrove flat and incoming seagrass channel and n = 7 for incoming seagrass-channel interfaces. The leading edge of pneumatophores was considered the border between mangroves and other habitats, but the border between sand break and sandflat was difficult to establish. Therefore, funnel trap openings were set up parallel to the pneumatophore edge of adjacent mangroves. For each funnel-trap observation, the abundance of smaller (<25 mm TL) juvenile whiting and larger (>25 mm TL) juvenile whiting individuals in 5-cm water depth increments from 0 to 30 cm were recorded. The size of juvenile whiting was estimated using 10-mm markers on the funnel walls. Water depth was determined by counting 10-mm markers along the funnel walls at the apex and was measured at average wave height. If whiting were observed moving against the tide, then the net movement of juvenile whiting was calculated. S. maculata, S. ciliata, and S. analis were not distinguished from each other because of the difficulty of visual identification of these species at small sizes (Weng 1983). Subadult and adult whiting (>10 cm) were excluded from analyses because too few were observed to make any sort of inference.
The five different habitat interfaces: intertidal seagrass–mangrove flats, seagrass–mangrove channels, intertidal sandflat–mangrove flats, sandflat–mangrove channels, and intertidal sandflat–sand break channels at (a) Adam’s Beach and (b) Myora, where funnel traps could be set up. Multiple examples of these habitat interfaces were identified and sampled at each location.
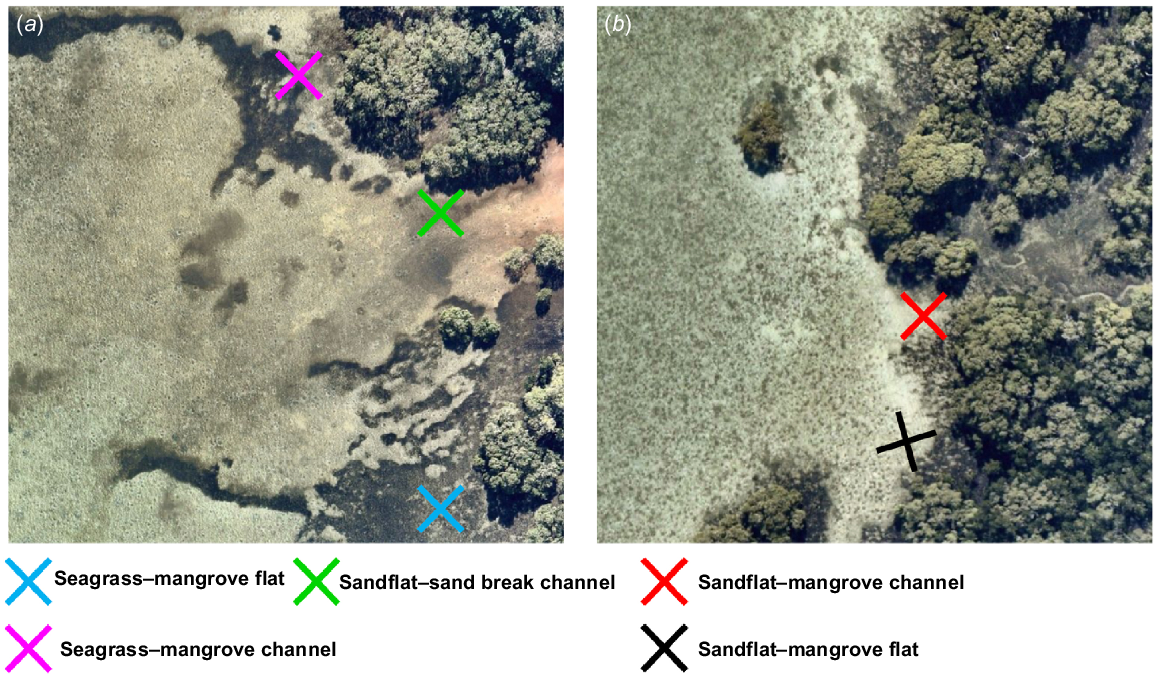
Video data statistical analysis
A generalised linear mixed-effects model (GLMM), with a Tweedie distribution, was conducted using the ‘lme4’ package (ver. 1.1-34, see https://CRAN.R-project.org/package=lme4; Bates et al. 2015) in R (ver. 4.1.2, R Foundation For Statistical Computing, Vienna, Austria, see https://www.R-project.org/). This model assessed statistical differences in the dependent variable, which was the rate of juvenile whiting passing the funnel apex per minute across three independent variables, namely, size class, water depth, and habitat interface, and a further two random variables, namely, month and study site. The numbers of smaller (<25 mm) and larger (>25 mm) whiting observed passing the trap per minute were calculated by quantifying the number of individuals observed passing the trap during the time (min) when a change in water depth of 5 cm occurred (i.e. six depth bins from 0 to 30 cm on rising and falling tides). Habitat interfaces included (1) flats and (2) channels separating mangroves and the sandflat, (3) flats and (4) channels separating mangroves and seagrass beds, and (5) channels separating the sandflat and sand breaks. All possible combinations of size classes, habitat interfaces, and water depths were compared against a base condition of smaller juveniles in mangrove channels at 0–<5-cm depth to determine significance.
Sediment sample collections
Sediment samples (and juvenile whiting for gut analysis) were collected from One Mile Beach, as considerably more juvenile whiting individuals were observed by funnel traps at this beach than at the other sites. Four sediment samples were collected from each of three potential high-tide foraging habitats, namely, sand breaks, sediment among pneumatophores at the mangrove–sandflat edge, and within mangroves. Four additional samples were collected from intertidal pools, both before and after high tide in the same week in February 2022. Samples for each habitat were collected within 6-m radius of the first sample and not within 1 m of each other. The habitats sampled were adjacent to each other (Fig. 4) and samples were collected near areas where smaller juvenile whiting individuals were caught in intertidal pools. These samples were collected to a depth of 1 cm using a 4.5-cm diameter syringe (total sample: 16 cm2), stained with Rose Bengal and kept in 50-mL sample tubes where they were fixed with a 70% ethanol:distilled water mix.
Processing and analysis of sediment samples
Each sediment sample went through three iterations of suspension, by using a CSV 90 Auto Vortex Mixer on a power setting of four, and sieving through a 45-μm mesh sieve. On the basis of Armenteros et al. (2008), it was expected that this method should recover over half of the harpacticoids from each sample. All matter retained on the sieve was transferred to a Petri dish and scanned for harpacticoid copepods by using an Olympus SZ-50 dissecting microscope. Once counted, each harpacticoid copepod was transferred into a sample tube containing the same morphotype to avoid recounting individuals. The total number of each morphotype was recorded until formal identification could occur. Morphotypes were subsequently identified down to genus under an Olympus BX-41 compound microscope at 10× magnification. A subsample of up to 50 individuals from each sample were mounted onto microscope slides and observed under the compound microscope to check that no cryptic genera were incorrectly identified under the same morphotype.
Non-metric multidimensional scaling (nMDS) was conducted using the PERMANOVA+ addon of the Plymouth Routines in Multivariate Ecological Research (PRIMER) software (ver. 6, see https://www.primer-e.com/; Anderson et al. 2008), to identify and assess differences among harpacticoid communities from different habitats. nMDS was calculated using square-root-transformed count data on Bray–Curtis dissimilarity. Differences in harpacticoid assemblage structure among habitats were identified using permutational multiple ANOVA (PERMANOVA), with 9999 permutations. The contribution of each genus to the dissimilarities observed was identified using similarity percentage analysis (SIMPER), also in PRIMER.
Whiting gut sample collections
Two samples of 10 smaller (10–25 mm TL) juvenile whiting individuals were caught for gut sample analysis from intertidal pools by using hand-held dip nets, euthanased in a 175 mg L−1 mixture of Aqui-S:seawater for 20 min, measured using a ruler to the nearest millimetre (±1 mm), and stored in Eppendorf tubes containing absolute ethanol. Larger juveniles (i.e. >25 mm TL) were not used because harpacticoids form only a small proportion of their diet (Krück et al. 2009). Ten individuals were caught each side of the high tide as pools became exposed and just before they were inundated by the rising tide. Fish were collected on the same day as the sediment samples at each site. Given that juvenile whiting evacuate their guts within 6 h of feeding (Coull 1999), the guts of whiting caught on the ebbing tide should contain harpacticoids consumed in high-tide foraging areas. The capture and observation of whiting were conducted under UQ Animal Ethics permit 2021/AE000280.
Processing and analysis of whiting samples
Euthanased whiting were dissected, with gut contents gently emptied onto a Petri dish. Gut fullness was qualitatively scored from 0 (empty) to four (full) on the basis of the quantity of gut contents relative to fish size. Harpacticoids from gut content samples were separated and identified to genus under an Olympus SZ-50 dissecting microscope and Olympus BX-41 compound microscope. To compare the harpacticoid copepod communities in the fish guts and in sediment samples, count data for both whiting and sediment samples were standardised into the proportions of each genus in a sample and square-root transformed. Data were again square-root transformed and visualised using nMDS calculated on Bray–Curtis dissimilarity. Differences in harpacticoid assemblage structure between habitats and the guts of whiting caught before and after high tide were quantified using PERMANOVA. The contribution of each genus to the differences between groups was identified using SIMPER. Gut fullness between whiting caught before and after high tide were compared using a Student’s t-test in R.
Results
Funnel-trap video records
In total, 354 whiting individuals were observed passing through funnels. Twenty-one other species, including potential predators of juvenile whiting, such as large Favonigobius spp., Platycephalus spp., and Pseudorhombus spp., were also observed. Most of the individuals observed were <120 mm TL and were likely to be juveniles (Table 1). All these species displayed differences regarding the depth they were observed at, the high-tide habitats they utilised, whether they were solitary or schooling, and the species with which they interacted. However, this report focuses on the trends observed in whiting only.
Species | Size range | Sand channel 16 (funnel traps) | Mangrove flat 14 | Mangrove channel 16 | Seagrass channel 15 | Seagrass flat 14 | |
---|---|---|---|---|---|---|---|
Sillago spp. | <25 mm | ++++ (73%) | + (18%) | + (44%) | + (13%) | + (19%) | |
>25 mm | ++ (47%) | ++ (29%) | +++ (75%) | ++ (27%) | + (25%) | ||
Favonigobius lentiginosus | <40 mm | ++++ (87%) | ++++ (94%) | ++++ (100%) | ++++ (87%) | +++ (94%) | |
>40 mm | ++ (27%) | +++ (41%) | ++ (19%) | +++ (53%) | +++ (50%) | ||
Platycephalus spp. | 50–120 mm | + (27%) | + (12%) | + (6%) | + (13%) | + (19%) | |
Acanthopagrus australis | <50 mm | + (27%) | ++ (35%) | ++ (44%) | ++ (53%) | ++ (50%) | |
Gerres spp. | <50 mm | + (7%) | – | + (6%) | ++ (40%) | ++ (19%) | |
Atherinomorus vaigiensis | <25 mm | +++ (40%) | ++ (30%) | + (13%) | ++ (33%) | ++ (25%) | |
Pseudorhombus jenynsii | 50–120 mm | – | – | + (19%) | + (27%) | – | |
Mugilidae spp. | <60 mm | – | – | – | ++ (13%) | + (6%) | |
>60 mm | + (7%) | – | + (6%) | + (7%) | – | ||
Ambassis marinus | <40 mm | – | + (6%) | ++++ (19%) | – | – | |
Centropogon australis | <25 mm | – | + (6%) | – | + (20%) | + (31%) | |
Terapon jarbua | <40 mm | + (13%) | + (6%) | ++ (19%) | ++ (33%) | + (13%) | |
Tylosurus gavialoides | >150 mm | – | + (12%) | + (6%) | – | – | |
Tetractenos hamiltoni | <60 mm | ++ (33%) | +++ (47%) | +++ (56%) | +++ (60%) | ++ (31%) | |
>60 mm | ++ (40%) | +++ (59%) | +++ (63%) | ++++ (93%) | ++++ (100%) | ||
Torquigener pleurogramma | <60 mm | + (13%) | +++ (35%) | +++ (81%) | ++ (33%) | + (6%) | |
Marylina pleurosticta | <40 mm | – | – | – | + (7%) | – | |
Lutjanus russelli | <40 mm | – | – | – | + (7%) | + (6%) | |
Pelates sexlineatus | <40 mm | – | – | + (6%) | + (7%) | – | |
Sphyraena sp. | <50 mm | – | – | + (13%) | – | – | |
Omobranchus sp. | >50 mm | – | – | – | – | + (6%) | |
Unknown sp. 1 | – | + (6%) | – | – | + (13%) | + (6%) | |
Unknown sp. 2 | – | – | – | + (6%) | – | – | |
Unknown sp. 3 | – | – | – | – | – | + (6%) |
–, no observation; +, 1–15 individuals observed; ++, 15–50 individuals; +++, 50–120 individuals; ++++, 120+ individuals. The percentages in parentheses are the proportion of funnel traps at different habitat interfaces the species was observed.
There was no significant difference in the mean rate per minute of smaller (<25 mm) and larger (>25 mm) juvenile whiting individuals (t = 0, P = 1) passing funnel traps at the interface of habitats. Sand channels, the interface between sand breaks and sand flats, were the only habitat that saw passage of significantly more small juvenile whiting per minute (t = 9.482, P < 0.0001). There was also no significant difference in the rate per minute of juvenile whiting observed at different water depths. Despite the lack of significant differences among these variables individually, several significant interactions were observed (Table 2) that do identify important differences between the two size classes of whiting, habitats occupied during at least part of the high tide, and the depths utilised by these juveniles.
Interactions | t-value | P-value | |
---|---|---|---|
Sand channel | 9.48 | <0.0001 | |
Sand channel × larger juvenile | −8.25 | <0.0001 | |
Sand channel × 5–<10-cm depth | −5.44 | <0.0001 | |
Sand channel × 10–<15-cm depth | −8.45 | <0.0001 | |
Sand channel × 15–<20-cm depth | −8.25 | <0.0001 | |
Sand channel × 20–<25-cm depth | −7.64 | <0.0001 | |
Sand channel × 25–<30-cm depth | −5.68 | <0.0001 | |
Sand channel × ≥30-cm depth | −8.25 | <0.0001 | |
Larger juvenile × 15–<20-cm depth | 3.01 | 0.0026 | |
Larger juvenile × 20–<25-cm depth | 3.60 | 0.0003 | |
Larger juvenile × 25–<30-cm depth | 2.15 | 0.0312 | |
Sand channel × 5–<10-cm depth × larger juvenile | 4.22 | <0.0001 | |
Sand channel × 10–<15-cm depth × larger juvenile | 6.35 | <0.0001 | |
Sand channel × 15–<20-cm depth × larger juvenile | 4.10 | <0.0001 | |
Sand channel × 20–<25-cm depth × larger juvenile | 3.03 | <0.0001 | |
Sand channel × 25–<30-cm depth × larger juvenile | 2.87 | 0.0041 | |
Sand channel × ≥30-cm depth × larger juvenile | 4.92 | <0.0001 | |
Seagrass channel × 20–<25-cm depth × larger juvenile | −2.00 | 0.0461 |
Significance determined from pairwise comparison with the base condition, depth 0–<5 cm, smaller juvenile, mangrove channel.
Differences occurred between the two size classes of juvenile whiting. A greater abundance of smaller whiting traversed sand channels than any other habitat, and they primarily did so at shallower (1.5 to <10 cm) depths, with a noticeable peak (mean ± s.e.) of 2.71 ± 1.12 whiting min−1 on the outgoing tide and 0.94 ± 0.61 whiting min−1 on the incoming tide between 1.5 and <5 cm (Fig. 5). There was a smaller second peak in abundance in the 25 to <30 cm depth bin. By contrast, larger juveniles were more abundant crossing the interface between mangroves and sand flats, particularly in channels, and they passed at greater depths of 15–30+ cm (Fig. 6). Although the GLMM model identified no significant difference in whiting abundance among mangrove−sandflat and mangrove−seagrass interface combinations (Table 3), except for fewer larger individuals transiting mangrove−seagrass channels at 20 < 25 cm (Tweedie−GLMM, t = −2.0, P < 0.0461), many more whiting were observed moving within the mangrove−sandflat habitat-interface combination (Fig. 6). There also appeared to be greater larger-juvenile whiting abundance in channels rather than flats; however, without data on sand beach−sandflat habitat interfaces, this is largely speculative (Fig. 6). Additionally, large variance of 26.07 was observed across the random variables, study site and month sampled. The high variance means that the observed trends in juvenile whiting movement are quite variable spatially across study sites and temporally across the spring and summer months.
Mean rate per minute of smaller (<25 mm TL) juvenile whiting individuals (black bars) and larger (>25 mm TL) juveniles (grey bars), observed by GoPro cameras, passing funnel traps at sand channels. GoPros were set to record for 1 h on the incoming tide from when the inundating water met the seaward limit of the trap and 1 h of ebb tide, so that at the end of the hour the trap was fully exposed, which translates to a change of ~30 cm (300 mm) in water depth. Each habitat refers to the interface between sandflats or intertidal seagrass and the higher littoral environments such as mangroves or sand breaks. The hatched bar represents the high tide where no recordings were made, covering a period of ~5–6 h.
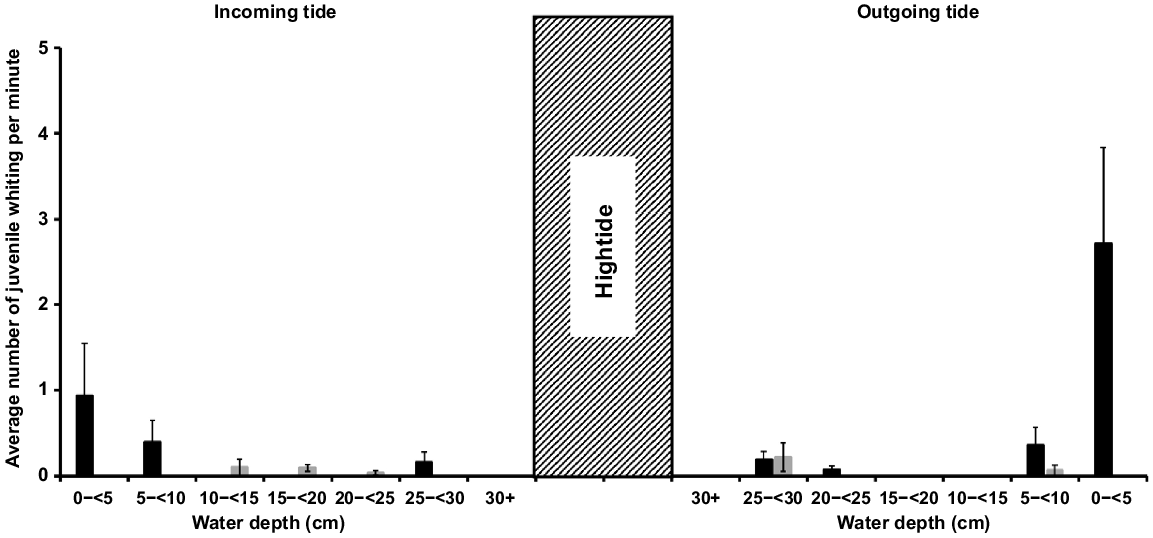
Mean rate per minute of smaller (<25 mm TL) juvenile whiting individuals (black bars) and larger (>25 mm TL) juveniles (grey bars), observed by GoPro cameras, passing funnel traps at (a) seagrass channels, (b) seagrass flats, (c) mangrove flats, and (d) mangrove channels. GoPros were set to record for 1 h on the incoming tide from when the inundating water met the seaward limit of the trap and 1 h of ebb tide, so that at the end of the hour, the trap was fully exposed, which translates to change of ~30 cm (300 mm) in water depth. Each habitat refers to the interface between sandflats or intertidal seagrass and the higher littoral environments such as mangroves or sand breaks. The hatched bar represents the high tide where no recordings were made, covering a period of ~5–6 h.
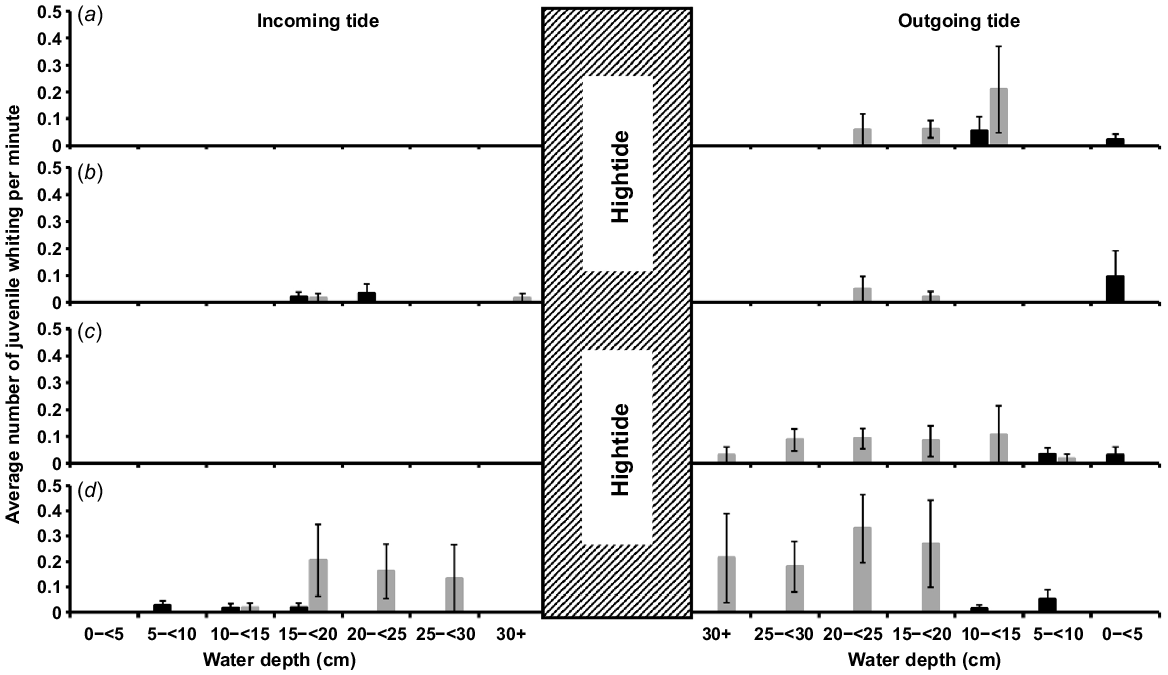
Interactions | t-value | P-value | |
---|---|---|---|
Mangrove flat | 0.388 | 0.7053 | |
Seagrass channel | −0.21 | 0.8386 | |
Seagrass flat | 0.48 | 0.6342 |
Significance determined from pairwise comparison with the base condition, mangrove channel.
Gut-sample and sediment-sample comparison
Harpacticoid copepods were observed in every sediment sample, but their abundance varied among habitats. Mangrove sediments had the highest density of harpacticoid copepods (385 ± 114, mean ± s.e.) per 16-cm2 sample, followed by pneumatophores at (40 ± 29, mean ± s.e.), and densities were lowest in intertidal pools (18 ± 8, mean ± s.e.) and sand-break samples (22 ± 3, mean ± s.e.). In these habitats, Coullana spp., Canuella spp., Brianolla spp., and Ectinosoma spp. were observed (Fig. 7); however, only the first three were common (Fig. 8). Canuella spp. were the most common species in the mangrove samples (355 ± 106, mean ± s.e.) and sand-break samples (20 ± 3, mean ± s.e.). Mangrove samples also had high abundance of Coullana spp. (28 ± 14, mean ± s.e.). Whereas in intertidal pools, Coullana spp. (11 ± 5, mean ± s.e.) and Brianolla spp. (7 ± 4, mean ± s.e.) were the most common genera. The harpacticoid copepod community within the pneumatophores was highly variable; in three replicates, fewer than four Canuella spp. individuals were identified but in the fourth there were 126; low numbers of Brianolla spp. (3 ± 2, mean ± s.e.) and Coullana spp. (5 ± 2, mean ± s.e.) were also present. Harpacticoid copepod communities were significantly different between sand breaks, mangrove, pneumatophores at the mangrove edge, and intertidal pools (PERMANOVA3,16, Pseudo-F = 8.1975, P = 0.0003). Differences in assemblages were observed between the intertidal pools and sand break, intertidal pools and mangroves, and sand break and mangroves (Table 4). The differences between these groups were quite noticeable (Fig. 9). SIMPER analysis showed that Coullana spp. abundance was the primary contributor to variation among sand break and intertidal pool samples (SIMPER, average dissimilarity = 43.92, contribution = 51.73%), sand break and mangrove samples (SIMPER, average dissimilarity = 45.26, contribution = 72.55%), and mangrove and intertidal pool samples (SIMPER, average dissimilarity = 62.83, contribution = 77.03%). Canuella spp. and Brianolla spp. also had a considerable effect on the differences among sediment samples (Fig. 9).
The four main genera of harpacticoid copepods stained with Rose Bengal, that were observed in sediment samples. (a) Coullana spp., (b) Brianolla spp., (c) Canuella spp., and (d) Ectinosoma spp., at 10× magnification.
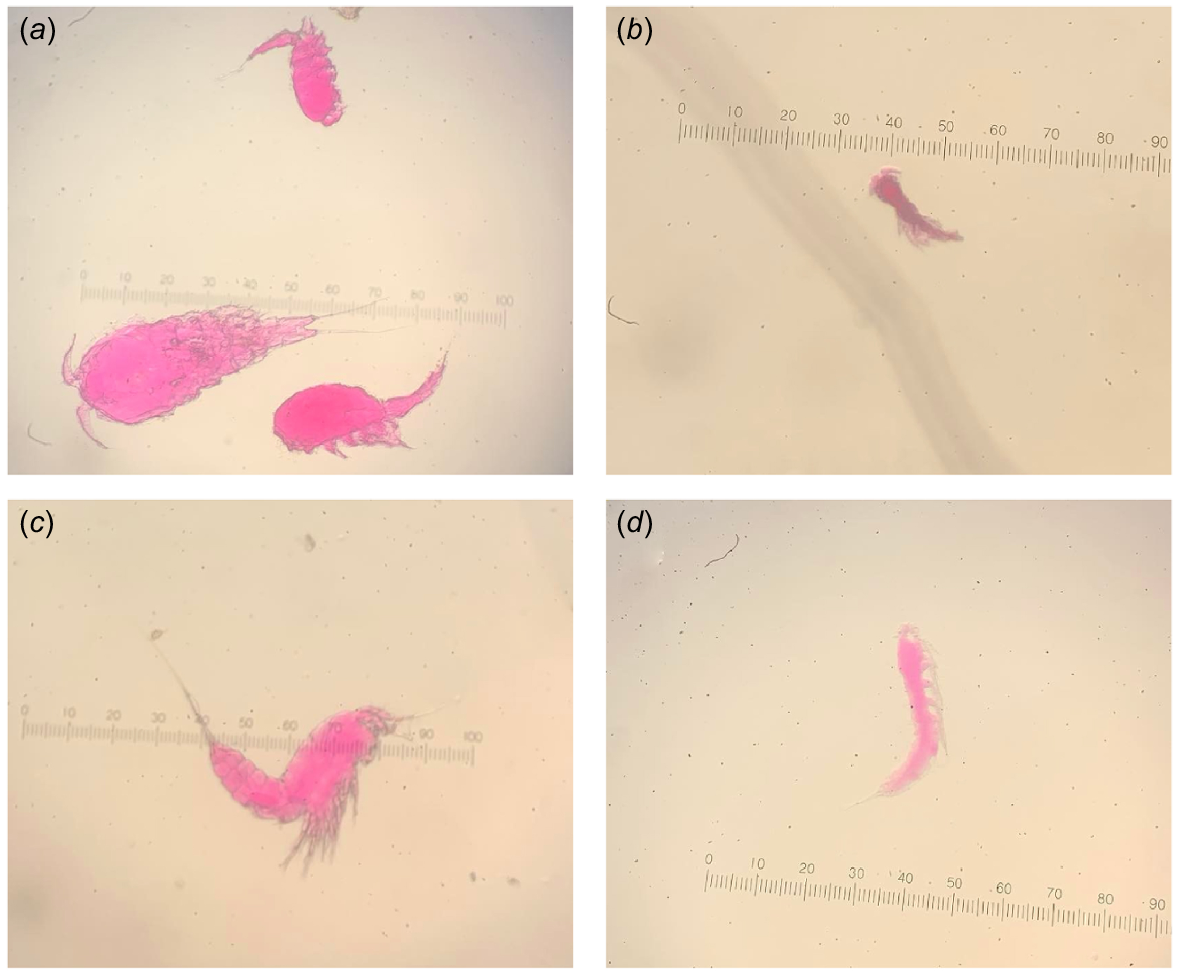
The average number (±s.e.) of Canuella spp. (white), Brianolla spp. (grey), and Coullana spp. (black) observed across a sand break, intertidal pools (control), mangroves, and the pneumatophores at the interface between sandflat and mangroves.
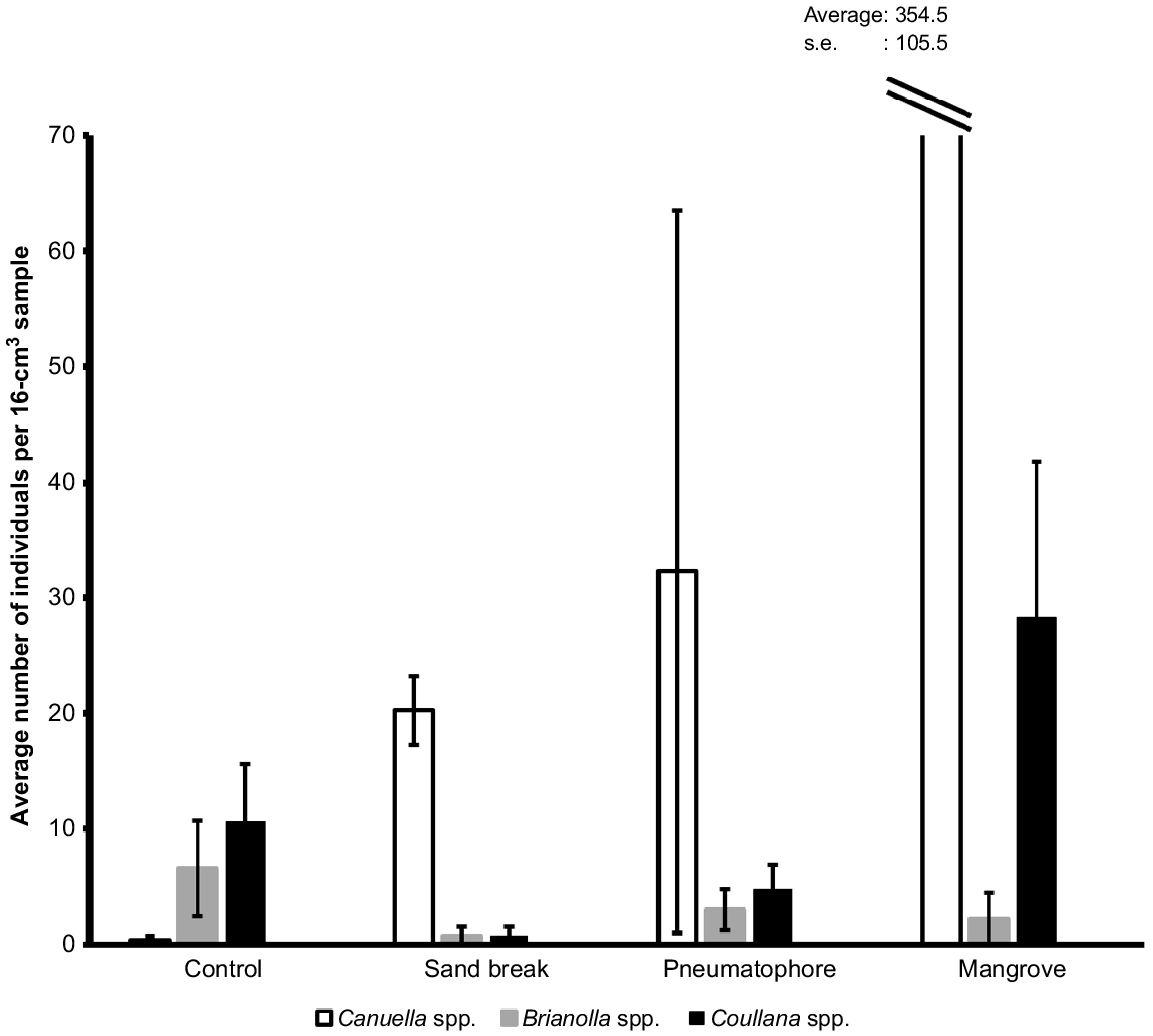
Comparison | t-value | P-value | |
---|---|---|---|
Intertidal pools – sand break | 4.47 | 0.0014* | |
Intertidal pools – pneumatophore | 1 | 0.4324 | |
Intertidal pools – mangrove | 3.88 | 0.0027* | |
Sand break – pneumatophore | 2.17 | 0.0592 | |
Sand break – mangrove | 3.85 | 0.0269* | |
Pneumatophore – mangrove | 1.9 | 0.1141 |
Probabilities are significant at: *, P < 0.05.
A multidimensional scaling (MDS) ordination plot of harpacticoid communities across the following four habitats: pneumatophores, intertidal pools, sand breaks, and mangroves, along a section of One Mile Beach, Dunwich. Vectors indicate the loadings of four harpacticoid genera observed within the habitats. Each symbol represents one sediment sample. Stress is 0.08.
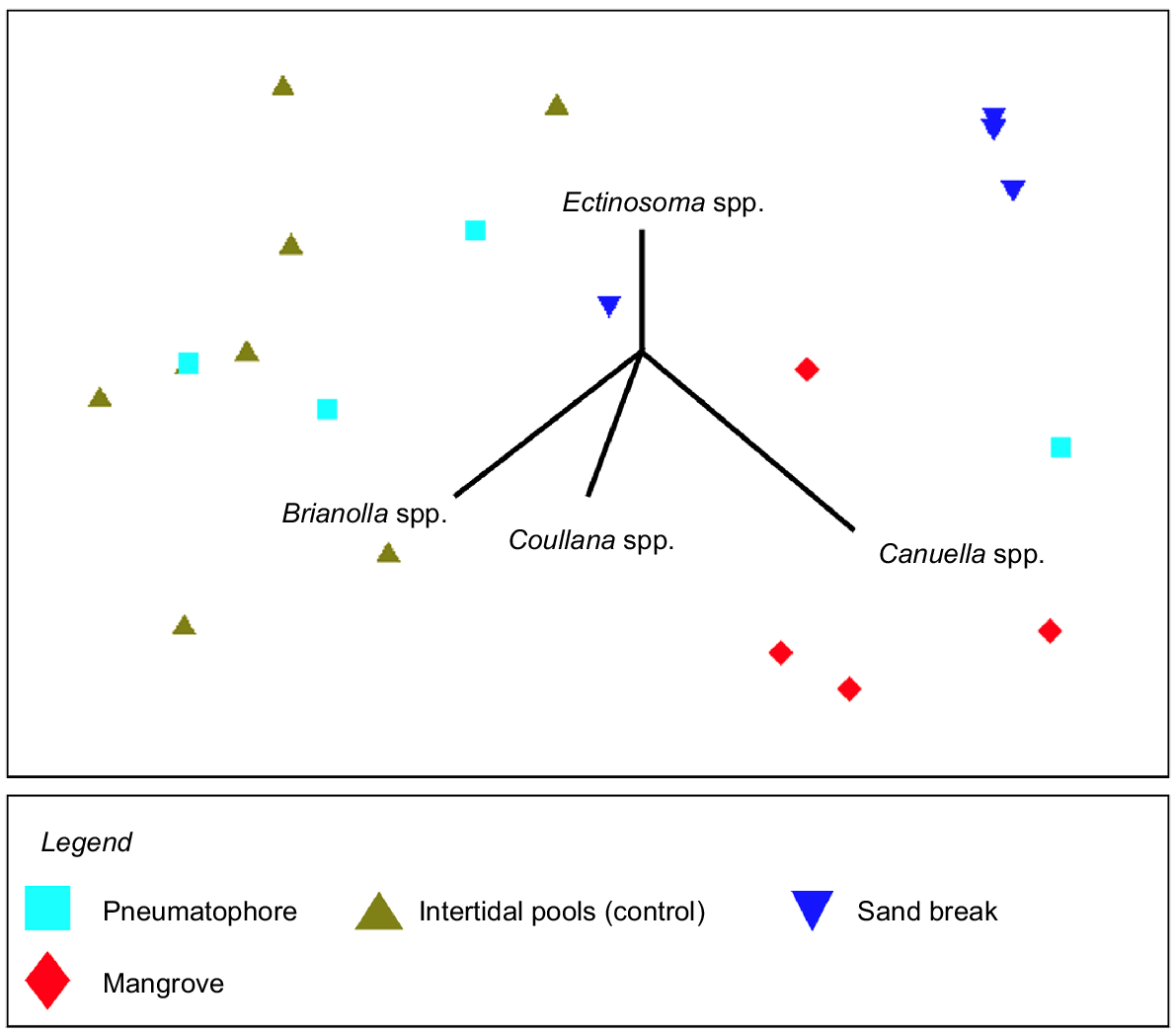
Proportion-transformed harpacticoid copepod abundance data from sediment and gut samples showed significant differences between harpacticoid copepod communities (PERMANOVA5,32, pseudo-F = 13.443, P < 0.0001). Pairwise comparison of these different groups determined that there were significant differences between sand-break sediment samples and intertidal pool samples, mangrove samples and intertidal pool samples, and among all habitats, except pneumatophore sediment samples, and gut samples before and after high tide (Table 5). Again, differences between different habitat sediment samples and whiting guts were clear (Fig. 10). The dissimilarity between sand breaks and mangroves samples, and outgoing whiting gut samples, was largely driven by Coullana spp. (50.71 and 47.13% contribution to dissimilarity respectively), which was more abundant in the gut samples and Canuella spp. (41 and 46.04% contribution respectively), which were more abundant in sediment samples. There was also a difference in the harpacticoid copepod communities in the guts of whiting caught before high tide and in the sediments of their low-tide refuge, intertidal pools, which was primarily driven by the presence of Brianolla spp. (44.77% contribution) in the sediment. The gut contents of the whiting did not change between those caught before and after high tide, suggesting that they consumed the same prey (Table 4). Average gut fullness also did not vary among groups of whiting (t18 = 0.82, P = 0.4218).
Comparison | t-value | P-value | |
---|---|---|---|
Intertidal pools – sand break | 5.29 | 0.0021* | |
Intertidal pools – pneumatophore | 1.31 | 0.2412 | |
Intertidal pools – mangrove | 4.82 | 0.0019* | |
Sand break – pneumatophore | 2.25 | 0.1371 | |
Sand break – mangrove | 0.58 | 0.6581 | |
Pneumatophore – mangrove | 1.96 | 0.1431 | |
Intertidal pools – guts after high tide | 3.33 | 0.0008* | |
Intertidal pools – guts before high tide | 2.78 | 0.0083* | |
Sand break – guts after high tide | 7.77 | 0.0019* | |
Sand break – guts before high tide | 7.95 | 0.0014* | |
Pneumatophore – guts after high tide | 1.93 | 0.0521 | |
Pneumatophore – guts before high tide | 1.61 | 0.111 | |
Mangrove – guts after high tide | 7.31 | 0.0018* | |
Mangrove – guts before high tide | 7.89 | 0.0025* | |
Guts after high tide – guts before high tide | 0.66 | 0.6384 |
Probabilities are significant at: *, P < 0.05.
A multidimensional scaling (MDS) ordination plot of the proportion of harpacticoid genera across the following four habitats: pneumatophores, intertidal pools, sand breaks, and mangroves and in smaller (<25 mm) juvenile whiting guts before and after high tide. Vectors indicate the loadings of four harpacticoid genera, and an additional ‘other’ group for individuals that could not be identified. Each symbol represents one 16-cm3 sediment sample or one gut sample; all samples were collected at One Mile Beach, Dunwich. Stress is 0.08.
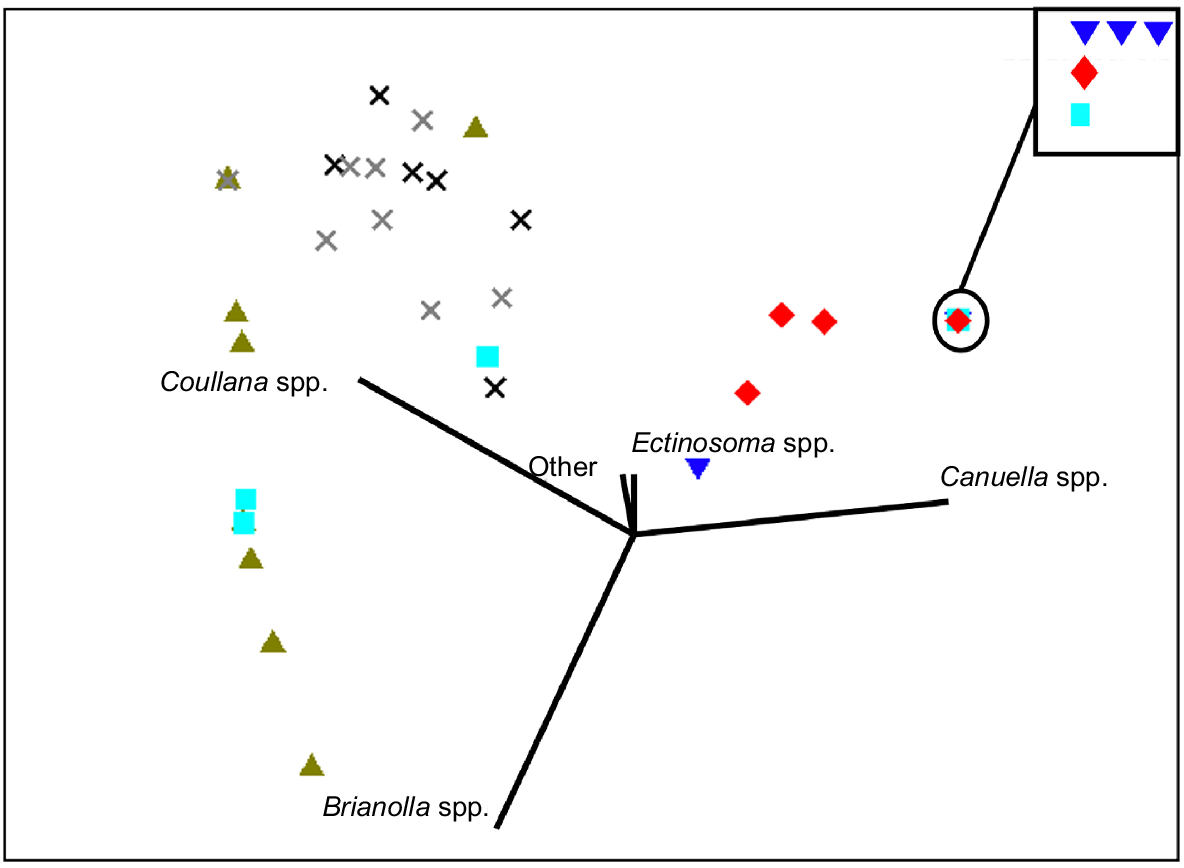
Observations on the visibility of whiting and potential predators
Although numerical measurements of interactions between whiting and their potential predators, and whiting camouflage, were not taken, several important observations from funnel-trap videos were noted. Smaller juvenile whiting were observed preceding several piscivorous predators, including large Favonigobius spp. (>40 mm), Platycephalus spp. (50–150 mm) and Pseudorhombus spp. (50–150 mm), on the incoming tide, and following these fish out on the outgoing tide. However, migrating larger juveniles were observed in proximity with Platycephalus spp. (Fig. 11) and Pseudorhombus spp. These larger juveniles also tended to occupy darker habitats, including among pneumatophores, where they stood out from their environment. By contrast, smaller juveniles were difficult to spot against the lighter-coloured sandy sediments (Fig. 12) and often they were spotted only because of their movement.
Cropped image from a funnel-trap recording that demonstrates the proximity of predatory flathead (Platycephalus spp.; red arrow) and larger juvenile whiting at the interface between sandflat and mangrove.
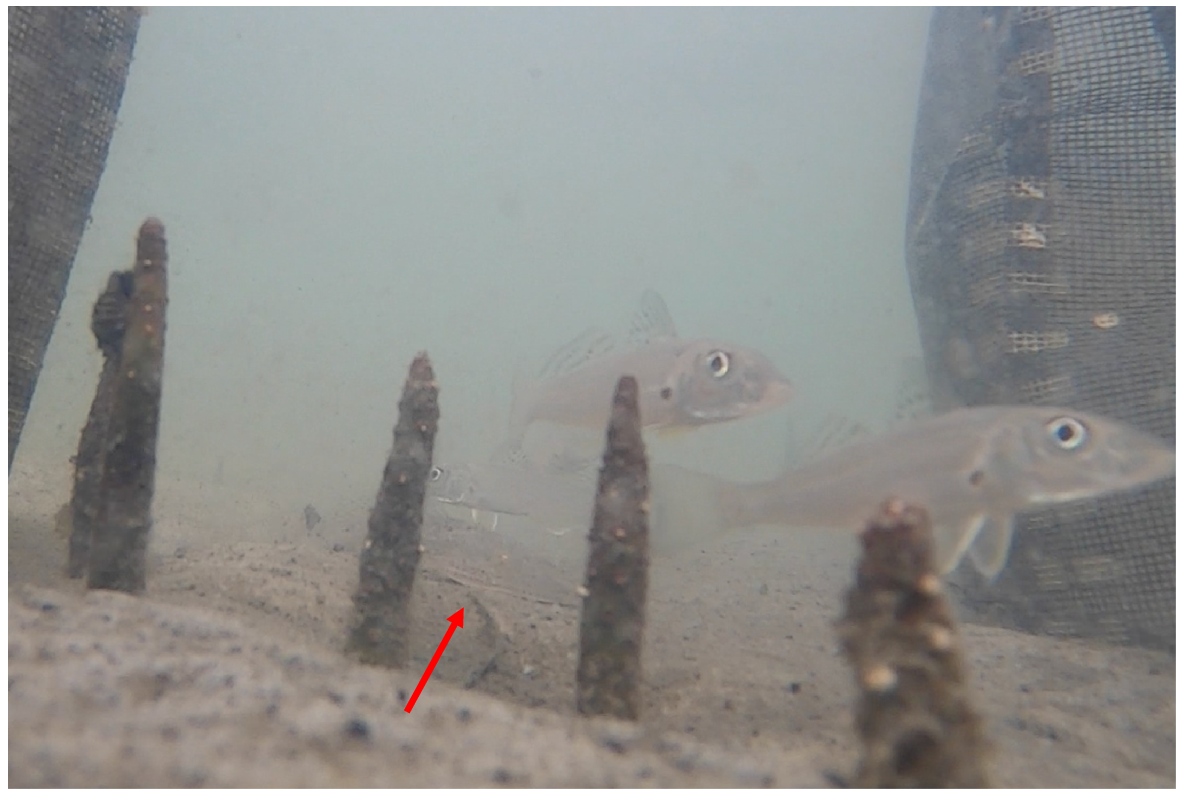
Cropped image from a funnel trap, demonstrating the effective camouflage of these fish in sandy habitats. Six smaller whiting juveniles are present in this image, but only one is easily visible against the black background of the trap wall. These fish were observed only because of their movement leading up to this still image.
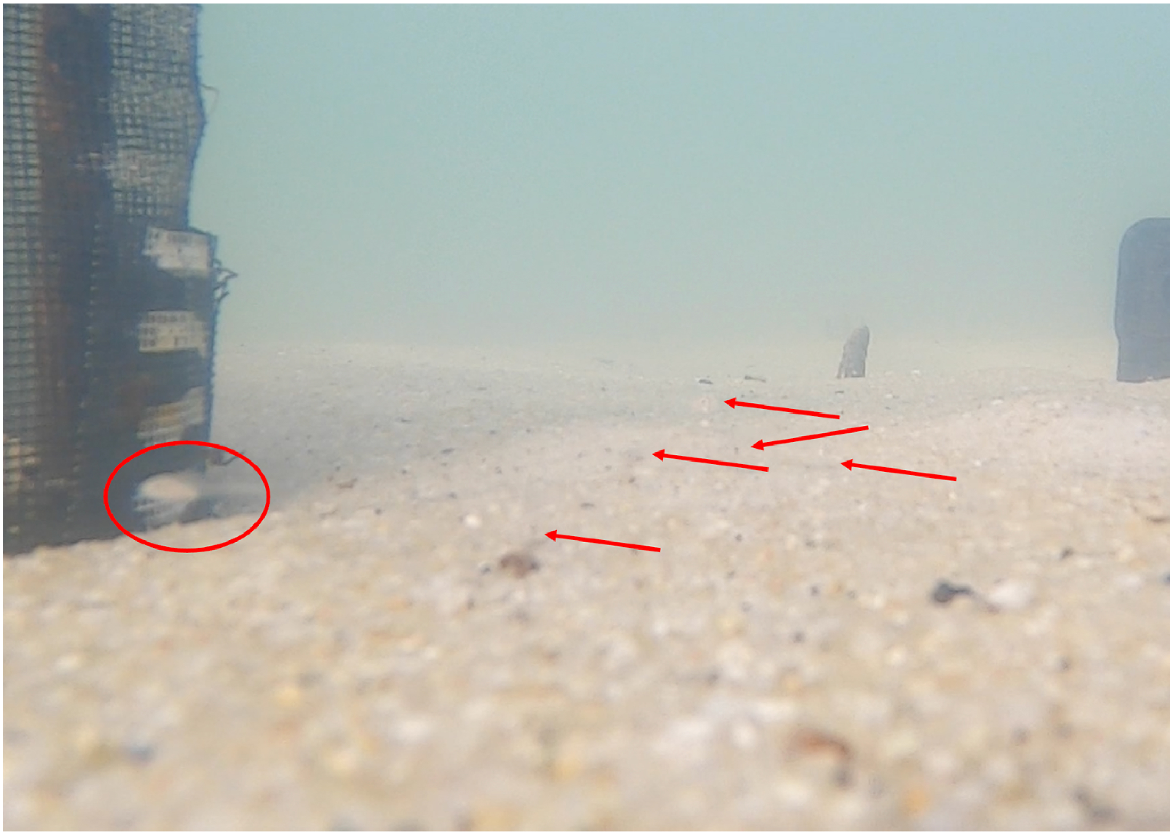
Discussion
The nursery seascape of juvenile whiting in eastern Moreton Bay is complex, with smaller (<25 mm) and larger (>25 mm) juveniles differing in the upper-shore habitats utilised, the paths by which they enter and exit upper-shore habitats, and the depths of water in which they make such movements, supporting all three hypotheses. On ebb and flood tides, smaller juveniles avoided mangrove and intertidal seagrass habitats, instead accessing, and leaving the upper shore via sand channels, the interface between sand breaks and sandflats, at shallow 15–<100-mm depths. Larger juveniles accessed mangroves from the sandflats at greater depths of 150–<300 mm.
The use of different habitat interfaces and timing of migration by smaller and larger juvenile whiting individuals reflect a significant ontogenetic shift where they also experience a change in low-tide refugia and diet (Krück et al. 2009). Smaller juveniles almost entirely avoided vegetated habitats, including mangroves and intertidal seagrass, and instead were frequently observed transiting sandy habitats. These smaller juveniles demonstrated effective colour matching in these sandy habitats, likely providing effective camouflage against visually hunting predators (Kjernsmo and Merilaita 2012; Price et al. 2019). This may suggest that predator avoidance is an ecological benefit driving this migration. The shallow depths utilised by these fish would also assist in predator avoidance because larger predators require deeper water (Bretsch and Allen 2006a, 2006b). Elevated predation rates on small fishes in part drives the elevated mortality experienced at younger ages (Type III mortality) displayed by some fish species (Caley 1998; Hixon and Jones 2005). This can encourage small fish to utilise shallow, colour-matching habitats to avoid larger aquatic predators (Rypel et al. 2007; Davis et al. 2017; Boswell et al. 2019).
Shallow-water migrations may help avoid predators such as Favonigobius spp., Platycephalus spp. and Pseudorhombus spp. It is possible that small juvenile whiting individuals utilise additional predator-avoidance strategies that do not require shallow-water occupation (Kelley and Magurran 2003; Kelley et al. 2017). Afterall, the total number of small juvenile whiting observed transiting upper-shore habitats was relatively low (2.71 whiting min−1 ± 1.12, mean ± s.e.), given the large numbers of fish that aggregate in tidepools (Krück et al. 2009; Chargulaf et al. 2011). Only a small proportion of smaller juveniles moves into nearby upper sandy habitats at shallow depths. Furthermore, these results suggest that juvenile whiting avoid the upper-shore vegetated habitats at less-disturbed, mangrove-lined shores and it is still unknown where these fish find refuge. A parsimonious explanation would be that most of the smaller juvenile whiting individuals remain on the sandflat around intertidal pools, where they rely on colour-matching to avoid predation. The idea that small juvenile whiting individuals occupy sandy habitats is supported by the similarity between harpacticoid copepods in the guts of whiting and those found in sand breaks and intertidal pool sediment samples.
Analysis of harpacticoid copepods in the guts of smaller individuals supported the hypothesis that the diet of small juvenile whiting individuals would resemble potential high-tide foraging habitats. The harpacticoid copepod communities in the sediments at genus level were different among sand breaks, mangroves, and intertidal pools, with no difference between these habitats and the sediment between pneumatophores at the boundary of mangroves and the sand flat. This confirmed previous work that harpacticoid communities are highly variable spatially (Findlay 1981; Coull 1999; Azovskii and Chertoprud 2002; Stringer et al. 2012; Chargulaf and Tibbetts 2015; Ghosh and Mandal 2019). The large variation, particularly in Coullana spp. and Canuella spp., provides an opportunity to infer high-tide foraging habitat of meiobenthivorous smaller juvenile whiting. Although cluster analysis identified similarities between pneumatophores and the gut contents of whiting caught before and after high tide; this finding was considered a coincidence because of the great variability among the sediment samples and the inability of whiting to forage here before high tide. These results indicated that juvenile whiting selectively target and consume Coullana spp. over other genera such as Brianolla spp. and Canuella spp. commonly found in the sediment.
The harpacticoid copepod community represented in the gut contents of smaller juvenile whiting individuals did not match the community represented in most of the sediments in potential foraging grounds. The upper-shore sediment harpacticoid copepod community was dominated by Canuella spp., whereas juvenile whiting appear to be selectively feeding on Coullana spp., which are more common in intertidal pool and mangrove sediments. Additionally, the mean gut fullness of smaller juveniles caught on the outgoing tide did not differ significantly from those captured on the incoming tide, indicating that whiting individuals had fed both during the 5–6 h that they occupied the upper shore and in the 6–7 h they spent in intertidal pools. The comparison of gut fullness values and the proportions of different harpacticoid copepod genera in the guts of smaller juvenile whiting individuals indicated that they continued to feed during the high tide and on the same harpacticoid genera (Coullana spp.). This is assuming a gut residence time of 6 h, which was observed in Krück et al. (2009). This partially supports the hypothesis that the harpacticoid genera observed in the gut of smaller juveniles would resemble the genera observed in high-tide foraging habitat. Coullana spp. was the most common genus found in the guts of whiting caught after high tide and were the dominant taxon observed in intertidal pools but were also common in mangrove sediments. Given the lack of smaller juveniles observed moving into mangroves, it stands to reason that these fish consumed these harpacticoid copepods while foraging on the sand flat. This supports the conclusion that most of the small juveniles remain on the sandflat during the high tide. It is possible that the lack of Coullana spp. in sandy upper-shore habitats is a result of top–down prey depletion of these harpacticoid copepods. Although this is unlikely, because we would have observed a similar lack of Coullana spp. in the intertidal pools where these fish reside and forage at low tide. Furthermore, Coull (1999) suggested the rate of reproduction of harpacticoid copepods is too high for the population to be controlled by predation.
Larger juvenile whiting individuals differed from their smaller counterparts by preferentially migrating into mangrove habitats at greater depths. These fish migrated at depths more than 15 cm and, as a result, were frequently observed in the presence of mesopredators such as juvenile Platycephalus spp. (50–120 mm) and Pseudorhombus spp. (50–120 mm). This could suggest that the avoidance of mesopredators is not a likely driver in the migration of larger juvenile whiting, but of course they may still be avoiding even larger predators. This subsequent hypothesis is further supported because the mangrove habitats that these fish move into contain darker, siltier, and organic-rich sediments as well as dark pneumatophores and mangrove trunks (Bulmer et al. 2017), which contrast the lighter colouration of whiting. However, the complexity of mangrove structures (roots, pneumatophores, stumps) does offer small fishes places to shelter and hide from predators (Nanjo et al. 2014). A detailed study investigating other potential drivers, such as additional food resource availability, is required to better understand the fish migration patterns observed here.
There remain several unanswered questions regarding how and why these fish utilise different intertidal nursery seascape features. This is reflected by the high degree of variance observed among sites and months. Despite sampling being conducted over relatively small spatial (within 3 km of Dunwich township) and temporal (over 5 months) scales, there were noticeable changes in the number of juvenile whiting observed and the habitats they utilised across these variables. Spatially, the sites sampled in this study did vary in the habitats they provided, including differing mangrove densities and the presence and absence of seagrass and sand channels. However, there were also several minor differences, including differing levels of boat wake, fishing pressure (of mesopredators), different-coloured sediments, differences in hydrology (particularly freshwater inflows) and differing shoreline orientations that affect the level of exposure to wind and waves. All these factors, and probably more, are likely to influence how juvenile fish utilise their intertidal nursery seascape and speak to the dynamic and complex nature of nursery seascapes. Temporally, differences are likely to come about because of the growth and development of the juvenile cohort, which typically has peak recruitment into intertidal areas around October and November, but continues to March (Chargulaf et al. 2011; Ochwada-Doyle et al. 2014). As discussed, these fish undergo significant ontogenetic shifts and, given they can grow in excess of 10 cm in their first year (Ochwada-Doyle et al. 2014), we predict that habitat usage of the cohort changes dramatically from spring to the end of summer. Although our study was unable to identify all the variables, conditions and patterns of nursery seascape use by juvenile whiting individuals, it has identified several trends that may form the basis of future studies.
In summary, both smaller juvenile and larger juvenile whiting individuals migrate up the shore with the tides, the former in shallower water to sandy, unvegetated habitats, and the latter in deeper water to mangrove habitats. Migration of smaller juveniles seems more likely to be driven by predator avoidance than by food availability, as indicated by analysis of their diet, whereas for larger juveniles, predator avoidance may be of lesser importance. This study has provided further evidence for the importance of intertidal sandflats and their mosaic of habitat patches as a nursery seascape for juvenile whiting and likely many other juvenile fish species. It has also indicated that several additional factors may be involved in determining the nursery value of an intertidal flat to juvenile whiting. The interaction between two distinct juvenile life-history stages with differing habitat patches is complex, but can be used to inform management decisions. Given that current stock levels of whiting are less than half of the 60% unfished biomass target outlined in Queensland’s Sustainable Fishing Strategy 2017–2027, management strategies that protect the nursery habitats of whiting post-larvae should be implemented and such habitats protected.
Acknowledgements
We thank the staff at Moreton Bay Research Station for their hospitality, Quandamooka Yoolooburrabee Aboriginal Corporation for permission to conduct this study on their Sea Country, Sarah Goh for assistance in the field, Dr Kennedy Wolfe and Dr Matthew Luskin for comments on a draft manuscript.
References
Adkins ME, Simpfendorfer CA, Tobin AJ (2016) Large tropical fishes and their use of the nearshore littoral, intertidal and subtidal habitat mosaic. Marine and Freshwater Research 67, 1534-1545.
| Crossref | Google Scholar |
Armenteros M, Pérez-García JA, Pérez-Angulo A, Williams JP (2008) Efficiency of extraction of meiofauna from sandy and muddy marine sediments. Revista de Investigaciones Marinas 29, 113-118.
| Google Scholar |
Azovskii AI, Chertoprud ES (2003) Spatio-temporal dynamics of the White Sea littoral harpacticoid community. Oceanology 43, 103-111.
| Google Scholar |
Bates D, Mächler M, Bolker B, Walker S (2015) Fitting linear mixed-effects models using lme4. Journal of Statistical Software 67, 1-48.
| Crossref | Google Scholar |
Birt MJ, Tibbetts IR (2007) Temperature changes in the tidal excursion front and surface sediment of a subtropical mudflat in autumn. Journal of Sea Research 58, 113-119.
| Crossref | Google Scholar |
Boswell KM, Kimball ME, Rieucau G, Martin JGA, Jacques DA, Correa D, Allen DM (2019) Tidal stage mediates periodic asynchrony between predator and prey nekton in salt marsh creeks. Estuaries and Coasts 42, 1342-1352.
| Crossref | Google Scholar |
Bretsch K, Allen DM (2006a) Effects of biotic factors on depth selection by salt marsh nekton. Journal of Experimental Marine Biology and Ecology 334, 130-138.
| Crossref | Google Scholar |
Bretsch K, Allen DM (2006b) Tidal migrations of nekton in salt marsh intertidal creeks. Estuaries and Coasts 29, 474-486.
| Crossref | Google Scholar |
Broekhuysen GJ (1940) A preliminary investigation of the importance of desiccation, temperature, and salinity as factors controlling the vertical distribution of certain intertidal marine gastropods in False Bay, South Africa. Transactions of the Royal Society of South Africa 28, 255-292.
| Crossref | Google Scholar |
Bucher D, Saenger P (1994) A classification of tropical and subtropical Australian estuaries. Aquatic Conservation: Marine and Freshwater Ecosystems 4, 1-19.
| Crossref | Google Scholar |
Bulmer RH, Schwendenmann L, Lohrer AM, Lundquist CJ (2017) Sediment carbon and nutrient fluxes from cleared and intact temperate mangrove ecosystems and adjacent sandflats. Science of The Total Environment 599–600, 1874-1884.
| Crossref | Google Scholar |
Caley MJ (1998) Age-specific mortality rates in reef fishes: evidence and implications. Austral Ecology 23, 241-245.
| Crossref | Google Scholar |
Chargulaf CA, Tibbetts IR (2015) Spatial and temporal variation of meiofauna community structure in soft-sediment pools around Moreton Bay, Australia. Australian Journal of Zoology 63, 204-213.
| Crossref | Google Scholar |
Chargulaf CA, Townsend KA, Tibbetts IR (2011) Community structure of soft sediment pool fishes in Moreton Bay, Australia. Journal of Fish Biology 78, 479-494.
| Crossref | Google Scholar |
Coull BC (1999) Role of meiofauna in estuarine soft-bottom habitats. Austral Ecology 24, 327-343.
| Crossref | Google Scholar |
Coull BC, Greenwood JG, Fielder DR, Coull BA (1995) Subtropical Australian juvenile fish eat meiofauna: experiments with winter whiting Sillago maculata and observations on other species. Marine Ecology Progress Series 125, 13-19.
| Crossref | Google Scholar |
Crook DA, Lowe WH, Allendorf FW, Eros T, Finn DS, Gillanders BM, Hadwen WL, Harrod C, Hermoso V, Jennings S, Kilada RW, Nagelkerken I, Hansen MM, Page TJ, Riginos C, Fry B, Hughes JM (2015) Human effects on ecological connectivity in aquatic ecosystems: integrating scientific approaches to support management and mitigation. Science of The Total Environment 534, 52-64.
| Crossref | Google Scholar |
Davis JP, Pitt KA, Fry B, Connolly RM (2015) Stable isotopes as tracers of residency for fish on inshore coral reefs. Estuarine, Coastal and Shelf Science 167, 368-376.
| Crossref | Google Scholar |
Davis JP, Pitt KA, Olds AD, Harborne AR, Connolly RM (2017) Seagrass corridors and tidal state modify how fish use habitats on intertidal coral reef flats. Marine Ecology Progress Series 581, 135-147.
| Crossref | Google Scholar |
Ellis WL, Bell SS (2008) Tidal influence on a fringing mangrove intertidal fish community as observed by in situ video recording: implications for studies of tidally migrating nekton. Marine Ecology Progress Series 370, 207-219.
| Crossref | Google Scholar |
Espadero ADA, Nakamura Y, Uy WH, Tongnunui P, Horinouchi M (2020) Tropical intertidal seagrass beds: an overlooked foraging habitat for fishes revealed by underwater videos. Journal of Experimental Marine Biology and Ecology 526, 151353.
| Crossref | Google Scholar |
Findlay SEG (1981) Small-scale spatial distribution of meiofauna on a mud- and sandflat. Estuarine, Coastal and Shelf Science 12, 471-484.
| Crossref | Google Scholar |
Ghosh M, Mandal S (2019) Does vertical distribution of meiobenthic community structure differ among various mangrove habitats of Sundarban Estuarine System? Regional Studies in Marine Science 31, 100778.
| Crossref | Google Scholar |
Gray CA, Kennelly SJ (2003) Catch characteristics of the commercial beach-seine fisheries in two Australian barrier estuaries. Fisheries Research 63, 405-422.
| Crossref | Google Scholar |
Hindell JS, Jenkins GP (2004) Spatial and temporal variability in the assemblage structure of fishes associated with mangroves (Avicennia marina) and intertidal mudflats in temperate Australian embayments. Marine Biology 144, 385-395.
| Crossref | Google Scholar |
Hixon MA, Jones GP (2005) Competition, predation, and density-dependent mortality in demersal marine fishes. Ecology 86, 2847-2859.
| Crossref | Google Scholar |
Kelley JL, Magurran AE (2003) Learned predator recognition and antipredator responses in fishes. Fish and Fisheries 4, 216-226.
| Crossref | Google Scholar |
Kelley JL, Taylor I, Hart NS, Partridge JC (2017) Aquatic prey use countershading camouflage to match the visual background. Behavioral Ecology 28, 1314-1322.
| Crossref | Google Scholar |
Kimball ME, Able KW (2012) Tidal migrations of intertidal salt marsh creek nekton examined with underwater video. Northeastern Naturalist 19, 475-486.
| Crossref | Google Scholar |
Kimirei IA, Nagelkerken I, Mgaya YD, Huijbers CM (2013) The mangrove nursery paradigm revisited: otolith stable isotopes support nursery-to-reef movements by Indo-Pacific fishes. PLoS ONE 8, e66320.
| Crossref | Google Scholar |
Kjernsmo K, Merilaita S (2012) Background choice as an anti-predator strategy: the roles of background matching and visual complexity in the habitat choice of the least killifish. Proceedings of the Royal Society of London – B. Biological Sciences 279, 4192-4198.
| Crossref | Google Scholar |
Kneib RT (1993) Growth and mortality in successive cohorts of fish larvae within an estuarine nursery. Marine Ecology Progress Series 94, 115-127.
| Crossref | Google Scholar |
Krück NC, Chargulaf CA, Saint-Paul U, Tibbetts IR (2009) Early post-settlement habitat and diet shifts and the nursery function of tidepools during Sillago spp. recruitment in Moreton Bay, Australia. Marine Ecology Progress Series 384, 207-219.
| Crossref | Google Scholar |
Laegdsgaard P, Johnson CR (1995) Mangrove habitats as nurseries: unique assemblages of juvenile fish in subtropical mangroves in eastern Australia. Marine Ecology Progress Series 126, 67-81.
| Crossref | Google Scholar |
Litvin SY, Weinstein MP, Sheaves M, Nagelkerken I (2018) What makes nearshore habitats nurseries for nekton? An emerging view of the nursery role hypothesis. Estuaries and Coasts 41, 1539-1550.
| Crossref | Google Scholar |
Lovelock C, Accad A, Dowling R, Duke N, Lee SY, Ronan M (2019) Mangroves and saltmarshes of Moreton Bay. In ‘Moreton Bay Quandamooka & Catchment: past, present, and future’. (Eds IR Tibbetts, PC Rothlisberg, DT Neil, TA Homburg, DT Brewer, AH Arthington) pp. 299–218. (Moreton Bay Foundation: Brisbane, Qld, Australia)
Madon SP, Williams GD, West JM, Zedler JB (2001) The importance of marsh access to growth of the California killifish, Fundulus parvipinnis, evaluated through bioenergetics modeling. Ecological Modelling 136, 149-165.
| Crossref | Google Scholar |
Martinho F, Cabral HN, Azeiteiro UM, Pardal MA (2012) Estuarine nurseries for marine fish: connecting recruitment variability with sustainable fisheries management. Management of Environmental Quality 23, 414-433.
| Crossref | Google Scholar |
Mattone C, Bradley M, Barnett A, Konovalov DA, Sheaves M (2022) Environmental conditions constrain nursery habitat value in Australian sub-tropical estuaries. Marine Environmental Research 175, 105568.
| Crossref | Google Scholar |
Meager JJ, Williamson I, King CR (2005) Factors affecting the distribution, abundance and diversity of fishes of small, soft-substrata tidal pools within Moreton Bay, Australia. Hydrobiologia 537, 71-80.
| Crossref | Google Scholar |
Morris S, Taylor AC (1983) Diurnal and seasonal variation in physico-chemical conditions within intertidal rock pools. Estuarine, Coastal and Shelf Science 17, 339-355.
| Crossref | Google Scholar |
Nagelkerken I, Blaber SJM, Bouillon S, Green P, Haywood M, Kirton LG, Meynecke J-O, Pawlik J, Penrose HM, Sasekumar A, Somerfield PJ (2008) The habitat function of mangroves for terrestrial and marine fauna: a review. Aquatic Botany 89, 155-185.
| Crossref | Google Scholar |
Nagelkerken I, Sheaves M, Baker R, Connolly RM (2015) The seascape nursery: a novel spatial approach to identify and manage nurseries for coastal marine fauna. Fish and Fisheries 16, 362-371.
| Crossref | Google Scholar |
Nanjo K, Kohno H, Nakamura Y, Horinouchi M, Sano M (2014) Effects of mangrove structure on fish distribution patterns and predation risks. Journal of Experimental Marine Biology and Ecology 461, 216-225.
| Crossref | Google Scholar |
Ochwada-Doyle FA, Stocks J, Barnes L, Gray CA (2014) Reproduction, growth, and mortality of the exploited sillaginid, Sillago ciliata Cuvier, 1829. Journal of Applied Ichthyology 30, 870-880.
| Crossref | Google Scholar |
Olson AM, Hessing-Lewis M, Haggarty D, Juanes F (2019) Nearshore seascape connectivity enhances seagrass meadow nursery function. Ecological Applications 29, e01897.
| Crossref | Google Scholar |
Price N, Green S, Troscianko J, Tregenza T, Stevens M (2019) Background matching and disruptive coloration as habitat-specific strategies for camouflage. Scientific Reports 9, 7840.
| Crossref | Google Scholar |
Rypel AL, Layman CA, Arrington DA (2007) Water depth modifies relative predation risk for a motile fish taxon in Bahamian tidal creeks. Estuaries and Coasts 30, 518-525.
| Crossref | Google Scholar |
Seitz RD, Wennhage H, Bergström U, Lipcius RN, Ysebaert T (2014) Ecological value of coastal habitats for commercially and ecologically important species. ICES Journal of Marine Science 71, 648-665.
| Crossref | Google Scholar |
Somero GN (2002) Thermal physiology and vertical zonation of intertidal animals: optima, limits, and costs of living. Integrative and Comparative Biology 42, 780-789.
| Crossref | Google Scholar |
Stringer TJ, Korsman JC, Peralta G, Keesing V, Tremblay LA, Glover CN (2012) Effects of environmental gradients on the distribution of harpacticoid copepods in an intertidal flat, Portobello Bay, Otago Harbour, New Zealand. New Zealand Journal of Marine and Freshwater Research 46, 385-397.
| Crossref | Google Scholar |
Sundblad G, Bergström U, Sandström A, Eklöv P (2014) Nursery habitat availability limits adult stock sizes of predatory coastal fish. ICES Journal of Marine Science 71, 672-680.
| Crossref | Google Scholar |
Thomas BE, Connolly RM (2001) Fish use of subtropical saltmarshes in Queensland, Australia: relationships with vegetation, water depth and distance onto the marsh. Marine Ecology Progress Series 209, 275-288.
| Crossref | Google Scholar |
Thomas ORB, Thomas KV, Jenkins GP, Swearer SE (2020) Spatio-temporal resolution of spawning and larval nursery habitats using otolith microchemistry is element dependent. Marine Ecology Progress Series 636, 169-187.
| Crossref | Google Scholar |
Weisberg SB, Whalen R, Lotrich VA (1981) Tidal and diurnal influence on food consumption of a salt marsh killifish Fundulus heteroclitus. Marine Biology 61, 243-246.
| Crossref | Google Scholar |
Weng HT (1983) Identification, habitats and seasonal occurrence of juvenile whiting (Sillaginidae) in Moreton Bay, Queensland. Journal of Fish Biology 23, 195-200.
| Crossref | Google Scholar |