Reproductive phenology of the kelp Ecklonia radiata at its Australian warm-range edge and the influence of environmental factors
R. J. Veenhof

A National Marine Science Centre, Faculty of Science and Engineering, Southern Cross University, Coffs Harbour, NSW, Australia.
B NSW Department of Primary Industries, National Marine Science Centre, Coffs Harbour, NSW, Australia.
Marine and Freshwater Research 74(11) 928-940 https://doi.org/10.1071/MF22259
Submitted: 1 December 2022 Accepted: 14 May 2023 Published: 7 June 2023
© 2023 The Author(s) (or their employer(s)). Published by CSIRO Publishing. This is an open access article distributed under the Creative Commons Attribution-NonCommercial-NoDerivatives 4.0 International License (CC BY-NC-ND)
Abstract
Context: Range-edge populations persist closer to their physiological thresholds, often limiting reproductive output. Kelps are foundation species on temperate reefs, but their reproductive phenology at range edges remains poorly understood.
Aims: We assess the reproductive phenology of Ecklonia radiata at its eastern Australian warm range edge in relation to local environmental conditions and make comparisons with populations throughout Australia.
Methods: E. radiata fertility was monitored over multiple years and environmental predictors of fertility were assessed using generalised additive models. Fertility responses were compared with data from centre and cold range-edge populations.
Key results: The proportion of fertile E. radiata plants increased as daylength declined and the length of fertile tissue was positively correlated with temperature. The magnitude of spore release was variable and responded to episodic swell and rainfall events. This is contrary to spore-release data from centre and cold range-edge populations, which demonstrated seasonal patterns correlated with temperature.
Conclusion: Spore release was lower at the warm range edge, yet year-round spore release demonstrated flexibility in response to local environmental conditions.
Implications: Flexible reproductive phenology of E. radiata at its warm range edge may be advantageous for sustaining populations at low latitudes under climate change.
Keywords: Ecklonia radiata, environmental analysis, fertility, kelp, reproduction, reproductive phenology, spores, warm-range edge.
Introduction
Range-edge populations have long been studied to understand fundamental ecological and evolutionary theories (MacArthur 1972; Sexton et al. 2009). The central abundance theory suggests that population size, reproduction, and genetic variability will decline from the centre of the population towards the range edge (Gaston 2003; Sagarin et al. 2006). This assumes that environmental conditions become less favourable towards the range edges, which will either increase mortality or decrease reproduction (Kawecki 2008). When reproduction is lower than mortality, the population at the edge of a species’ distribution will decline and may contribute to range contractions (Gaston 2003). In practice, many exceptions exist to the pattern of lowered reproductive output at range edges (Parmesan and Hanley 2015; Pironon et al. 2017). This non-conformity can be caused, for example, by different life-history strategies and reproductive plasticity at the range edge (Levin 2012; Almeida et al. 2017; Sinclair et al. 2020). In terrestrial systems, considerable variation in life histories within plant communities facilitates exceptions to the central-abundance theory (Abeli et al. 2014; Shay et al. 2021). When reproductive output at the range edge is higher than or equal to that in centre populations, reproduction is often driven by local environmental conditions rather than large-scale environmental gradients (Lester et al. 2007; Granado-Yela et al. 2013; Zardi et al. 2015; Pironon et al. 2017). Teasing out the environmental drivers that alter patterns of reproduction between centre and range-edge populations can therefore be informative in understanding the underlying mechanisms that structure species range edges and contribute to range shifts.
The timing of reproductive events (or reproductive phenology) can vary throughout a species distribution, and this may be driven by changes in environmental conditions across a species range (Hampe and Petit 2005). In some cases, the way reproductive phenology changes in response to environmental factors may differ between range-edge populations and populations at the centre of species distributions (Lara-Romero et al. 2014; Hargreaves and Eckert 2019; Dangremond et al. 2022). Highly variable reproductive phenology is also often reported at range edges (Sexton et al. 2009; Duarte and Viejo 2018), which may mitigate the negative effects of lower reproductive output, contributing to stable range edges (Hampe and Petit 2005; Hargreaves and Eckert 2014). Reproductive strategies such as asexual reproduction or highly variable reproductive output that prevail at species range edges can make a population more suitable to marginal conditions, but may also leave populations vulnerable to increased environmental stress through lowered genetic variation (Kawecki 2008; Oppliger et al. 2014).
Marine and coastal species that persist across strong environmental gradients provide opportunities to better understand adaptive phenology at range edges (Sagarin et al. 2006; Almeida et al. 2017). Large macroalgae are foundation species that have a variety of life-history strategies, often grow along strong latitudinal gradients (Dayton 1985; Wernberg et al. 2019a) and, in the case of kelps (Laminariaceae), have a heteromorphic life cycle where adult sporophytes alternate with microscopic gametophytes (Pedersen 1981). Kelps underpin temperate ecosystems globally and are undergoing climate-driven declines globally, particularly at their warm range edges (e.g. Wernberg et al. 2016; Assis et al. 2017; Filbee-Dexter et al. 2020; Coleman et al. 2022). Understanding phenology across the range of kelps, but particularly at warm range edges will help predict their resilience and ability for recovery from climate-change impacts. Compared with central populations, range-edge populations of kelps show broad variations in patterns of genetic structure (e.g. Robuchon et al. 2014; Vranken et al. 2021; Coleman et al. 2022), thermal tolerance thresholds (e.g. King et al. 2019; Liesner et al. 2020), recruitment of sporophytes (Muth et al. 2019) and reproductive strategies of the gametophyte (Camus et al. 2021), and sporophyte (Brown et al. 2016).
Differences in kelp reproductive phenology have been compared among some populations, although not explicitly compared across species geographic distributions. Kelp reproductive phenology can either consist of a defined reproductive season or continuous fertility year-round (Kain 1989; Santelices 1990; Liu et al. 2017). Fertile seasons are often governed by growth cycles, which in turn are induced by environmental cues unaffected by environmental change such as daylength (e.g. Amsler and Neushul 1989; tom Dieck 1991). By contrast, species that are continuously fertile can display peaks in fertility (e.g. spore release) tied to environmental factors such as temperature (Reed et al. 1996; Bartsch et al. 2013; Mohring et al. 2013a), increased water motion (Gordon and Brawley 2004; McConnico and Foster 2005) and nutrients (Reed et al. 1996). In continuously reproducing kelps, environmental cues can cause synchronous spore release, which promotes long-distance dispersal and recolonisation (Reed et al. 1997; Graham 2003; Edwards 2022). Seasonal fertility can have the advantage of investing energy in reproduction only when it is most likely to be successful, which is often the case for species growing in environments characterised by strong seasonality (but see Buschmann et al. 2004; Liu et al. 2017). Conversely, continuous fertility allows for a more flexible response to environmental conditions, which may be of advantage to kelps growing in locations where environmental conditions are less favourable (Hoffmann 1987; Buschmann et al. 2006), such as range edges.
The kelp Ecklonia radiata is the main canopy-forming kelp growing throughout Australia’s temperate coastlines and is experiencing local declines because of heatwaves, eutrophication, and direct and indirect effects of ocean warming (Johnson et al. 2011; Wernberg et al. 2013; Vergés et al. 2016; Wernberg et al. 2016; Carnell and Keough 2019). The warmer, equator-ward edges of this population represent some of the most thermally tolerant kelp populations globally (Wernberg et al. 2019b) but are also experiencing more frequent and intense climate disturbances (Hobday and Lough 2011). Sporophyte fertility patterns have not been assessed at the warm range edges of E. radiata. In other parts of the Australian E. radiata distribution, fertility appears seasonal and is influenced by seasonally varying environmental factors, such as temperature (Mohring et al. 2013a; Tatsumi et al. 2022). Interpopulation differences are found where some populations increase (Mohring et al. 2013a) or decrease (Tatsumi et al. 2022) fertility in response to rising temperatures. Warm range edge populations of E. radiata may have different reproductive strategies that may be adapted to conditions that approximate the physiological thresholds for E. radiata, contributing to the realised range edge of this species (Hampe and Petit 2005; Oppliger et al. 2014).
Here, we characterise the reproductive phenology of E. radiata sporophytes from the warm range-edge population off eastern Australia. Through multi-year monitoring of plant fertility, we aim to assess seasonality in E. radiata reproduction and test for correlative relationships between E. radiata sporophyte fertility and a suite of environmental variables. We synthesise our findings with previous studies of E. radiata sporophyte fertility to construct a more complete picture of interpopulation variability in fertility, specifically comparing a warm range-edge population to centre and cold range-edge populations throughout Australia. Through this study, we aim to improve the currently limited understanding of E. radiata phenology throughout its Australian distribution.
Materials and methods
Location and sampling
Field sampling was undertaken fortnightly, weather permitting, over a full 2-year period encompassing July 2020–July 2022, at the following three sites: Diggers Beach, (30°28′S, 153°15′E), Charlesworth Bay Beach, (30°27′S, 153°14′E) and Sawtell Beach (30°37′S, 153°08′E). These three sites were chosen because they represent the warmer range edge of this species. Warm-edge populations can be defined by in situ temperature regimes, which vary by both latitude and depth in marine environments or altitude in terrestrial environments (Hampe and Petit 2005; Habibzadeh et al. 2021). We define warm range edge here as populations occurring in low latitudes of the species distribution that occurs in the upper 10% of the temperature range (Hampe and Petit 2005; Rehm et al. 2015; Wijffels et al. 2018), as well as occurring in the spatial extent that is projected to be lost under the most conservative future climate projections (Rehm et al. 2015; Davis et al. 2022) and that harbour local adaptations characteristic of the warmer range edge (Habibzadeh et al. 2021; Nimbs et al. 2023). Although there are some small and isolated populations of E. radiata at lower latitudes further north (as far as Moreton Island at 27°S), these are found only in deeper water below a thermocline and at a thermal environment similar to the shallow-water populations surveyed here. The forests we sampled are the lowest-latitude continuous and dense functioning canopy-forming forests (Davis et al. 2021, 2022). The three individual sites chosen encompassed ~13 km of coastline and were variably exposed to prevailing swell directions and accessible on snorkel to ensure fortnightly sampling was possible. Data were pooled across sites for analysis of temporal fertility trends and to assess the effects of environmental covariates on fertility, as there was no evidence for differences among sites. However, all models used to analyse temporal fertility and effects of environmental covariates were assessed for spatial collinearity to confirm the absence of an effect of ‘Site’ (see ‘Data analysis’). Sampling was conducted at depths of 2–5 m by randomly selecting five plants along a 25-m transect and removing tissue by cutting 10 cm above the top of the stipe. This allows regeneration from the intercalary meristem located at the base of the thallus (Mabin et al. 2013) and reduces potential long-term impacts of sampling on the adult population. Pilot studies showed that fertile material was almost exclusively only on the top 15 cm of plants. Samples were transported back to the laboratory in a moist dark calico sample bag and processed on the same day.
Fertility metrics
To assess the reproductive phenology of the study population we measured (1) the proportion of all collected plants that were fertile, (2) the length (cm) of fertile tissue and (3) the number of spores that were released per square millimetre of fertile tissue. Plants were cleared of epiphytes by gentle scraping with a blade and visually examined for fertile tissue, which presents as raised and slightly discoloured tissue on the central lamina and upper laterals of the plant. Plants presenting fertile tissue contributed to the total percentage of plants fertile and the length of fertile tissue on the central lamina of these individuals was recorded. The thallus was then left to dry for one hour at room temperature (~19°C). After drying, a hole-punch (950 mm2) was used to take 10 equally distributed cores across the fertile tissue available per plant, which were all placed in 50 mL of 1-μm-filtered, UV-sterilised seawater and gently stirred for 20 min to induce spore release (as per Mohring et al. 2013a; Alsuwaiyan et al. 2019). A haematocytometer was used to count spores from four 1-mL subsamples from each 50-mL spore sample. This established an average number of spores of 1-mL spore solution, which was then related to spores per square millimetre of fertile tissue (as in Mohring et al. 2013a; Tatsumi et al. 2022).
For a 3-month period, the lengths of the thallus, stipe, and entire plant were recorded in situ for each collected plant to test for relationships between fertility and sporophyte morphology. However, correlations between fertility response variables (i.e. proportion of plants fertile, fertile tissue length and spore release) and morphology predictors (i.e. thallus, stipe and total plant length) were weak (r2 < 0.05) and not statically significant (at alpha level 0.05), so effects of plant morphology on fertility were not incorporated into subsequent analyses (Supplementary Table S1).
Environmental covariates
To investigate potential environmental drivers of sporophyte fertility, environmental data were obtained for the sampling period from variable sources (Table 1, Supplementary Fig. S1) and used to assess for correlative relationships with fertility responses. The suite of environmental predictors selected for analyses was based on previously published relationships (e.g. Reed et al. 1996; Gordon and Brawley 2004; Mohring et al. 2013a) and predictors hypothesised to have biological relevance to kelp fertility. Environmental variables were assessed for covariance and only non-covarying (i.e. r2 < 0.5 and >−0.5) environmental variables were retained for statistical analysis (Zuur et al. 2009; Table 1; see Supplementary Table S2 for environmental variables not included in final analysis).
Predictor | Description | Range | Source |
---|---|---|---|
SST | In situ daily sea-surface temperature derived from three daily readings. Averaged over the 2 days prior to each sample date. | 18.37–25.75°C | NSW DPI Fisheries shark-listening station, Park Beach, Coffs Harbour |
Swell height | Swell height from Coffs Harbour Wave Rider buoy. Average for 3 days prior to sample date. | 1.98–0.93 m | Manly Hydraulics Laboratory, located at 30°22′S, 153°16′E |
Wave period | Coffs Harbour Wave Rider buoy. Average for 3 days prior to sample date. | 13.71–7.68 s | Manly Hydraulics Laboratory, located at 30°22′S, 153°16′E |
Daylength | Hours of sunlight daily. | 14.08–10.18 h | Bureau of Meteorology Coffs Harbour station, located at 30°32′S, 153°12′E |
Chlorophyll-a | 8-day composite mass concentration of chlorophyll-a in seawater (level 4). | 0.16–13.11 mg m−3 | Globcolour downloaded from CMEMS (product #009_082). Spatial resolution 4 km |
Temperature anomaly | Sea-level anomaly, maximum intensity of marine heatwave. | 2.31 to −1.63°C | marineheatwaves.org, pixel used located at 30°38′S, 153°13′E |
Storms | Number of days with +3 m waves within 10 days preceding sampling from Coffs Harbour Wave Rider buoy. | 0–2 days | Manly Hydraulics Laboratory, buoy located at 30°22′S, 153°16′E |
Rainfall | Rainfall | 0–29.8 mm2 day−1 | Bureau of Meteorology Coffs Harbour station, located at 30°32′S, 153°12′E |
For every predictor, distinct spatial coordinates were used to extract data for each monitoring site (i.e. Sawtell, 30°37′S, 153°08′E; Charlesworth, 30°27′S, 153°14′E; and Diggers, 30°28′S, 153°15′E) over the sampling period unless otherwise indicated.
Data analysis
We applied a generalised linear modelling (GLM) framework to assess for temporal trends in each of the fertility response variables. The spore-release response variable was analysed with data for infertile plants included and excluded from the analyses. We did this as each response relates to separate aims of this study, given the number of spores that are released into the environment at any given time represents the total reproductive output of the studied population, and the number of spores released by fertile plants throughout the year is indicative of temporal variability of E. radiata fertility in a previously unstudied geography. Each response was modelled as a function of the sample year and month in which data were recorded to assess for temporal patterns among the fertility response variables during the sampling period. GLMs used a logistic link function to model the proportion of plants fertile (i.e. binomially distributed response variable), the number of spores released from all plants (i.e. zero-inflated negative binomially distributed response variable) and the number of spores released by fertile plants (i.e. negative binomially distributed response variable; Zuur et al. 2009). We used an identity link function to relate the length of fertile tissue (i.e. gamma-distributed response variable) to the year and month in which these data were recorded (Zuur et al. 2009). Model terms ‘Year’, ‘Month’ and their interaction were initially included, with individual terms iteratively removed and model quality evaluated using the Akaike information criterion (AIC), where lower AIC values are indicative of reasonably parsimonious models. To assess for temporal trends in the number of spores released from all plants (i.e. a zero-inflated response), model selection involved using a binomial distribution to account for the zero-inflated component of this response and a negative binomial distribution to account for all non-0 data (Zuur et al. 2009). Predicted values from optimal models were obtained to assess temporal trends.
After initial analysis of temporal trends, some fertility responses appeared to covary. Simple linear models were fitted to assess for relationships among these responses. There was a weak (r2 = 0.13) but significant (F1,212 = 31.21, P < 0.01) positive linear relationship between the length of fertile tissue and the proportion of fertile plants (Supplementary Fig. S2a). Additionally, there was a weak (r2 = 0.11) but significant (F1,212 = 25.24, P < 0.001) negative linear relationship between the number of spores from fertile plants and the proportion of fertile plants (Fig. S2b). There was no significant relationship between the number of spores and the length of fertile tissue (r2 < 0.01, F1,212 = 0.61, P = 0.44).
Generalised additive models (GAMs) were applied to test for effects of environmental factors on fertility responses by using the same link functions and distributions as described above. Smoothing functions were removed if the effective degrees of freedom were approximately equal to 1, which is indicative of approximately linear responses (Zuur et al. 2009). AIC-informed backwards model selection was applied to identify the most parsimonious model for each fertile response. Residual plots were assessed visually to confirm that the GAMs and GLMs satisfied assumptions of homogeneity of variance. Model residuals were plotted against ‘sampling location’ (i.e. ‘Site’ with three levels) for all GAMs and GLMs to assess whether this variable should be included as a random effect to account for potential spatial collinearity within the dataset. However, model residuals were randomly distributed in response to sampling location (Supplementary Fig. S3, S4), suggesting that site-level effects were not apparent and confirming a mixed-effects modelling approach was not required. All analyses were undertaken in R (ver. 4.0.3, R Foundation for Statistical Computing, Vienna, Austria, see https://www.R-project.org/) using the pscl package (ver. 1.5.5.1, see https://github.com/atahk/pscl/) to fit zero-inflated GLM (Zeileis et al. 2008) and the mgcv package (ver. 1.8-42, see https://cran.r-project.org/package=mgcv; Wood et al. 2016) to fit all other GAMs and GLMs.
Results
Proportion of fertile plants in sample population
The percentage of plants that were fertile varied among months but not years, indicating consistent changes in the number of fertile plants throughout the sampling period. ‘Month’ was the only explanatory variable included in the optimal temporal model; however, this was not statistically significant (χ2 = 9.41, P = 0.58; Supplementary Table S3). This is most likely due to the model being fitted to a response associated with considerably fewer data points than for other response variables assessed, because each sample date was associated with only one data point for the proportion of fertile plants within the sampled population (Fig. 1, Table S3). Therefore, the black line denoting the fitted values of the model presented in Fig. 1 should be interpreted with caution. Nevertheless, there was a clear trend toward higher numbers of fertile plants between March and July during the Austral autumn and early winter. The lowest number of fertile plants appeared between September and December, which is concurrent with Austral spring.
Results of GLMs relating the proportion of total plants fertile to the factor ‘month’. Dot points represent individual data points, whereas the black line denotes the predicted model output of the optimal GLM. The red line represents the variation in daylength averaged per month as used in the environmental analyses. Note the different y-axis. Sampling year 1 encompassed the period July 2020–July 2021, whereas Sampling year 2 encompassed July 2021–June 2022.
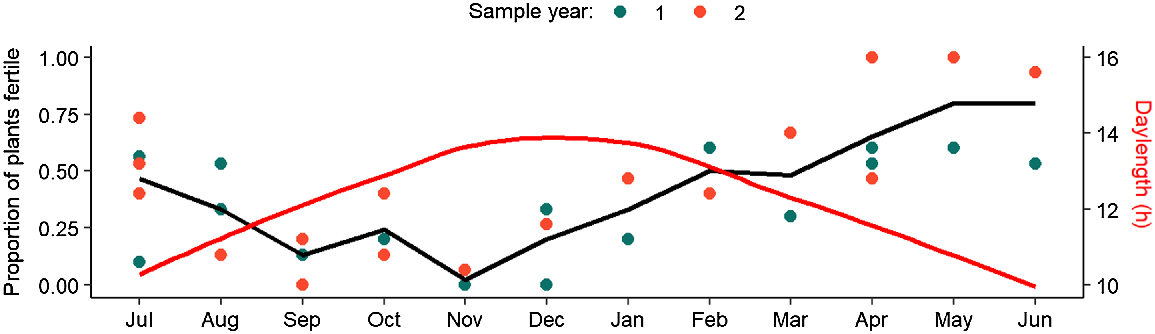
The optimal environmental model for the percentage of plants that were fertile contained daylength as the single predictor (Table S4), providing strong additional evidence for a seasonal pattern in the occurrence of fertile plants and no influence of any of the environmental predictors. The smoothing function was dropped for daylength, favouring a negative linear relationship (coefficient estimate: −0.56; Fig. 2) that was marginally significant (P = 0.05). With increasing daylength, fewer plants were fertile (Fig. 2). This was seen as an observable decrease in the proportion of fertile plants after the winter solstice (June), followed by an increase after the summer solstice (December; Fig. 1).
Length of fertile tissue
The length of fertile tissue on the thallus of plants varied between and within years, but plants displayed fertile tissue year-round (Fig. 3). The optimal temporal model included a significant effect of ‘Month’ (F = 2.329, P = 0.010; Table S3) and ‘Year’ (F = 9.382, P = 0.003; Table S3). The length of fertile tissue was slightly higher in the second sample year than in the first sampling year (Fig. 3). The length of fertile tissue decreased between August and November, during the Austral spring (Fig. 3).
Results of the GLM relating the length of fertile tissue (cm) to month and year. Dot points represent individual data points, whereas the lines represent the predicted model output of the optimal GLM fitted to data from each sample year. Sampling year 1 encompassed the period July 2020–July 2021, whereas Sampling year 2 encompassed July 2021–June 2022. Note missing values for July in Sampling year 1, where fertile tissue length was not recorded initially.
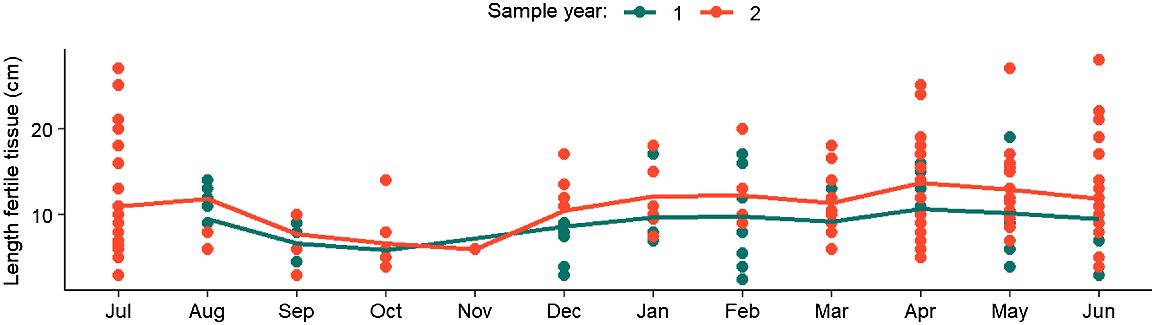
The optimal environmental model for the length of fertile tissue contained the predictors SST, swell height, the number of storm days in the 10 days preceding sampling and chlorophyll-a concentration (Table S4). Each of these factors was a statistically significant predictor of the length of fertile tissue (at alpha level 0.05; Table S5) and demonstrated that both seasonal and non-seasonal ocean factors contribute to the length of fertile tissue in E. radiata at its warm range edge off eastern Australia. For SST, the smoothing function was dropped in favour of a positive linear term (Fig. 4a). The positive relationship between the length of fertile tissue and temperature (Fig. 4a) was driven by differences in fertile tissue between sample years. The second sampling year was characterised by higher SST between January and July (~+1°C degrees; Fig. S1), which coincided with longer fertile-tissue length during this period (Fig. 3). Swell height had a unimodal influence on fertile tissue, with a maximum positive effect on fertile-tissue length occurring at ~1.3 m of swell (Fig. 4b). These intermediate swell heights coincided with longer fertile tissue between March and June of the second sample year (Fig. S1). The occurrence of one storm day in the 10 days preceding sampling had the maximal positive effect on the length of fertile tissue (Fig. 4c). For chlorophyll-a, the smoothing function was dropped to favour a positive linear term (Fig. 4d). This positive effect was driven by multiple small episodic events of high (i.e. >10 mg m3) chlorophyll-a concentration (Fig. S1).
Partial effects of (a) sea-surface temperature (SST, °C), (b) swell height (m), (c) storm days (number in 10 preceding days of sample collection), and (d) chlorophyll-a concentration (mg m−3) on the fitted values of the optimal model for length of fertile tissue. Grey areas denote the 95% confidence interval and rugs (short stripes) on each individual graph represent the observations of fertile-tissue length.
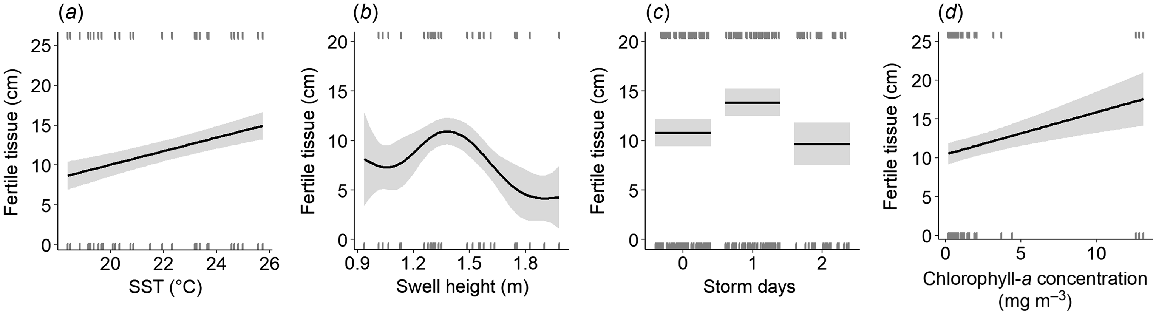
Spore release
Spore-release data showed that spores could be released year-round (Fig. 5). For spore-release data inclusive of infertile plants (i.e. zeros), a zero-inflated model was used consisting of two parts. The part of the optimal temporal model explaining the non-0 data included significant interaction of ‘Year’ and ‘Month’ (χ2 = 82.094, P < 0.01; Table S3). ‘Month’ had a significant effect on the part of the optimal temporal model explaining the non-0 data only (χ2 = 110.42, P < 0.001; Table S3). This indicated variation in the number of spores released both within and between years. Predicted values from this model indicated that the number of spores released each month was higher in the first sampling year than in the second year between February and May, but showed similar trends between June and January for both years (Fig. 5a). In both sampling years, there was a minor peak in spore release in February, which was more pronounced in the first year than in the second. Spore release was highly variable across the sampling periods, except for a period of relatively lower spore release that was apparent between September and November (Austral spring) in both sample years (Fig. 5a).
Results of GLMs relating (a) spores released per square millimetre of all plants to Month and Year, and (b) spores released per square millimetre of fertile plants only to Month and Year covariates. Dot points represent individual data points, whereas lines are the predicted model outputs of the best-fitting GLMs for each response variable. Sampling year 1 encompassed the period of July 2020–July 2021, whereas Sampling year 2 encompassed July 2021–June 2022.
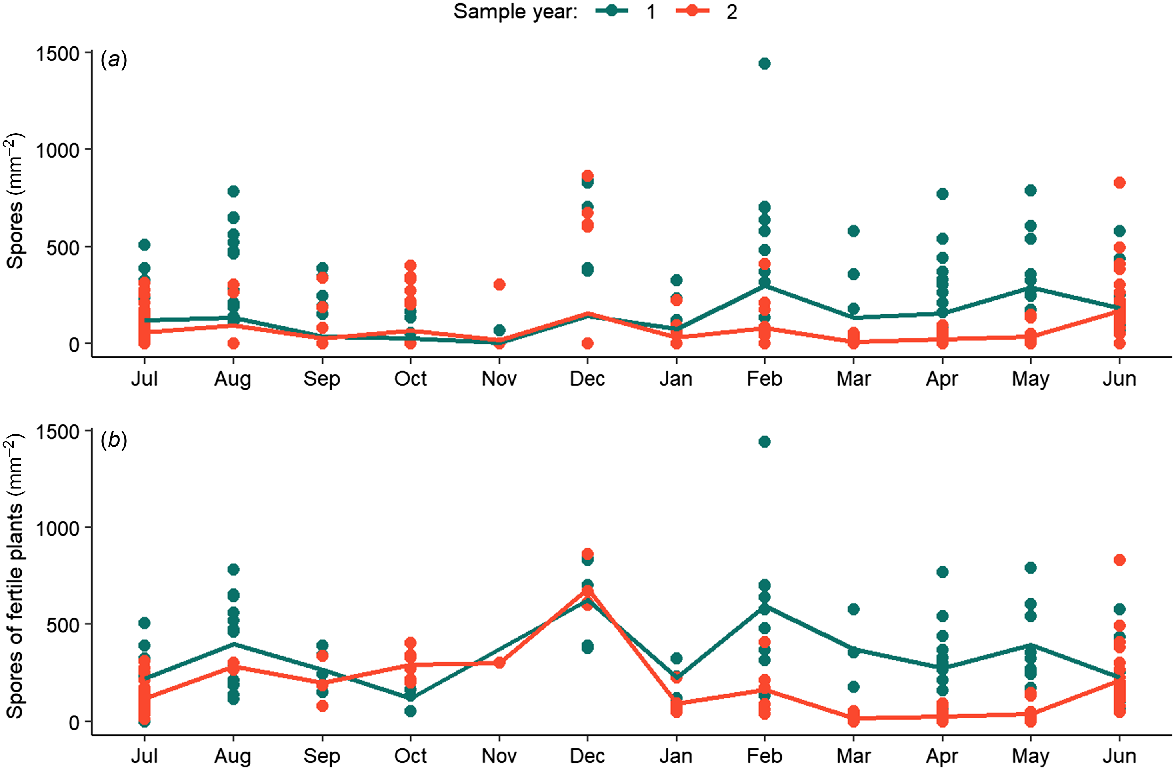
Spore-release data from only fertile plants showed peak patterns slightly different from data with infertile plants included. The optimal temporal model included a significant interaction between ‘Year’ and ‘Month’ (χ2 = 76.652, P < 0.001; Table S3), indicating variable responses for different months between sampling years. The modelled relationship showed that fertile plants release between 200 and 1400 spores mm−2 year-round (Fig. 5b). The peak in February was more pronounced when omitting data of infertile plants, and this peak was higher in the first sample year as opposed to the second (Fig. 5b). This dataset also identified an additional peak in December, which was similar for both sample years, and showed similar trends between years in spore release between June and January. Spore release between February and May was lower in the second sampling year, which is in contrast to the response of fertile-tissue length, which was lower in the first year than in the second sampling year.
The optimal environmental model for the number of spores released from fertile plants contained the predictors swell height, wave period, chlorophyll-a concentration, and rainfall (Table S4). This showed that spore release was driven mostly by environmental factors that are not associated with strong seasonal trends within the study region. Each of these environmental covariates was a statistically significant predictor of spore release (Table S6). Swell height generally had a marginally positive effect on spore release between 1.2 and 1.6 m and had a maximum positive effect at swell heights of ~1.8 m, before declining steeply as swell exceeded this height (Fig. 6a). The level of swell for optimal spore release was ~0.5 m higher than that for optimal fertile length. The influence of wave period appeared unimodal, where wave periods ranging between 9 and 11 s had a positive effect on spore release, and higher values (>11 s) had a negative effect (Fig. 6b). Wave period and swell height were highly variable throughout the sampling period (Fig. S1) and contributed to considerable temporal variation in spore release (Fig. 5b).
Partial effects of (a) swell height (m), (b) wave period (s), (c) chlorophyll-a (mg m−3), and (d) rain (mm day−1) on the fitted values of the optimal spore-release model. Grey areas denote the 95% confidence interval and rugs (short stripes) on each individual graph represent observed spore-release data.
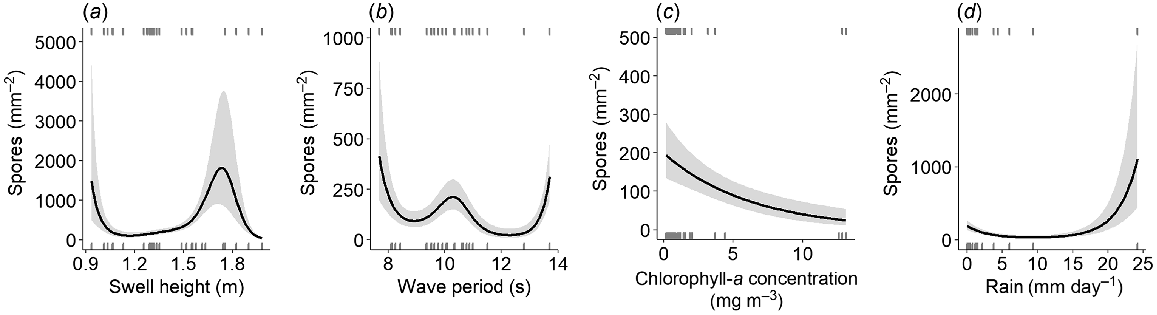
The smoothing function was dropped from chlorophyll-a in the optimal model in favour of a negative linear term (Fig. 6c), which was driven by higher values of chlorophyll-a concentration during July and September of the second sample year (Fig. S1). Rainfall generally did not have a large effect on spore release, but exceptionally high rainfall events (i.e. >25 mm day−1) affected spore release positively (Fig. 6d). This response was driven by fewer than five data points of high rainfall in December in both sample years (Fig. S1), which contributed to the peak of spore release noted in December (Fig. 5b).
Discussion
Populations at their warm range edge are often associated with variable reproductive phenology as an adaptation to environmental conditions that approach species’ physiological thresholds (Sexton et al. 2009; Duarte and Viejo 2018). Here, we showed that the fertility of E. radiata at its warm range edge off eastern Australia was highly variable and related to both seasonal and non-seasonal effects. The proportion of fertile plants was related to daylength, with shorter daylengths (Austral autumn and early winter) coinciding with higher proportions of fertile plants, which was consistent between sampling years. The length of fertile tissue on plants was only partly related to seasonally varying factors, with higher temperatures (Austral summer and autumn) contributing to more fertile tissue. However, episodic events such as intermediate swell events were associated with the greatest length of fertile tissue on thalli. Spores could be released from fertile plants year-round and the magnitude of spores released was influenced by episodic events including swells and rainfall. These specific fertility responses can be part of an adaptive reproductive response at the warm range edge and indicate a general versatility in phenology that may enhance species persistence at the range edge (Sexton et al. 2009).
The number of fertile E. radiata individuals varied seasonally and was not related to dynamic environmental factors. Seasonal patterns in growth and reproduction are well documented within kelps, where photoperiod or strict temperature thresholds can trigger a switch from vegetative growth to the production of reproductive tissue (Kain 1979; Lüning 1979; Lüning and tom Dieck 1989). Here, short daylengths coincided with a higher proportion of fertile plants, which occurred during Austral autumn and early winter. Seasonal influence was also detected in the length of fertile tissue, where higher sea-surface temperatures were related to increases in the length of fertile tissue. This indicated that Austral autumn (March–May) is the most fertile season for E. radiata at the warm range edge. During these months off eastern Australia, days start to shorten, yet the lag between atmospheric temperatures and SST means that the warmest ocean conditions are commonly experienced during this period (Fig. S1) (Whetton 1990), providing optimal conditions for a high proportion of the fertile plants and a high length of fertile tissue. These findings concur with those of other studies that have identified autumn as part of the peak of fertility within populations in Tasmania (Mabin et al. 2013; Tatsumi et al. 2022) and Western Australia (Mohring et al. 2013a), which may be related to daylengths between 10 and 12 h, identified in this study as correlating with peak fertility. Seasonal investment in reproduction is also recorded for other kelps, where set reproductive seasons unaffected by environmental change occur in Pterygophora californica (Reed et al. 1996) or narrow temperature windows drive the fertile season in Laminaria digitata (Bartsch et al. 2013). Variable and episodic environmental factors also affected the length of fertile tissue in E. radiata. For instance, higher temperatures in one sample year correlated with longer fertile tissue which may be related to increased growth during hotter summer months (Novaczek 1984a; Kain 1989; Wernberg et al. 2019b). Intermediate swells coincided with a higher length of fertile tissue, whereas higher swells were correlated with a decrease in the length of fertile tissue. This may potentially be due to periods of higher erosion and rates of sloughing old reproductive tissue during higher swells (Novaczek 1984a).
Whereas the length and proportion of fertile tissue might be highest in Austral autumn in each year, spore release was variable between sampling years. Continuous spore release was shown over both sample years, supporting the idea that reproduction can be more variable at the range edge. Minor peaks in spore release were related to episodic events such as higher swells and rainfall and periods after storms. Higher spore release in the first sample year coincided with higher swells. This indicates that these factors may promote spore release at the range edge of E. radiata’s distribution. Water motion has been shown to promote spore release in Alaria escuelenta (Gordon and Brawley 2004; McConnico and Foster 2005) and increased water motion can aid kelps with long-distance dispersal of spores (Graham 2003; Gaylord et al. 2006; de Bettignies et al. 2013), as may occur during storms. In addition, synchronised spore release can increase long-distance dispersal (Reed et al. 1997). The production of spores year-round also means that plants can readily respond to favourable environments (Reed et al. 1996). Year-round production of spores was also noted in Ecklonia maxima (Joska and Bolton 1987) at the pole-ward range edge and in Lessonia trabeculata (Tala et al. 2004) throughout the range, although the magnitude of spore release fluctuated temporally. Exposed areas were reproductive later in the year in L. trabeculata than were protected locations (Tala et al. 2004) and had a higher total spore output than did sheltered locations (Edding et al. 1993). This adaptation to exposure supports the notion that continuously reproducing plants can be adapted to locally favourable conditions. It should be noted that many of the significant predictors of spore release were complex predictors, and so making exact inferences is difficult. However, each variable predicting spore release was a stochastic event, indicating a dynamic response to environmental factors. Interestingly, the length of the fertile tissue was promoted by lower swells but spore release by higher swells in this study. This may indicate that plants grow and experience less erosion and produce more fertile tissue during calmer conditions, ready to release spores during periods of optimal high swell (de Bettignies et al. 2013).
Fertility in the form of spore release by E. radiata at the warm range edge was markedly different from that in populations at higher latitudes. Data on spore release are available for southern populations in Tasmania (i.e. the current, poleward cold range edge) and mid-latitude populations in Western Australia (i.e. a central population). First, the magnitude of peak spore release for E. radiata was ~50% less at the eastern Australian warm range edge (this study; maximum of 1440 spores mm−2 released) than in the central population off Western Australia (maximum of 2845 spores mm−2; Mohring et al. 2013a). Spore release in both of these locations represented only a small proportion of the maximum number of spores released at the current cold range edge in Tasmania (125 000 spores mm−2; Tatsumi et al. 2022). Second, spores were released year-round and at an approximately constant rate at the warm range edge in this study, whereas a defined season of spore release is apparent in Western Australia (summer and autumn; Mohring et al. 2013a; Fig. 7) and Tasmania (autumn and winter; Tatsumi et al. 2022) (Fig. 7). Third, the number of spores released per square millimetre was related to temperature only in Western Australia and Tasmania (Fig. 7), whereas there was no significant relationship between temperature and spore release at the eastern Australian warm range edge.
Comparison of spore-release data of three Ecklonia radiata populations in Australia. The warm range-edge population of this study shows a lower number of spores but is nearly constant throughout the year. The centre population of Western Australia shows seasonal spore release that peaks from 21 to 22.5°C and is positively correlated with temperature. The cold range edge has seasonal spore release that peaks at 14–16°C and is negatively correlated with temperature. The highest proportion of fertile plants is at 12.5 h of daylength at the warm range edge. Data were extracted from Tatsumi et al. (2022) for the Tasmanian populations and from Mohring et al. (2013a) for the Western Australian populations. Note the log10y-axis in the spore release graph.
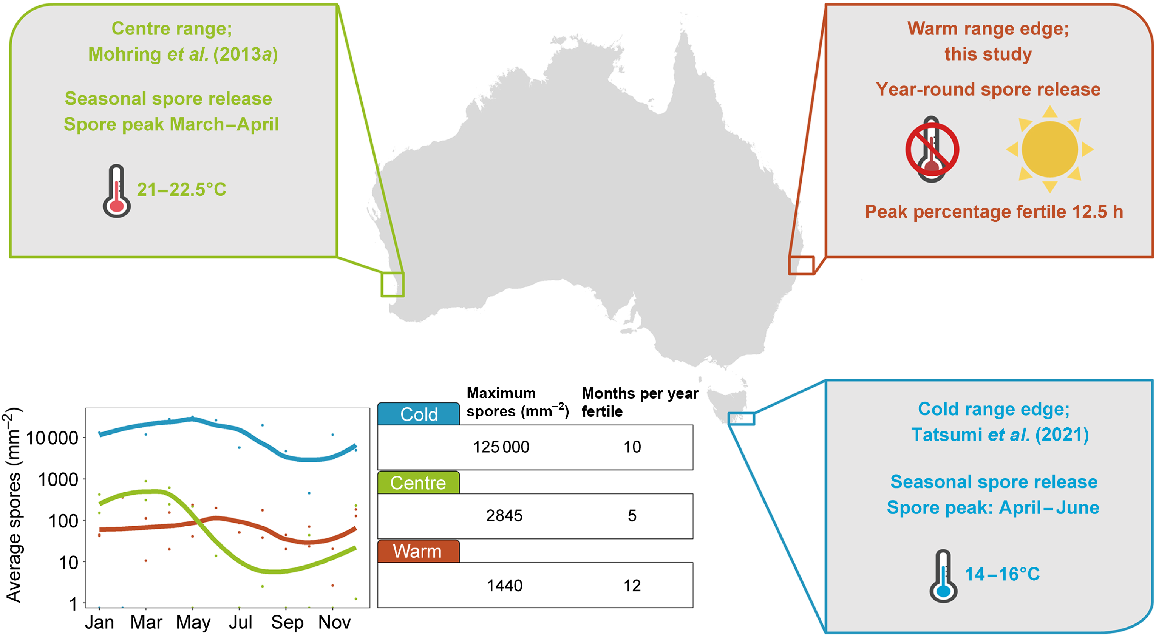
This may represent an altered reproductive strategy at the warm range edge where there is less distinct seasonality. The relatively lower number of spores released at the warm range edge supports the central-abundance theory (Gaston 2003; Sagarin et al. 2006); however, year-round fertility may compensate for lower numbers of spores released during peaks. The flexible response to environmental conditions may ensure that fertility matches favourable conditions for dispersal, whereas centre populations produce spores at set times of the year that may favour the survival of gametophytes (Mohring et al. 2013b). Because the production of spores appears to not incur large energetic costs for kelps (Joska and Bolton 1987; De Wreede and Klinger 1988; Santelices 1990), their fertility response may be driven by conditions favourable for gametophyte survival (Mohring et al. 2013b). Warm range-edge populations often produce gametophytes that are more thermally tolerant (Hollarsmith et al. 2020; Liesner et al. 2020), which is true for E. radiata (Mohring et al. 2014). The production of spores during warmer months may be beneficial for range edge populations, because these spores can utilise summer storms for increased dispersal and the thermally tolerant gametophytes may contribute to improved survival under higher temperatures. Conversely, narrower temperature thresholds for current cold range-edge gametophytes may limit spore production during warm months (Tatsumi et al. 2022). It should be noted that spores from Western Australia and this study were collected between 2- and 5-m depth, and spores from Tasmania at a depth of 11 m. Timing of spore release may be delayed at greater depths (~15 m), although this has not been found to influence spore density (Mohring et al. 2013a; Giraldo-Ospina et al. 2021). This may explain a slightly later spore-release peak in Tasmania than in Western Australia (Fig. 7).
The reproductive phenology of the range edge population of E. radiata off eastern Australia appears to be well adapted to current and predicted environmental conditions. The positive relationship between temperature and and the length of fertile tissue, and the increased spore release with storm events indicated that even under future climate change scenarios, the fertility of this population is unlikely to be restricted. Additionally, a positive correlation between temperature and spore release is found in Western Australian centre populations, but high temperatures decreased spore release in Tasmanian cold range-edge populations of E. radiata (Fig. 7). These responses indicated that poleward populations of E. radiata may be negatively affected by increasing temperatures (Tatsumi et al. 2022), contrary to populations elsewhere throughout the Australian distribution of E. radiata. This apparent adaptive fertility of E. radiata is in contrast with kelps from the northern hemisphere that grow in considerably cooler waters, where spore production and release are defined by a narrow temperature window, and so is more vulnerable to climate change (Bartsch et al. 2013). In Australia, it is more likely that populations will be limited by gametophyte reproduction and recruitment into young sporophytes, rather than spore release or survival of gametophytes. The temperature range and other environmental factors under which E. radiata gametophytes reproduce and recruit to young sporophytes in the field remain understudied (Wernberg et al. 2019b), although the temperature range is likely to be between 16 and 20°C in populations between ~43 and ~34°S (Novaczek 1984b; Mabin et al. 2013; Mohring et al. 2014; Alsuwaiyan et al. 2021) and in low-light conditions found under adult kelp canopies (Tatsumi and Wright 2016; Layton et al. 2020). This is a narrower temperature window than that in which the release of spores can occur (e.g. between 18 and 26°C in this study) and gametophytes can survive, which is reported to be up to 27°C (Mohring et al. 2014; Alsuwaiyan et al. 2021).
The results of this study can be used to identify the optimal timing to harvest fertile E. radiata plants for aquaculture or restoration. With a rising demand for sustainable food production, kelp aquaculture has received more attention in recent years (Grebe et al. 2019; Forbes et al. 2022). Traditional cultivation of kelp in Asian countries has led the way for research into other suitable kelp species for aquaculture (Grebe et al. 2019; Hu et al. 2021). E. radiata is not yet farmed on industrial scales (Wiltshire et al. 2015). This is partially due to the restricted knowledge we have of the microscopic life processes of E. radiata (Praeger et al. 2022). Our results indicated that with forecasts of temperature and daylength at weekly to monthly lead times, and accurate forecasts of wave activity up to 1 week ahead of time, the timing of optimal spore release and the presence of fertile tissue may be predictable at the warm range edge off eastern Australia, and certainly elsewhere where there were stronger links to temperature. More generally, Austral autumn appears to be the optimal season for collecting fertile plants throughout the Australian distribution of E. radiata. At the continental scale, seasonal peaks might be utilised to maximise spore release in central and cold range-edge populations (Fig. 7).
Here, we have filled knowledge gaps surrounding the phenology and fertility of a dominant but declining kelp, E. radiata. We showed that E. radiata has variable fertility depending on its geographical location. There was seasonality in the proportion of fertile plants, which increased with shortened daylengths. Strong seasonal spore release was noted in centre and cold range-edge populations, which might be connected to survival and recruitment of subsequent life-history phases. At the warm range edge off eastern Australia, spores can be released year-round but at lower rates than in the centre and cold range-edge populations. Episodic events such as storms and high swells were related to the magnitude of spore release at the range edge, which may benefit dispersal. The fertility patterns of E. radiata at the range edge both conform with (lowered spore counts) and deviate from (ability to reproduce year-round) the central-abundance theory. Apparent local adaptation indicates that sporophyte fertility is unlikely to be influenced by ocean warming, and the opportunistic use of environmental conditions favourable for dispersal may enhance spore release as the frequency and intensity of storms increase under climate change (Coumou and Rahmstorf 2012). Investigating reproductive phenology across temporal and spatial scales that encompass multiple populations exposed to variable environmental conditions can greatly improve the understanding of the plasticity of kelp. This plasticity can be vital to understanding the persistence of kelp under climate change and the contribution of kelp to the emerging blue economy.
Data availability
The data that support this study will be shared upon reasonable request to the corresponding author.
Declaration of funding
This work contributes to the NSW Primary Industries Climate Change Research Strategy, funded by the NSW Climate Change Fund. Funding was also provided by Australian Research Council grant DP190100058 awarded to M. A. Coleman. Funding was also provided by the Southern Cross University Higher Degree Research Scholarship. This work is supported by the Holsworth Wildlife Research Endowment, awarded to R. J. Veenhof.
Acknowledgements
Water-temperature data at Coffs Harbour were provided by NSW DPI Fisheries ‘shark research’ team from their tagged shark-listening station. We thank Manly Hydraulics Lab for the provision of their Wave Rider Buoy data. Wave data were collected under the NSW Coastal Data Network Program by Manly Hydraulics Laboratory, managed by Biodiversity and Conservation, Department of Planning, Industry and Environment. We thank M. De Brauwer and J. D. George for their assistance in the field. We thank two anonymous reviewers for their helpful feedback, which improved the paper greatly.
References
Abeli T, Gentili R, Mondoni A, Orsenigo S, Rossi G (2014) Effects of marginality on plant population performance. Journal of Biogeography 41(2), 239-249.
| Crossref | Google Scholar |
Almeida SC, Nicastro KR, Zardi GI, Pearson GA, Valero M, Serrão EA (2017) Reproductive strategies and population genetic structure of Fucus spp. across a Northeast Atlantic biogeographic transition. Aquatic Living Resources 30, 16.
| Crossref | Google Scholar |
Alsuwaiyan NA, Mohring MB, Cambridge M, Coleman MA, Kendrick GA, Wernberg T (2019) A review of protocols for the experimental release of kelp (Laminariales) zoospores. Ecology and Evolution 9(14), 8387-8398.
| Crossref | Google Scholar |
Alsuwaiyan NA, Vranken S, Filbee-Dexter K, Cambridge M, Coleman MA, Wernberg T (2021) Genotypic variation in response to extreme events may facilitate kelp adaptation under future climates. Marine Ecology Progress Series 672, 111-121.
| Crossref | Google Scholar |
Amsler CD, Neushul M (1989) Diel periodicity of spore release from the kelp Nereocystis luetkeana (Mertens) Postels et Ruprecht. Journal of Experimental Marine Biology and Ecology 134(2), 117-127.
| Crossref | Google Scholar |
Assis J, Berecibar E, Claro B, Alberto F, Reed D, Raimondi P, Serrão EA (2017) Major shifts at the range edge of marine forests: the combined effects of climate changes and limited dispersal. Scientific Reports 7(1), 44348.
| Crossref | Google Scholar |
Bartsch I, Vogt J, Pehlke C, Hanelt D (2013) Prevailing sea surface temperatures inhibit summer reproduction of the kelp Laminaria digitata at Helgoland (North Sea). Journal of Phycology 49(6), 1061-1073.
| Crossref | Google Scholar |
Brown CJ, O’Connor MI, Poloczanska ES, Schoeman DS, Buckley LB, Burrows MT, Duarte CM, Halpern BS, Pandolfi JM, Parmesan C, Richardson AJ (2016) Ecological and methodological drivers of species’ distribution and phenology responses to climate change. Global Change Biology 22(4), 1548-1560.
| Crossref | Google Scholar |
Buschmann AH, Vásquez JA, Osorio P, Reyes E, Filún L, Hernández-González MC, Vega A (2004) The effect of water movement, temperature and salinity on abundance and reproductive patterns of Macrocystis spp. (Phaeophyta) at different latitudes in Chile. Marine Biology 145(5), 849-862.
| Crossref | Google Scholar |
Buschmann AH, Moreno C, Vásquez JA, Hernández-González MC (2006) Reproduction strategies of Macrocystis pyrifera (Phaeophyta) in southern Chile: the importance of population dynamics. Journal of Applied Phycology 18(3), 575-582.
| Crossref | Google Scholar |
Camus C, Solas M, Martínez C, Vargas J, Garcés C, Gil-Kodaka P, Ladah LB, Serrão EA, Faugeron S (2021) Mates matter: gametophyte kinship recognition and inbreeding in the giant kelp, Macrocystis pyrifera (Laminariales, Phaeophyceae). Journal of Phycology 57(3), 711-725.
| Crossref | Google Scholar |
Carnell PE, Keough MJ (2019) Reconstructing historical marine populations reveals major decline of a kelp forest ecosystem in Australia. Estuaries and Coasts 42(3), 765-778.
| Crossref | Google Scholar |
Coleman MA, Reddy M, Nimbs MJ, Marshell A, Al-Ghassani SA, Bolton JJ, Jupp BP, De Clerck O, Leliaert F, Champion C, Pearson GA, Serrão EA, Madeira P, Wernberg T (2022) Loss of a globally unique kelp forest from Oman. Scientific Reports 12(1), 5020.
| Crossref | Google Scholar |
Coumou D, Rahmstorf S (2012) A decade of weather extremes. Nature Climate Change 2(7), 491-496.
| Crossref | Google Scholar |
Dangremond EM, Hill CH, Louaibi S, Muñoz I (2022) Phenological responsiveness and fecundity decline near the southern range limit of Trientalis borealis (Primulaceae). Plant Ecology 223(1), 41-52.
| Crossref | Google Scholar |
Davis TR, Champion C, Coleman MA (2021) Climate refugia for kelp within an ocean warming hotspot revealed by stacked species distribution modelling. Marine Environmental Research 166, 105267.
| Crossref | Google Scholar |
Davis TR, Champion C, Coleman MA (2022) Ecological interactions mediate projected loss of kelp biomass under climate change. Diversity and Distributions 28(2), 306-317.
| Crossref | Google Scholar |
Dayton PK (1985) Ecology of kelp communities. Annual Review of Ecology and Systematics 16(1), 215-245.
| Crossref | Google Scholar |
de Bettignies T, Wernberg T, Lavery PS, Vanderklift MA, Mohring MB (2013) Contrasting mechanisms of dislodgement and erosion contribute to production of kelp detritus. Limnology and Oceanography 58(5), 1680-1688.
| Crossref | Google Scholar |
Duarte L, Viejo RM (2018) Environmental and phenotypic heterogeneity of populations at the trailing range-edge of the habitat-forming macroalga Fucus serratus. Marine Environmental Research 136, 16-26.
| Crossref | Google Scholar |
Edding ME, Fonck E, Orrego P, Venegas M, Macchiavello J (1993) A comparison between two populations of Lessonia trabeculata (Phaeophyta: Laminariales) microscopic stages. Hydrobiologia 260, 231-237.
| Crossref | Google Scholar |
Edwards MS (2022) It’s the little things: the role of microscopic life stages in maintaining kelp populations. Frontiers in Marine Science 9, 871204.
| Crossref | Google Scholar |
Filbee-Dexter K, Wernberg T, Grace SP, Thormar J, Fredriksen S, Narvaez CN, Feehan CJ, Norderhaug KM (2020) Marine heatwaves and the collapse of marginal North Atlantic kelp forests. Scientific Reports 10(1), 13388.
| Crossref | Google Scholar |
Forbes H, Shelamoff V, Visch W, Layton C (2022) Farms and forests: evaluating the biodiversity benefits of kelp aquaculture. Journal of Applied Phycology 34, 3059-3067.
| Crossref | Google Scholar |
Gaylord B, Reed DC, Raimondi PT, Washburn L (2006) Macroalgal spore dispersal in coastal environments: mechanistic insights revealed by theory and experiment. Ecological Monographs 76(4), 481-502.
| Crossref | Google Scholar |
Giraldo-Ospina A, Kendrick GA, Hovey RK (2021) Reproductive output, synchrony across depth and influence of source depth in the development of early life stages of kelp. Journal of Phycology 57, 311-323.
| Crossref | Google Scholar |
Gordon R, Brawley SH (2004) Effects of water motion on propagule release from algae with complex life histories. Marine Biology 145(1), 21-29.
| Crossref | Google Scholar |
Graham MH (2003) Coupling propagule output to supply at the edge and interior of a giant kelp forest. Ecology 84(5), 1250-1264.
| Crossref | Google Scholar |
Granado-Yela C, Balaguer L, García-Verdugo C, Carrillo K, Méndez M (2013) Thriving at the limit: differential reproductive performance in range-edge populations of a Mediterranean sclerophyll (Olea europaea). Acta Oecologica 52, 29-37.
| Crossref | Google Scholar |
Grebe GS, Byron CJ, Gelais AS, Kotowicz DM, Olson TK (2019) An ecosystem approach to kelp aquaculture in the Americas and Europe. Aquaculture Reports 15, 100215.
| Crossref | Google Scholar |
Habibzadeh N, Ghoddousi A, Bleyhl B, Kuemmerle T (2021) Rear-edge populations are important for understanding climate change risk and adaptation potential of threatened species. Conservation Science and Practice 3(5), e375.
| Crossref | Google Scholar |
Hampe A, Petit RJ (2005) Conserving biodiversity under climate change: the rear edge matters. Ecology Letters 8(5), 461-467.
| Crossref | Google Scholar |
Hargreaves AL, Eckert CG (2014) Evolution of dispersal and mating systems along geographic gradients: implications for shifting ranges. Functional Ecology 28(1), 5-21.
| Crossref | Google Scholar |
Hargreaves AL, Eckert CG (2019) Local adaptation primes cold-edge populations for range expansion but not warming-induced range shifts. Ecology Letters 22(1), 78-88.
| Crossref | Google Scholar |
Hobday AJ, Lough JM (2011) Projected climate change in Australian marine and freshwater environments. Marine and Freshwater Research 62(9), 1000-1014.
| Crossref | Google Scholar |
Hoffmann AJ (1987) The arrival of seaweed propagules at the shore: a review. Botanica Marina 30(2), 151-166.
| Crossref | Google Scholar |
Hollarsmith JA, Buschmann AH, Camus C, Grosholz ED (2020) Varying reproductive success under ocean warming and acidification across giant kelp (Macrocystis pyrifera) populations. Journal of Experimental Marine Biology and Ecology 522, 151247.
| Crossref | Google Scholar |
Hu Z-M, Shan T-F, Zhang J, Zhang Q-S, Critchley AT, Choi H-G, Yotsukura N, Liu F-L, Duan D-L (2021) Kelp aquaculture in China: a retrospective and future prospects. Reviews in Aquaculture 13(3), 1324-1351.
| Crossref | Google Scholar |
Johnson CR, Banks SC, Barrett NS, Cazassus F, Dunstan PK, Edgar GJ, Frusher SD, Gardner C, Haddon M, Helidoniotis F, Hill KL, Holbrook NJ, Hosie GW, Last PR, Ling SD, Melbourne-Thomas J, Miller K, Pecl GT, Richardson AJ, Ridgway KR, Rintoul SR, Ritz DA, Ross DJ, Sanderson JC, Shepherd SA, Slotwinski A, Swadling KM, Taw N (2011) Climate change cascades: shifts in oceanography, species’ ranges and subtidal marine community dynamics in eastern Tasmania. Journal of Experimental Marine Biology and Ecology 400(1–2), 17-32.
| Crossref | Google Scholar |
Joska MAP, Bolton JJ (1987) In situ measurement of zoospore release and seasonality of reproduction in Ecklonia maxima (Alariaceae, Laminariales). British Phycological Journal 22(2), 209-214.
| Crossref | Google Scholar |
Kain JM (1979) A view of the genus Laminaria. Oceanography and Marine Biology: an Annual Review 17, 101-161.
| Google Scholar |
Kain JM (1989) The seasons in the subtidal. British Phycological Journal 24(3), 203-215.
| Crossref | Google Scholar |
Kawecki TJ (2008) Adaptation to marginal habitats. Annual Review of Ecology, Evolution, and Systematics 39, 321-342.
| Crossref | Google Scholar |
King NG, McKeown NJ, Smale DA, Wilcockson DC, Hoelters L, Groves EA, Stamp T, Moore PJ (2019) Evidence for different thermal ecotypes in range centre and trailing edge kelp populations. Journal of Experimental Marine Biology and Ecology 514–515, 10-17.
| Crossref | Google Scholar |
Lara-Romero C, García-Camacho R, Escudero A, Iriondo JM (2014) Genetic variation in flowering phenology and reproductive performance in a Mediterranean high-mountain specialist, Armeria caespitosa (Plumbaginaceae). Botanical Journal of the Linnean Society 176(3), 384-395.
| Crossref | Google Scholar |
Layton C, Cameron MJ, Tatsumi M, Shelamoff V, Wright JT, Johnson CR (2020) Habitat fragmentation causes collapse of kelp recruitment. Marine Ecology Progress Series 648, 111-123.
| Crossref | Google Scholar |
Lester SE, Gaines SD, Kinlan BP (2007) Reproduction on the edge: large-scale patterns of individual performance in a marine invertebrate. Ecology 88(9), 2229-2239.
| Crossref | Google Scholar |
Levin DA (2012) Mating system shifts on the trailing edge. Annals of Botany 109(3), 613-620.
| Crossref | Google Scholar |
Liesner D, Fouqueau L, Valero M, Roleda MY, Pearson GA, Bischof K, Valentin K, Bartsch I (2020) Heat stress responses and population genetics of the kelp Laminaria digitata (Phaeophyceae) across latitudes reveal differentiation among North Atlantic populations. Ecology and Evolution 10(17), 9144-9177.
| Crossref | Google Scholar |
Liu X, Bogaert K, Engelen AH, Leliaert F, Roleda MY, De Clerck O (2017) Seaweed reproductive biology: environmental and genetic controls. Botanica Marina 60(2), 89-108.
| Crossref | Google Scholar |
Lüning K (1979) Growth strategies of three Laminaria species (Phaeophyceae) inhabiting different depth zones in the sublittoral region of Helgoland (North Sea). Marine Ecology Progress Series 1(3), 195-207.
| Google Scholar |
Lüning K, tom Dieck I (1989) Environmental triggers in algal seasonality. Botanica Marina 32(5), 389-398.
| Crossref | Google Scholar |
Mabin CJT, Gribben PE, Fischer A, Wright JT (2013) Variation in the morphology, reproduction and development of the habitat-forming kelp Ecklonia radiata with changing temperature and nutrients. Marine Ecology Progress Series 483, 117-131.
| Crossref | Google Scholar |
McConnico LA, Foster MS (2005) Population biology of the intertidal kelp, Alaria marginata Postels and Ruprecht: a non-fugitive annual. Journal of Experimental Marine Biology and Ecology 324(1), 61-75.
| Crossref | Google Scholar |
Mohring MB, Wernberg T, Kendrick GA, Rule MJ (2013a) Reproductive synchrony in a habitat-forming kelp and its relationship with environmental conditions. Marine Biology 160(1), 119-126.
| Crossref | Google Scholar |
Mohring MB, Kendrick GA, Wernberg T, Rule MJ, Vanderklift MA (2013b) Environmental influences on kelp performance across the reproductive period: an ecological trade-off between gametophyte survival and growth? PLoS ONE 8(6), e65310.
| Crossref | Google Scholar |
Mohring MB, Wernberg T, Wright JT, Connell SD, Russell BD (2014) Biogeographic variation in temperature drives performance of kelp gametophytes during warming. Marine Ecology Progress Series 513, 85-96.
| Crossref | Google Scholar |
Muth AF, Graham MH, Lane CE, Harley CDG (2019) Recruitment tolerance to increased temperature present across multiple kelp clades. Ecology 100(3), e02594.
| Crossref | Google Scholar |
Nimbs MJ, Wernberg T, Davis TR, Champion C, Coleman MA (2023) Climate change threatens unique evolutionary diversity in Australian kelp refugia. Scientific Reports 13(1), 1248.
| Crossref | Google Scholar |
Novaczek I (1984a) Development and phenology of Ecklonia radiata at two depths in Goat Island Bay, New Zealand. Marine Biology 81(2), 189-197.
| Crossref | Google Scholar |
Novaczek I (1984b) Response of gametophytes of Ecklonia radiata (Laminariales) to temperature in saturating light. Marine Biology 82(3), 241-245.
| Crossref | Google Scholar |
Oppliger LV, von Dassow P, Bouchemousse S, Robuchon M, Valero M, Correa JA, Mauger S, Destombe C (2014) Alteration of sexual reproduction and genetic diversity in the kelp species Laminaria digitata at the southern limit of its range. PLoS ONE 9(7), e102518.
| Crossref | Google Scholar |
Parmesan C, Hanley ME (2015) Plants and climate change: complexities and surprises. Annals of Botany 116(6), 849-864.
| Crossref | Google Scholar |
Pironon S, Papuga G, Villellas J, Angert AL, García MB, Thompson JD (2017) Geographic variation in genetic and demographic performance: new insights from an old biogeographical paradigm. Biological Reviews 92(4), 1877-1909.
| Crossref | Google Scholar |
Praeger C, Magnusson M, Lawton R (2022) Optimising the zoospore release, germination, development of gametophytes and formation of sporophytes of Ecklonia radiata. Journal of Applied Phycology 34, 2535-2549.
| Crossref | Google Scholar |
Reed DC, Ebeling AW, Anderson TW, Anghera M (1996) Differential reproductive responses to fluctuating resources in two seaweeds with different reproductive strategies. Ecology 77(1), 300-316.
| Crossref | Google Scholar |
Reed DC, Anderson TW, Ebeling AW, Anghera M (1997) The role of reproductive synchrony in the colonization potential of kelp. Ecology 78(8), 2443-2457.
| Crossref | Google Scholar |
Rehm EM, Olivas P, Stroud J, Feeley KJ (2015) Losing your edge: climate change and the conservation value of range-edge populations. Ecology and Evolution 5(19), 4315-4326.
| Crossref | Google Scholar |
Robuchon M, Le Gall L, Mauger S, Valero M (2014) Contrasting genetic diversity patterns in two sister kelp species co-distributed along the coast of Brittany, France. Molecular Ecology 23(11), 2669-2685.
| Crossref | Google Scholar |
Sagarin RD, Gaines SD, Gaylord B (2006) Moving beyond assumptions to understand abundance distributions across the ranges of species. Trends in Ecology & Evolution 21(9), 524-530.
| Crossref | Google Scholar |
Santelices B (1990) Patterns of reproduction, dispersal and recruitment in seaweeds. Oceanography and Marine Biology: An Annual Review 28, 177-276.
| Google Scholar |
Sexton JP, McIntyre PJ, Angert AL, Rice KJ (2009) Evolution and ecology of species range limits. Annual Review of Ecology, Evolution, and Systematics 40(1), 415-436.
| Crossref | Google Scholar |
Shay JE, Pennington LK, Mandussi Montiel-Molina JA, Toews DJ, Hendrickson BT, Sexton JP (2021) Rules of plant species ranges: applications for conservation strategies. Frontiers in Ecology and Evolution 9, 700962.
| Crossref | Google Scholar |
Sinclair EA, Edgeloe JM, Anthony JM, Statton J, Breed MF, Kendrick GA (2020) Variation in reproductive effort, genetic diversity and mating systems across Posidonia australis seagrass meadows in Western Australia. AoB PLANTS 12(4), plaa038.
| Crossref | Google Scholar |
Tala F, Edding M, Vasquez J (2004) Aspects of the reproductive phenology of Lessonia trabeculata (Laminariales: Phaeophyceae) from three populations in northern Chile. New Zealand Journal of Marine and Freshwater Research 38(2), 255-266.
| Crossref | Google Scholar |
Tatsumi M, Wright JT (2016) Understory algae and low light reduce recruitment of the habitat-forming kelp Ecklonia radiata. Marine Ecology Progress Series 552, 131-143.
| Crossref | Google Scholar |
Tatsumi M, Mabin CJT, Layton C, Shelamoff V, Cameron MJ, Johnson CR, Wright JT (2022) Density-dependence and seasonal variation in reproductive output and sporophyte production in the kelp, Ecklonia radiata. Journal of Phycology 58, 92-104.
| Crossref | Google Scholar |
tom Dieck I (1991) Circannual growth rhythm and photoperiodic sorus induction in the kelp Laminaria setchellii (Phaeophyta). Journal of Phycology 27(3), 341-350.
| Crossref | Google Scholar |
Vergés A, Doropoulos C, Malcolm HA, Skye M, Garcia-Pizá M, Marzinelli EM, Campbell AH, Ballesteros E, Hoey AS, Vila-Concejo A, Bozec Y-M, Steinberg PD (2016) Long-term empirical evidence of ocean warming leading to tropicalization of fish communities, increased herbivory, and loss of kelp. Proceedings of the National Academy of Sciences 113(48), 13791-13796.
| Crossref | Google Scholar |
Vranken S, Wernberg T, Scheben A, Severn-Ellis AA, Batley J, Bayer PE, Edwards D, Wheeler D, Coleman MA (2021) Genotype–environment mismatch of kelp forests under climate change. Molecular Ecology 30(15), 3730-3746.
| Crossref | Google Scholar |
Wernberg T, Smale DA, Tuya F, Thomsen MS, Langlois TJ, de Bettignies T, Bennett S, Rousseaux CS (2013) An extreme climatic event alters marine ecosystem structure in a global biodiversity hotspot. Nature Climate Change 3(1), 78-82.
| Crossref | Google Scholar |
Wernberg T, Bennett S, Babcock RC, de Bettignies T, Cure K, Depczynski M, Dufois F, Fromont J, Fulton CJ, Hovey RK, Harvey ES, Holmes TH, Kendrick GA, Radford B, Santana-Garcon J, Saunders BJ, Smale DA, Thomsen MS, Tuckett CA, Tuya F, Vanderklift MA, Wilson S (2016) Climate-driven regime shift of a temperate marine ecosystem. Science 353(6295), 169-172.
| Crossref | Google Scholar |
Wernberg T, Coleman MA, Babcock RC, Bell SY, Bolton JJ, Connell SD, Hurd CL, Johnson CR, Marzinelli EM, Shears NT, Steinberg PD, Thomsen MS, Vanderklift MA, Vergés A, Wright JT (2019b) Biology and ecology of the globally significant kelp Ecklonia radiata. Oceanography and Marine Biology: An Annual Review 57, 265-324.
| Google Scholar |
Whetton PH (1990) Relationships between monthly anomalies of sea-surface temperature and mean sea level pressure in the Australian region. Australian Meteorological Magazine 38(1), 17-30.
| Google Scholar |
Wijffels SE, Beggs H, Griffin C, Middleton JF, Cahill M, King E, Jones E, Feng M, Benthuysen JA, Steinberg CR, Sutton P (2018) A fine spatial-scale sea surface temperature atlas of the Australian regional seas (SSTAARS): seasonal variability and trends around Australasia and New Zealand revisited. Journal of Marine Systems 187, 156-196.
| Crossref | Google Scholar |
Wood SN, Pya N, Säfken B (2016) Smoothing parameter and model selection for general smooth models. Journal of the American Statistical Association 111(516), 1548-1563.
| Crossref | Google Scholar |
Zardi GI, Nicastro KR, Serrão EA, Jacinto R, Monteiro CA, Pearson GA (2015) Closer to the rear edge: ecology and genetic diversity down the core-edge gradient of a marine macroalga. Ecosphere 6(2), 1-25.
| Crossref | Google Scholar |
Zeileis A, Kleiber C, Jackman S (2008) Regression models for count data in R. Journal of Statistical Software 27(8), 1-25.
| Crossref | Google Scholar |