Effects of common carp on water quality and submerged vegetation: results from a short-term mesocosm experiment in an artificial wetland
Douglas Peterson
A US Fish and Wildlife Service, Abernathy Fish Technology Center, 1440 Abernathy Creek Road, Longview, WA 98632, USA.
B US Fish and Wildlife Service, Malheur National Wildlife Refuge, 36391 Sodhouse Lane, Princeton, OR 97721, USA.
C US Fish and Wildlife Service, Columbia River Fish and Wildlife Conservation Office, 1211 SE Cardinal Court, Suite 100, Vancouver, WA 98683, USA.
D Present address: East Bay Municipal Utility District, 1 Winemaster Way, Lodi, CA 95240, USA.
Marine and Freshwater Research 73(7) 973-994 https://doi.org/10.1071/MF22008
Submitted: 4 December 2021 Accepted: 24 March 2022 Published: 30 May 2022
© 2022 The Author(s) (or their employer(s)). Published by CSIRO Publishing. This is an open access article distributed under the Creative Commons Attribution-NonCommercial-NoDerivatives 4.0 International License (CC BY-NC-ND)
Abstract
Bioturbation by non-native common carp (carp) can facilitate an ecosystem phase shift from clear to turbid water in shallow lakes and ponds, with negative effects on abundance of aquatic animals and plants. Management of carp often involves reducing populations below a threshold biomass at which important components of the aquatic ecosystem, like submerged aquatic vegetation (SAV), recover. To evaluate control targets for a nuisance carp population in Malheur Lake in SE Oregon, USA, we conducted a mesocosm experiment in a small wetland within the lake’s drainage basin. We stocked 200-m2 enclosures with individual or multiple carp of different age classes at target biomasses of 50, 100, and 300 kg ha−1. We measured turbidity, total suspended sediments, nutrients, chlorophyll-a, and surface coverage of SAV four times within a single growing season. Turbidity increased through time and soluble P was higher in 300 kg ha−1 carp treatments compared to the control, but few changes were observed for any of the other variables at any biomass. Results suggest that with well established SAV, a maintenance carp biomass threshold of <200 kg ha−1 will not result in a phase shift from a clear- to turbid-water state in the short-term.
Keywords: alternative stable states, bioturbation, Cyprinus carpio, mesocosm, N, non-native, P, UAS imagery, water clarity.
Introduction
Shallow lakes and ponds can exist in clear or turbid stable states that are driven and maintained by reinforcing feedback loops (alternative stable states theory; Scheffer et al. 1993; Williams 2005; Janssen et al. 2014). The clear state is characterised by an abundance of aquatic macrophytes, diverse aquatic biota, low water column nutrients and phytoplankton biomass, whereas the turbid state is characterised by the opposite (Scheffer et al. 1993; Hargeby et al. 2004; Janssen et al. 2014). A shift from a clear to a turbid state can be induced by several physical or ecological factors, and interactions among them, including climatic drivers, nutrient fluxes, hydrologic variability, and biotic invaders (Scheffer et al. 1993; Güneralp and Barlas 2003; Scheffer and Carpenter 2003). Biotic invaders, in particular, have been shown to facilitate shifts in water clarity in shallow wetland and lake ecosystems, with cascading effects on ecosystem services (Scheffer et al. 1993; Crooks 2002; Kaemingk et al. 2017). One such biotic invader frequently implicated in the shift of shallow lakes and ponds are Eurasian common carp (Cyprinus carpio; hereafter ‘carp’).
Carp are the eighth most prevalent non-native invader in the world (Lowe et al. 2000), with an extensive introduced range, where they achieve high population densities (>1000 kg ha−1), and have devastated aquatic ecosystems on multiple continents (e.g. Bajer et al. 2009; Weber and Brown 2009; Vilizzi et al. 2015). Carp are considered ‘ecosystem engineers’ (sensu Jones et al. 1994) because they can alter the structure and function of aquatic systems through middle-out processes (Kaemingk et al. 2017), which is related to their mode of feeding (benthic foraging) where they physically uproot aquatic vegetation and simultaneously suspend sediment, while also excreting nutrients into the water column (reviewed by Weber and Brown 2009). These factors increase turbidity by (1) increased suspended sediment concentrations and (2) increased water column nutrients, which promote the growth of algae (Weber and Brown 2009; Badiou and Goldsborough 2010, 2015), further diminishing light penetration, and in turn inhibiting the recruitment and growth of aquatic vegetation (Weber and Brown 2009). These processes initiate reinforcing feedback loops implicated in the state transition from clear to turbid water (e.g. Scheffer et al. 1993; Vilizzi et al. 2014), which can lead to changes in the community composition and abundance of aquatic vegetation (Bajer et al. 2009; Kaemingk et al. 2017), benthic invertebrates (Parkos et al. 2003), fishes (Kopf et al. 2019; Marshall et al. 2019), and water birds (Bajer et al. 2009; Maceda-Veiga et al. 2017).
Effects of carp appear to follow a density–impact relationship. For example, Bajer et al. (2009) demonstrated in a shallow lake in the Midwestern USA that vegetative cover and duck abundance decreased dramatically after carp density increased above 100 kg ha−1. Studies in other locations have reported substantially higher biomass levels of carp before negative effects were apparent (e.g. Fletcher et al. 1985; Drenner et al. 1997; Lougheed et al. 1998), which suggests threshold values may be site-specific and context dependent. Vilizzi et al. (2015) conducted a meta-analysis on the effects of carp and observed considerable variation in results among study methods and geography. Analyses indicated average threshold densities for carp at which negative effects were observed were dependent on whether the response variable was abiotic (e.g. water clarity) or biotic (e.g. abundance of organisms), and for organisms it can vary by taxon (Vilizzi et al. 2015). From a management context, these results indicate that carp populations could potentially be suppressed to a level of functional extinction (sensu Green and Grosholz 2021) such that improved water quality promotes the recovery of desired plant and wildlife populations.
A variety of methods have been used to control nuisance carp populations (Rytwinski et al. 2019), including active and passive fishery suppression (traps, netting, electrofishing; Bajer et al. 2011; Stuart and Conallin 2018), overfishing by commercial harvest (Koehn et al. 2000), fish toxicants (Huser et al. 2022), and by blocking access to spawning habitat (Shields 1958; Hillyard et al. 2010; Taylor et al. 2012). However, carp are very difficult to eradicate from large waterbodies due to density dependent responses to suppression and their ability to survive in refugia habitat (Brown and Walker 2004; Weber and Brown 2009; Bajer and Sorensen 2010).
The effect of carp may differ by size or life stage (e.g. Driver et al. 2005). For example, carp shift from pelagic feeding on zooplankton to benthic feeding on macroinvertebrates as they grow (e.g. diet shift at >100-mm length), therefore the effect of bioturbation of sediment can change with size structure of the population (e.g. Kloskowski 2011). Excretion has been cited as one mechanism for nutrient enrichment by carp, and smaller fish have higher per-capita excretion rates than larger fish (e.g. Morgan and Hicks 2013). Environmental variation can lead to shifts in age and size structure of a carp population (Maiztegui et al. 2019). For instance, modelling has demonstrated that carp populations can exhibit boom or bust reproduction related to water level fluctuations, such that persistent drought can skew the population towards large adults (Pearson et al. 2022). Fisheries management can also influence population structure, as some capture methods are size selective (e.g. Weber et al. 2011). Control efforts targeting adults may result in unintended responses in the carp population dynamics due to compensatory responses (i.e. increased recruitment and juvenile survival; Pine et al. 2009; Weber et al. 2016).
Malheur Lake is a large, shallow, endorheic lake located within the Malheur National Wildlife Refuge (Malheur NWR), and provides critical habitat for migratory waterfowl (e.g. Pacific Flyway) as well as resident birds and fishes (Duebbert 1969; Cornely 1982). Common carp were first noted in Malheur Lake in the 1950s with subsequent losses of submerged aquatic vegetation, reduced populations of waterfowl, and more generally a shift in the stable state from a clear- to turbid-water state (e.g. Scheffer et al. 1993; Ivey et al. 1998). Controlling the carp population in Malheur Lake is a key part of the overall management of Malheur NWR (US Fish and Wildlife Service 2013). The biomass for carp at which ecological processes or ecosystem components would start to recover in Malheur Lake is unknown. Therefore, we conducted a manipulative field experiment in a small wetland within Malheur NWR to identify a carp biomass threshold applicable to the physiographic context of Malheur Lake. We constructed enclosures (mesocosms) in a wetland where carp were absent and submerged aquatic vegetation (SAV) was already established. Enclosures were stocked with individual carp or multiple age classes of carp at three biomass levels. We measured water clarity, suspended sediments, chlorophyll-a, nutrients, and SAV in treatment enclosures, control enclosures (no carp) and unenclosed reference plots during a single growing season (June–October) in 2019.
Methods
Study area, sample plot collection, and enclosures
Windmill Pond is an ∼3.2-ha human-made waterbody located within the western portion of Malheur NWR in south-eastern Oregon, USA (Fig. 1). The pond is in the floodplain of Silver Creek, a tributary to Harney Lake, but is physically isolated except in years with flooding by overland flow. Water level in Windmill Pond is maintained by pumping water from a well (∼1 km away) through an underground pipe that discharges into the south-west edge of the pond. The pond was selected for the study because it was small, fishless, and the water level could be controlled, to some extent. Windmill Pond was dry as of 2014, re-watering began in spring 2015, and by summer 2016 aquatic macrophytes had begun to reestablish.
Windmill Pond was divided into three blocks based on position relative to the pond’s water source and an exploratory principal components analysis of specific conductivity, water temperature, turbidity and chlorophyll-a concentration collected using a sonde (YSI model 6920, Yellow Springs, OH, USA1). Enclosure sites were randomly selected within blocks (see the ‘Additional details for methods’ section in the Supplementary material). In 2017, the 21 walled enclosures (0.02 ha, 14.1 m per side) were constructed of nylon reinforced plastic sheeting with the 1.5 m tall sides supported by metal fenceposts and fastened by plastic cable ties. The bottom edge was buried in the substrate and secured with earth anchors and sandbags (see the ‘Additional details for methods’ section in the Supplementary material for additional details). In 2018, we discovered that flooding the previous spring had permitted common carp to invade Windmill Pond. The invading carp and their progeny were eradicated with piscicide in July 2018, and the study was delayed until 2019 to permit recovery of any invertebrate fauna affected by the piscicide treatment. During 19–20 June 2019, enclosures were inspected for damage and gaps, and the sides were raised above the water line and secured.
Biomass treatments and fish stocking
We tested carp biomass treatments of 50, 100, and 300 kg ha−1, and each biomass treatment was stocked with either a single or multiple fish. The 100 kg ha−1 treatment was based on Bajer et al. (2009), which had been incorporated as an interim management target in Malheur Lake under Malheur NWR’s Comprehensive Conservation Plan (US Fish and Wildlife Service 2013). The 50 kg ha−1 treatment was based on a threshold for effects on waterfowl from a meta-analysis of studies using exclosures and natural experiments (Vilizzi et al. 2015). The 300 kg ha−1 treatment would be expected to cause significant changes to many components of the ecosystem (water quality, vegetation, invertebrates, and vertebrates; Vilizzi et al. 2015) and is similar to estimated and modelled biomass of carp in Malheur Lake (e.g. Pearson et al. 2019). At each biomass, we used two stocking treatments consisting of: (1) three to four carp of multiple age classes (age-1+; representing a population with annual reproduction) or (2) a single large carp (representing a population where recruitment may be periodic due to abiotic factors). Both scenarios have been observed in the endorheic Malheur Lake, where fluctuations in lake area, both seasonally and annually (e.g. minimum ∼550 ha in 1992, maximum ∼54 000 ha in 1986), are driven by winter precipitation and snowmelt runoff (Hubbard 1975; Pearson et al. 2022). Each of the three blocks had eight sample units: an open reference plot lacking enclosure walls, a zero biomass enclosure, and six enclosures with carp (three biomass treatments × two size/age structures). Carp and zero biomass treatments were randomly assigned within blocks.
Carp were captured in Malheur Lake and Moon Reservoir (dammed portion of Silver Creek), moved to the study site in a 1136-L aerated fish transport tank installed on a truck, and held temporarily in 660-L circular tanks staged adjacent to the pond. Study fish were sedated with MS-222, measured (total length, TL, to the nearest millimetre), weighed (to the nearest gram), and then double tagged with a 12-mm full-duplex PIT tag implanted into the dorsal sinus cavity using a syringe and a 23-mm half-duplex PIT tag implanted into the body cavity through a small incision. Tagged study fish were stocked during 28–29 June 2019. At the end of the study (29 September−2 October 2019), we used a pair of custom-made mobile PIT tag antenna systems (PIT-packs, sensu Hill et al. 2006) to locate carp within enclosures, and then used a pair of backpack electrofishers operated at 175–250 V and 30–40 Hz (LR-24, Smith-Root, Vancouver, WA, USA) to immobilise fish for netting. Recaptured carp were measured, weighed, and then euthanased.
Nutrients and water quality
Field data collection
We monitored a suite of water quality parameters in enclosures and reference plots during four monthly sampling events: once in June (24–26 June) immediately before stocking carp in enclosures, and three times after stocking (29–31 July, 3–5 September, and 24–26 September). A 1-L plastic sample pitcher connected to a telescoping fibreglass pole was used to collect a water sample at ∼40% of the water depth in the centre of each of four equal-area quadrants within each enclosure or reference plot (see the ‘Additional details for methods’ section in the Supplementary material for additional details). Each water sample was poured into a clean, pre-rinsed 1-L Nalgene collection bottle and placed on ice inside a cooler. Concurrently, a sonde (YSI model 6920) was used to measure instantaneous water temperature (0.01°C) at 40% depth, and a telescoping measuring rod was used to measure water depth (nearest cm) at each sample location.
Laboratory processing and analyses
Chilled water samples were transported to a wetlab at Malheur NWR and kept on ice for no more than 24 h before processing and shipping to an analytical laboratory. In the wetlab, the four 1-L samples from each enclosure were combined and mixed in an 8-L churn splitter (Bel-Art SP Scienceware, South Wayne, NJ, USA) to produce a composite from which samples were drawn and processed for laboratory analyses. Before drawing samples, the specific conductance (μS cm−1), pH, turbidity (NTU), and chlorophyll-a content (μg L−1 and RFU) in the composite was measured with a sonde (YSI model 6920).
Dissolved nutrients samples (P, N, and Si) were collected by filtering ∼40–45 mL of water through surfactant-free cellulose filters with pore size 0.45 μm and diameter 2.8 cm (Corning, Inc., Corning, NY, USA) affixed to a 60-mL syringe. A clean syringe and 60-mL HDPE sample bottle was used for each composite sample, and both were rinsed three times with sample water before collecting the filtrate. Filtrate samples were frozen, shipped on dry ice to the analytical laboratory, and subsequently analysed using a continuous flow automated analyser (SEAL AutoAnalyzer Model AA3, Seal Analytical, Inc., Mequon, WI) after Gordon et al. (1993) and following standard methods (SM4500-) for ammonia (NH4), nitrate (NO3) and nitrite (NO2), phosphate (PO4), and silicic acid (Si(OH)4) (Rice et al. 2012). Samples for total nitrogen (TN) and total phosphorus (TP) were collected by measuring a known volume of composited water (generally 40 mL) with a graduated cylinder rinsed three times with sample water, and pouring the sample into an autoclaved 60-mL HDPE sample bottle rinsed once with sample water. The TN and TP sample was frozen, shipped on dry ice to the analytical laboratory, and analysed using a continuous flow automated analyser (Technicon Model AAII, SEAL Analytical, Inc., Mequon, WI) after SM4500-P-J (Rice et al. 2012).
Total suspended sediments (TSS) samples were collected by filtering a known volume of composite sample water (nearest mL) through a pre-weighed glass fibre filter (GFF) with pore size 0.7 μm and diameter 2.5 cm (Whatman 1825-025, GE Healthcare Life Sciences, Chicago, IL, USA) placed in a 25-mm polysulfone filter funnel (50-mL capacity, Pall Inc., Port Washington, NY, USA) connected to a 125-mL capacity glass filtering flask placed under vacuum (<20 Hg) using a mechanical pump (Welch model 2522B-01, Mount Prospect, IL, USA). Filters were shipped to the analytical laboratory, dried at 103–105°C, and the dry weight of the filtered material was recorded using a microbalance (nearest 0.01 mg, Mettler-Toledo AX205, Columbus, OH, USA) after SM2540-D (Rice et al. 2012).
Chlorophyll-a samples were analysed by filtering a known volume of composite water through the same type of GFF filter, filtration, and vacuum system as for TSS. The filters were placed in plastic vials, covered with aluminium foil, and immediately frozen. Frozen filters were shipped on dry ice to the analytical laboratory where they were extracted with acetone and analysed using fluorometry (Turner Model TD700 Fluorometer, Turner Designs, San Jose, CA, USA) following method EPA 445.0. All laboratory analyses for nutrients, TSS, and chlorophyll-a were performed at the University of Washington’s Marine Chemistry Laboratory.
Submerged aquatic vegetation
SAV surface area coverage
We used aerial imagery collected with a small unmanned aerial system (UAS; 3DR Solo, Berkley, CA, USA) fitted with a multi-spectral camera that takes geo-referenced images (MicaSense RedEdge-M, Seattle, WA, USA) to estimate the surface area coverage of SAV within enclosures and reference plots four separate times (22–26 June, 31 July, 4 September, and 24–25 September) concurrent with monthly water quality sampling. Images were collected by autonomous flights at 91.3-m elevation, 6.1 m s−1 flight speed, 75% endlap, and 75% sidelap, which resulted in a ground sample distance of 6.22 cm. Flights were typically flown in the morning, under clear conditions. We used Agisoft Metashape (ver. 1.5.5, Saint Petersburg, Russian Federation) to convert images to composite georeferenced orthomosaics. We used ArcMap (ver. 10.6, ESRI, Redlands, CA, USA) to perform a raster split to isolate the pond area, and ENVI (ver. 5.5, 64-bit, Harris Geospatial, Broomfield, CO, USA) to create regions of interest (ROI) representing the water surface within each enclosure or reference plot, excluding any shadows. We then developed a supervised maximum likelihood classification routine to assign individual pixels as water or vegetation (see the ‘Additional details for methods’ section in the Supplementary material for additional details), and used ENVI to extract the relative surface coverage of vegetation within each enclosure or reference plot.
SAV composition and biomass
At the end of the study (26–27 September, 1 October 2019) we used ocular surveys in four 1-m2 sample plots (within each enclosure and reference plot) to determine SAV composition and surface coverage. A 1-m2 PVC frame was placed randomly within each of four quadrants of the enclosure (or reference plot), and percentage surface coverage by SAV functional group was estimated (nearest 1%) within the plot area enclosed by the frame. We defined functional groups as ecologically and morphologically similar genera, e.g. pondweeds (Potamogeton, Stukenia, Zanichellia), milfoil (Myriophyllum), or algae (Chara). After estimating surface (canopy) coverage, we gently parted the SAV canopy and recorded the presence of any additional SAV functional groups below the water surface (understorey). We recorded the water depth (nearest cm) at each sample plot. We calculated the Simpson’s dominance index (λp) for each plot (p) based on surface coverage of each taxa as:
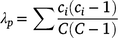
where ci is the proportion surface coverage of the ith taxon and C is the total surface coverage of each sample plot. Simpson’s dominance index ranges from 0 (infinite diversity) to 1 (no diversity; complete dominance by a single taxon). We calculated the arithmetic mean λ for each enclosure based on the four plot-level estimates.
At one randomly selected plot within each enclosure or reference plot, we also collected SAV samples using a two-headed rake similar the methods in US Department of the Interior (2018). For the selected plot, three SAV sub-samples were collected by dragging the rake for 1 m across the pond bottom, twisting the rake head 180° as it is lifted off the bottom, gently sweeping it in the water to remove sediment, and gently compressing the vegetation along the rake tines. Individual sub-samples were drained, placed in labelled plastic bags, and frozen. We estimated SAV biomass per 1-m2 plot by weighing (nearest g) individual rake sub-samples dried in an isothermal oven at 70°C for 4–6 days.
Analyses
Values for nitrate (NO3-N) and nitrite (NO2-N) were combined (NOx-N) before analyses. We also calculated a set of four stoichiometry variables with nitrogen and phosphorus to better understand the nutrient dynamics of Windmill Pond and whether they changed in response to carp. The ratio of soluble N (nitrate + nitrite + ammonium) to total N (soluble N:TN) and the ratio of soluble P (orthophosphate) to total P (soluble P:TP) were calculated to determine whether nitrogen and phosphorus tended to be bioavailable or sequestered. We calculated the ratio of soluble N to soluble P (soluble N:soluble P) and total N to total P (TN:TP) to help understand which nutrient was limiting.
We used linear mixed models and simple linear regression models followed by a set of a priori contrasts to evaluate the effect of carp biomass, fish composition, spatial variation, and enclosure effects on water quality, nutrients, and water surface coverage of SAV. For analysis of carp effects, we created a composite variable termed ‘treatment combination’ with eight levels comprising the open reference plots, zero biomass control enclosures, and the six combinations of carp biomass × fish composition. Data were transformed using ln (water quality parameters and nutrients), logit (nutrient ratios), or arcsine square root (proportion SAV cover) before analyses.
For each dependent variable, we fit a mixed model with block, sample period, treatment combination, and treatment combination × sample period as fixed effects, and enclosure as a random intercept using restricted maximum likelihood (R package lme4, ver. 1.1-21, see https://CRAN.R-project.org/package=lme4; Bates et al. 2015). Enclosure and block could represent much of the same spatial variation in response variables among enclosures, so we conducted a likelihood ratio test of the random enclosure term using the rand function in the R package lmerTest (ver. 3.1-2, see https://CRAN.R-project.org/package=lmerTest; Kuznetsova et al. 2017) and compared the model’s conditional R2 (which includes fixed and random effects) with the marginal R2 (fixed effects only) to evaluate how much additional variation was explained by the random enclosure term. If the likelihood ratio test for the random enclosure term was statistically significant, then the term was retained; fit was assessed by inspecting residuals, and the mixed model was used for linear contrasts. If the random enclosure term was not statistically significant, then it was dropped, and a simple linear model was fit and used for contrasts after inspecting residual plots.
To understand the extent of spatial variation in response variables within the pond, we performed linear contrasts to compare the marginal means among blocks while averaging over the other independent variables (R package emmeans, ver. 1.4.5, R. Lenth, see https://CRAN.R-project.org/package=emmeans). To determine whether there were enclosure effects resulting from isolating water within enclosures, we performed linear contrasts to compare the marginal means of the reference plots to marginal means of the zero biomass control enclosures for each sample period.
To test for an effect of carp biomass, we compared the marginal means for zero biomass controls to those for 50, 100, and 300 kg ha−1 biomass treatments at each sample period (12 comparisons per dependent variable). To test the effect of fish composition, we compared the marginal means of single v. multiple-fish treatments within each level of biomass (50, 100, 300 kg ha−1) during each sample period (12 comparisons total). For both contrasts we adjusted the significance level for comparisons within a biomass level (i.e. family of four comparisons). We used α = 0.1 as the threshold for statistical significance given the modest sample sizes in this study and the desire to reduce the probability of a Type II error. Significance levels of contrasts were adjusted using the Holm method (Holm 1979).
To evaluate whether dry biomass of SAV at the end of the study was affected by the presence and biomass of carp, we conducted an ANCOVA-type analysis. We fit a fixed-effects model for dry biomass of SAV to the ocular estimate of SAV surface water coverage in 1-m2 sample plots and its interaction with the categorical variable for carp biomass. We determined the significance of the interaction term and, subsequently, carp biomass using likelihood ratio tests on nested models, and evaluated any significant effect of carp biomass on SAV biomass using linear contrasts. Owing to small sample sizes and lack of temporal replication, we did not attempt to evaluate enclosure effects, block effects, or differences between single- and multiple-fish biomass carp treatments. For analysis we combined reference plots and zero biomass controls into single group, and at each biomass treatment combined the single- and multiple-fish treatments such that there were four categories for carp biomass (0, 50, 100, and 300 kg ha−1).
Animal research ethics
The use of piscicide (Rotenone) to remove common carp that had invaded the study site within Malheur National Wildlife Refuge was authorised under Pesticide Use Proposal (PUP) number R1-18-13570-020 approved under the authority of the US Fish and Wildlife Service’s Integrated Pest Management Policy (569 FW 1). Use of carp in the experiment followed guidance in Use of Fishes in Research Committee (2014).
Results
Carp
At the time of tagging, the 41 carp stocked in enclosures ranged from 181 to 800 mm TL and from 86 to 6650 g in weight (Table 1). Across all enclosures the stocked biomasses averaged within 2.9, 7.1 and 9.1% of the target biomass for 50, 100, and 300 kg ha−1 treatments. In total, 36 of the 41 carp were recaptured at the end of the study. All four carp escaped from enclosure X-5 (multiple-fish 100 kg ha−1 treatment) because of a separated seam in the enclosure wall, so this enclosure was dropped from the analyses. One fish escaped from enclosure Y-2 (multiple-fish 300 kg ha−1 treatment), but the carp biomass at capture indicated the target biomass of 300 kg ha−1 was achieved so the enclosure was included in analyses. Carp grew well in enclosures, and at recapture fish ranged 246–810 mm TL and 270–7950 g (see Table S1 and Fig. S1 of the Supplementary material). Specific growth rate (Eqn 1) averaged 0.63% day−1 (s.d. = 0.43) and ranged from 0.10 to 1.45% day−1. Specific growth rate was inversely related to initial fish size according to the linear equation: 5.424 − 0.812(ln(TL)) (d.f. = 34, P < 0.001, R2 = 0.90, see Fig. S1). Biomass tended to increase more rapidly in the multiple-fish treatments than the single-fish treatments (Table 1), because smaller fish exhibited higher SGR.

![]() |
General water chemistry and temperature
Windmill Pond water was basic, with a pH ∼9.5–10.0 in early summer and early autumn, and pH 10.0–10.5 in mid-summer (see Fig. S2 in the Supplementary material). Specific conductance exhibited strong spatial variation and was ∼400 μS cm−1 in block A (nearest the pond’s water source; see Fig. 2), but averaged ∼100–200 μS cm−1 higher in blocks B and C. Specific conductance also exhibited a seasonal increase in blocks B and C. Water temperatures at the time of sample collection (morning to early afternoon on a given day), ranged from ∼12.5°C in early autumn to nearly 20°C in summer. Water level in enclosures and reference plots declined between summer and early autumn (see Table S2 of the Supplementary material).
![]() |
Water quality, nutrients, and SAV time-series data
A labelling error within the wetlab resulted in unusable chlorophyll-a data for approximately half the analytical laboratory results from the third sampling occasion. Consequently, for the time-series analysis of chlorophyll-a, we used the measurements recorded with the calibrated sonde. The chlorophyll-a values recorded using the wetlab sonde were highly correlated with the verified analytical laboratory estimates (d.f. = 77, Pearson’s r = 0.90, P < 0.001), from which we infer wetlab sonde data were representative.
During the study, water clarity was generally high in Windmill Pond as chlorophyll-a (mean generally <30 μg L−1), TSS (generally <50 mg L−1), and turbidity (mean generally <20 NTU) were all low (Fig. 3). Mean ammonia concentrations were generally low (<25 μg L−1), nitrite plus nitrate was very low (usually <10 μg L−1), and total N was high (often >2000 μg L−1; see Fig. 4). Mean phosphate concentration ranged from ∼250 to 600 μg L−1, and mean TP was ∼500–900 μg L−1 (Fig. 4). Most of the nitrogen present was not in soluble form, as the ratio of soluble N (ammonium + nitrite + nitrate) to TN was very low (generally <0.015), whereas most of the phosphorus was in soluble form because the ratio of soluble P (phosphate) to TP was ∼0.5–0.8 (Fig. 5). We infer the system may have been nitrogen limited during the study given the low ratio of soluble N to soluble P (Fig. 5). The mean ratio of TN:TP ranged from ∼2.5 to 6.0 (Fig. 5). Before stocking carp, mean surface coverage of SAV averaged 70% overall but was higher in the zero biomass controls (mean = 92%) than in the reference plots and treatment enclosures (mean = 67%; Fig. 6). Mean SAV coverage in the zero biomass controls increased to 97% by the second sample period and remained at or above that level for the remainder of the study, whereas the reference plot remained ∼68–71% surface coverage. Mean SAV coverage for enclosures assigned to carp treatments increased from a pre-stocking average of 67% in late June to 91% in late July and remained very high (mean = 94%) during September (Fig. 6).
![]() |
![]() |
![]() |
Model fitting
Many of the response variables (10 of 14) exhibited evidence of non-constant variance based on Levene’s test, but other diagnostic indicators such as normality of errors and residual plots did not suggest serious violations of regression assumptions, so we concluded the models were valid. We found substantial variation among enclosures (i.e. random intercept for enclosure) for three response variables: chlorophyll-a, soluble N:TN, and surface coverage of SAV (Table 2). Consequently, 11 of 14 of the estimation models were fixed effects models (Table 2). Whereas the random effect was deemed not statistically significant in most of these cases based on a likelihood ratio test, there were three response variables – nirite + nitrate, TN, and soluble P:TP – where we found evidence that including a random intercept for enclosure would result in overfitting (i.e. singularity) so the random term was dropped. For the fixed effects models, we presume that any spatial variation present was best accounted for by the block effect. In most models the carp biomass × sample period variable was not statistically significant (Table 2), but fits included both reference plots and controls (as well as the biomass treatments) so that a single estimation model could be used to evaluate both enclosure and carp effects by linear contrasts. Consequently, the outcome of the linear contrasts were the proper basis for evaluating carp effects, and not the P-value of the carp biomass × sample period interaction term.
![]() |
Spatial variation in water clarity and nutrient concentration appeared to depend on proximity to the pond’s water source with higher clarity and lower nutrient concentrations (except for silica) in block A, whereas SAV surface coverage was greater in block C than block B (see Fig. S3 of the Supplementary material). We found statistically significant differences between reference plots and zero biomass control enclosures in one or more sample periods for 9 of 14 of the time-series variables though there were only a few instances where the difference was consistent through time (see Table S3 of the Supplementary material). To be conservative and because several carp escaped enclosures and were at large in the study area, we used zero biomass controls for the linear contrasts testing carp effects.
Carp treatment effects
Biomass
With a few exceptions, effects of carp were only observed for the 300 kg ha−1 treatment group. Turbidity in the 300 kg ha−1 carp treatment was two to three times that of the zero biomass control in sample periods two (marginal means: 5.6 NTU control v. 17.0 NTU 300 kg ha−1), three (6.5 v. 18.4 NTU) and four (4.9 v. 14.9 NTU; Table 3). Phosphate was elevated in the 300 kg ha−1 biomass treatments compared to the control in sample periods two (marginal means: 178 μg L−1 control v. 328 μg L−1), three (271 v. 523 μg L−1), and four (291 v. 660 μg L−1); however, the contrasts were nearly statistically significant during periods two and three (i.e. P = 0.106; see Table 3). Total P concentrations also appeared ∼200–360 μg L−1 higher at 300 kg ha−1 treatment compared to the zero biomass control (Fig. 4), but the contrast was nearly statistically significant only in the third sample period (marginal means: 538 v. 898 μg L−1, P = 0.124; see Table 3).
Nitrite + nitrate (NOx-N) concentration in the 300 kg ha−1 carp treatment was greater than the control at sample period three (marginal means: 2.8 μg L−1 control v. 6.8 μg L−1; Table 3), but this result was influenced by an unusually high value (40.4 μg L−1) in one enclosure, whereas in general, NOx-N concentrations were almost uniformly low and invariant (Fig. 4). Consequently, we cannot attribute this difference to an effect of carp. Total N concentrations in control enclosures and treatments exhibited a seasonal increase (Fig. 4) and there were a few instances of potential carp effects, but the differences were not consistent by biomass or through time (Table 3). For example, TN concentrations were greater in the carp biomass treatments compared to controls at 100 kg ha−1 in sample period three (marginal mean: 3307 v. 2889 μg L−1), and at 300 kg ha−1 in sample period two (2393 v. 1950 μg L−1; Table 3). There was some evidence that soluble P:TP was greater in carp treatments at the end of the study, as the marginal mean proportions for 50 kg ha−1 (0.70), 100 kg ha−1 (0.67), and 300 kg ha−1 (0.71) were higher than the control (0.50) though the difference was not statistically significant (P = 0.151) for 100 kg ha−1 (Table 3; Fig. 5). At the end of the study TN:TP ratio was marginally lower at 300 kg ha−1 (marginal mean = 3.1) than in the controls (5.3, P = 0.14 for linear contrast; Table 3; Fig. 5), which may reflect the contribution of increased PO4-P concentration (see Fig. 4).
Soluble N:soluble P ratios and TSS also did not appear to be influenced by the presence or density of carp (Table 3). Ammonium, SiO4, and chlorophyll-a exhibited temporal patterns in their concentrations (generally increasing through time, Table 3; Fig. 3, 4) there were no statistically significant increases that could be attributed to the effect of carp biomass (Table 3). In fact, at sample period four the chlorophyll-a concentration was significantly lower in the 50 kg ha−1 biomass (28.0 μg L−1) treatment compared to the control (40.2 μg L−1). Similarly, SAV exhibited an increase in surface water coverage through time and did not change in response to carp (Table 3; Fig. 6). In the first sample period the proportion coverage of SAV was lower in the 50 kg ha−1 carp treatment (marginal mean = 0.62) than in the zero biomass control enclosures (0.93; Table 3), but this reflects initial conditions before stocking of carp. In general, proportion surface coverage of SAV was very high in enclosures where carp were present; by the second sample period marginal means ranged 0.91–0.97 across the three biomass treatment, and by the third period surface coverage was near total (0.96–1.0; Fig. 6).
Fish composition
In 300 kg ha−1 treatments, turbidity in the multiple-fish treatments was approximately double that in the single-fish treatments during the third (marginal means = 26.5 v. 12.7 NTU) and fourth sample periods (21.7 v. 10.3 NTU), though statistically the differences were marginal (P = 0.102, Table 3). Ammonium concentration, soluble N:TN, and soluble N:soluble P were all greater in the single-fish treatments for 100 kg ha−1 the first sample period, but these reflect initial conditions before stocking carp. Total nitrogen concentration was ∼33% higher in the 300 kg ha−1 multiple-fish treatment (marginal mean = 3401 μg L−1) compared to the single-fish treatment (2548 μg L−1) but only in the final sample period when the overall 300 kg ha−1 biomass treatment was statistically indistinguishable from the zero biomass control (Table 3). We did not detect any statistically significant differences between single- and multiple-fish treatments for the other nine response variables (Table 3).
SAV coverage and biomass from direct sampling
Mean ocular estimates of percentage SAV coverage based on the four 1-m2 sample plots ranged 66–100% (mean = 92.1%; Fig. 7a) and was highly correlated with the estimated surface coverage of SAV derived from UAV aerial imagery in the final sample period (d.f. = 21, r = 0.88, P < 0.001). This suggests the ocular estimates were representative of SAV coverage within an enclosure. Milfoil (genus Myriophyllum) was the most common taxon of SAV observed and was present in every sample plot in every enclosure (see Table S4 and Fig. S4 of the Supplementary material). Thin-leaf pondweed (genus Stuckenia) and broad-leaf pondweed (genus Potamogeton) were present in either the SAV canopy or understorey in all 24 and 13 enclosures respectively. The algae Chara was present but rare. Simpson’s index of species dominance (λ) was nearly uniformly high across 23 enclosures and reference plots (mean = 0.93, lower quartile = 0.89, range = 0.75–0.995; Fig. 7a), which reflected milfoil’s near total dominance of the SAV canopy in most enclosures. The one exception to canopy dominance by milfoil was in enclosure X-5 (average λ = 0.74), where Stuckenia was dominant (see also Table S4).
Dry biomass of SAV averaged 366.8 g m−2 for the individual 1-m2 sample plots within the 23 enclosures included in analyses (range = 166.2−598.2 g m−2; Fig. 7b; see also Table S4). Biomass of SAV was not related to the presence or biomass of carp, as neither the percentage surface coverage of SAV × carp biomass interaction (likelihood ratio test: = 3.7, P = 0.30) nor the carp biomass alone were statistically significant (
= 0.76, P = 0.86); nor was it related to the ocular estimate for surface coverage of SAV within the plot where the sample was collected (
= 1.97, P = 0.16).
Discussion
Effect of carp on nutrients, phytoplankton, and aquatic vegetation in Windmill Pond
Our study indicated that a semi-natural wetland (pond) with abundant macrophytes can resist a phase shift from clear to turbid water over one growing season at carp biomasses up to 300–450 kg ha−1. Water clarity was affected by carp, as we observed an increase in turbidity through time at the 300 kg ha−1 biomass treatment. Presumably, this was a result of feeding-related bioturbation by the carp (Ritvo et al. 2004; Matsuzaki et al. 2007; Huser et al. 2016). Despite the presence of carp, turbidity generally remained below 20 NTU, close to a threshold at which dramatic decreases in diversity and biomass of SAV has been observed in other systems (Lougheed et al. 1998; Badiou and Goldsborough 2015), and only exceeded 20 NTU, on average, for the 300 kg ha−1 multiple-fish treatment. Dissolved phosphorus (orthophosphate, PO4-P) increased in the highest carp biomass, though the result was marginally significant until the final sampling occasion. Total phosphorus also appeared to increase at the same carp treatment, though the trend was not statistically significant. Variability in phosphorus concentrations and application of a conservative adjustment to the test statistic (Holm’s method) influenced this statistical outcome. Regardless, TP and PO4-P values were highly correlated (r = 0.89), which suggests that much of observed changes in TP resulted from the increase in the soluble fraction. By the end of the study in late September, the ratio of soluble P to TP was 34–42% higher across all three biomass treatments. This suggests that phosphorous was not a limiting nutrient at the study site – an observation also supported by the very low soluble N:soluble P ratios – and that excretion by carp may have contributed to an accumulation of soluble phosphorus at all treatment levels.
We did not observe wholesale changes in water clarity, nutrient concentrations, or aquatic macrophytes in response to carp. In part, this was expected because the study design included carp biomass threshold levels reported in the literature – 50 kg ha−1 (Vilizzi et al. 2015) and 100 kg ha−1 (Bajer et al. 2009) – at or near levels that different components of the aquatic ecosystem may not be affected by carp. Changes to water quality, nutrients, invertebrates, and aquatic vegetation at 50 kg ha−1 carp biomass may be infrequent, and impacts would most likely occur at higher trophic levels, such as in fish and birds (Vilizzi et al. 2015). Indeed, this was much the case in the current study as we saw few, if any, carp-related effects on turbidity and phosphorus concentrations apart from those at the 300 kg ha−1 treatment. Other studies have also found that not all measured response variables were affected by carp (e.g. Fletcher et al. 1985; Angeler et al. 2002; Badiou and Goldsborough 2015; Marshall et al. 2019; see also reviews by Weber and Brown 2009; Vilizzi et al. 2015). Our study was conducted over ∼100 days within a single growing season, however much longer periods may be needed to observe a phase shift in stable states caused by carp (e.g. Zambrano and Hinojosa 1999). The measurable outcomes for such field experiments will always be, to some extent, idiosyncratic because of the complex dynamics between abiotic and biotic processes that structure aquatic systems (Weber and Brown 2009). We speculate that water clarity did not change dramatically despite the presence of carp, in part, because of a robust prey base. Although not explicitly sampled as a part of this study, aquatic insects (e.g. larval Dipterans and Odonates) were abundant and amphibians (especially frogs) were present in enclosures, which suggests a functional food web with adequate prey base for multiple species of vertebrates. We did not measure carp diets, but we did observe that they grew well during the study (see Table S1 and Fig. S1). Bioturbation by carp can depend on food availability and foraging time (Werner and Anholt 1993; Zambrano and Hinojosa 1999; Zambrano et al. 2001; Badiou and Goldsborough 2010), so abundant prey could have established conditions for less disturbance and sediment resuspension caused by the carp feeding behaviour.
Meta-analyses of carp studies from many continents support the hypothesis that carp reduce the density of aquatic macrophytes (e.g. Vilizzi et al. 2015). The uprooting of SAV is believed to be one of the mechanisms by which carp alter aquatic habitats and facilitate a phase shift to turbid water (Weber and Brown 2009). Consequently, we assumed there would be a significant reduction in the water surface coverage of SAV within enclosures containing the highest carp biomass treatment category (300 kg ha−1). Instead, we found that surface coverage of SAV was stable or even increased during summer and early autumn. Not all taxa of aquatic vegetation are equally vulnerable to physical disturbance by carp (e.g. Drenner et al. 1998; Chumchal et al. 2005; Miller and Crowl 2006), and effects may depend on plant morphology. For example, evidence from exclosure and pond experiments indicates the macroalgae Chara is more sensitive to the presence of common carp than sago pondweed (S. pectinatus; e.g. King and Hunt 1967; Parkos et al. 2003; Evelsizer and Turner 2006), with the inference that stronger root systems confer greater resistance to carp. Species of eel grass (Vallisneria) also tend to be strongly affected by carp (e.g. Roberts et al. 1995) leading to speculation that SAV that form canopies (e.g. Hydrilla) may be more resistant to carp than species that have more of their biomass within the water column and grow in meadows (e.g. Vallisneria, Qiu et al. 2019). Milfoil (genus Myriophyllum) can form dense canopies and appears to be one of the more resistant SAV taxa to physical disturbance by carp. In an Australian pond, abundance of native milfoil (M. papillosum) did not change in the presence of carp and the authors found that the force required to break the root tissue was higher than that for Chara and Vallisnera, which were significantly reduced in carp treatments (Roberts et al. 1995). The timing of vegetation growth may influence whether carp affect SAV, and young, rapidly growing plants are presumably more vulnerable (King and Hunt 1967). At the outset of our study, northern milfoil M. sibiricum had already formed a dense canopy within enclosures (e.g. Fig. 6; see also Fig. S4). Given the initial conditions of a well-established and abundant SAV taxa with comparatively strong root system, coupled with a moderate (∼300 kg ha−1) high-biomass treatment, it was perhaps unsurprising that SAV surface coverage did not decrease in the presence of carp.
Aquatic macrophytes and alternative stable states in ponds and shallow lakes
A suite of factors can collectively reinforce feedback loops that maintain a particular stable state (e.g. Hargeby et al. 2004), and we presume the presence of robust stands of aquatic macrophytes, particularly M. sibiricum, was a main driver that stabilised the clear state in Windmill Pond. Aquatic macrophytes can reduce wind-driven sediment resuspension by shortening the effective wind fetch, which increases sedimentation (e.g. Roberts et al. 1995; Badiou and Goldsborough 2015) and subsequently reduces internal phosphorous loading, promoting the growth of benthic algae (Horppila et al. 2013; Zhang et al. 2013). Benthic algae can further remove nutrients from the water column and decrease the susceptibility of the sediment to wind resuspension (Zhang et al. 2013). Additionally, aquatic macrophytes can directly limit phytoplankton growth by outcompeting them for nutrients both in the water column and in the sediment (Carpenter et al. 1983; Ozimek et al. 1993; Williams 2005), by releasing allelopathic substances that limit growth of algae (Wium-Andersen et al. 1982; reviewed by van Donk and van de Bund 2002), and by providing habitat for zooplankton that feed upon algae (Timms and Moss 1984). Consequently, any nutrients mobilised by carp by feeding or excretion (e.g. Driver et al. 2005; Matsuzaki et al. 2007) might not lead to increases in chlorophyll-a and turbidity.
The uniformly high surface coverage of SAV at moderate-to-high biomass (up to 600 g m−2 based on limited sampling; cf. Drenner et al. 1997) suggests a potential to buffer effects of bioturbation by carp in Windmill Pond. Others have speculated about a regulating effect of Myriophyllum on bioturbation by carp. For example, in Texas, USA, Drenner et al. (1998) observed that turbidity, TP, and chlorophyll-a were lower in ponds with high biomass of Eurasian milfoil (M. spicatum, >1 kg m−2 for air-dried specimens), and hypothesised that M. spicatum regulated nutrient-carp synergisms. We know from previous sampling that higher turbidity and higher concentrations of chlorophyll-a and nutrients are possible in Windmill Pond than were observed in 2019.
Single carp v. multiple carp and the challenge of a biomass threshold value
We sought to evaluate whether the effect of carp biomass depended on the size and number of carp, but fish growth made it difficult to attribute any changes in the response variables to fish composition per se. Physical disturbance and nutrient excretion by carp can depend on the size and number of fish present (e.g. Driver et al. 2005; Kloskowski 2011; Morgan and Hicks 2013), so environmental variation and size-selective suppression might produce very different population structures in wild fish populations (Longhurst 2002). Turbidity in the multiple-fish 300 kg ha−1 treatment was approximately double the single-fish treatment in the final two sample periods. However, by the end of the study the carp biomass in the multiple-fish 300 kg ha−1 treatments was higher than the single-fish 300 kg ha−1 treatments in two of three blocks, and would likely have been higher in all three if one fish had not escaped one of the multiple-fish enclosures (Table 1). The relative differences in final carp biomass between single- and multiple-fish were even greater for the 50 and 100 kg ha−1 treatments, such that fish composition and biomass treatments were confounded.
The confounding results underscore that measuring fish growth relative to the time when the treatment biomass is measured can be a perplexing design consideration for these types of studies. Treatment biomass of carp has been characterised variously as initial value (e.g. Roberts et al. 1995; Lougheed et al. 1998; Matsuzaki et al. 2007, 2009), final value (e.g. Zambrano and Hinojosa 1999; Chumchal et al. 2005), or estimated as time series concurrent with the response variables (e.g. Vilizzi et al. 2014). Such temporal variability in the treatment effect of biomass suggests critical biomass or threshold values gleaned from individual studies (Bajer et al. 2009), qualitative review (Weber and Brown 2009), or meta-analyses (Matsuzaki et al. 2009; Vilizzi et al. 2015) are best considered approximations with heuristic value and should be applied cautiously to specific management situations in the absence of site-specific data. For analyses, we designated carp biomass as a categorical variable, but this still provides actionable information when both endpoints (beginning and ending biomasses) are considered. For example, we saw few effects of carp below 200 kg ha−1 biomass (highest endpoint for 100 kg ha−1 treatment), and only began to see an increase in turbidity and perhaps phosphorus at 300 kg ha−1 or higher biomass (lower endpoint for 300 kg ha−1 treatment).
Management implications for Malheur Lake and other shallow lakes
Our data indicated a ‘maintenance biomass threshold’ for carp of <200 kg ha−1 in Windmill Pond because we saw few, if any, changes to water clarity, nutrients, or SAV in the 50 kg ha−1 and 100 kg ha−1 carp treatments in which carp biomass increased to ∼200 kg ha−1 by the end of the experiment. We believe this demonstrates a resistance to change that an intact aquatic ecosystem can exhibit when faced with a non-catastrophic perturbation, in this instance the introduction of non-native bethivorous carp at moderate density. The carp biomass at which SAV would begin to re-establish in turbid-water system presently lacking SAV, like Malheur Lake, is unknown but may be characterised as a ‘recovery biomass threshold’. The recovery threshold first must be achieved to facilitate growth of SAV from the latent seed bank, and only after SAV were well established would the maintenance threshold apply. The recovery threshold would likely require much lower carp biomass than the maintenance threshold due to hysteresis, where a greater energy or perturbation may be necessary to overcome the current momentum pulling the system towards the turbid state (e.g. resiliency; Scheffer 1998). To achieve the recovery threshold for carp, a suite of control measures that concurrently target multiple life history stages may be needed (e.g. Brown and Gilligan 2014; Thresher et al. 2014; Pearson et al. 2019; Yick et al. 2021). The development of techniques to reduce non-native carp is an active area of research, including several proposed methods such as: Judas fish (Bajer et al. 2011), separation cages (Stuart and Conallin 2018), cyprinid herpesvirus (McColl et al. 2014), embryo electroshocking (Simpson et al. 2018), daughterless carp (Thresher et al. 2014), and spawning sabotage (Yick et al. 2021).
Our results suggest an important, though not novel, lesson for management of Malheur Lake and other shallow lakes: a clear-water state can persist in the presence of carp provided SAV are established and abundant. The need to reestablish a robust SAV community as pre-condition for a phase shift in shallow lakes from turbid- to clear-water has been recognised for some time (e.g. Scheffer et al. 1993; Williams 2005; Weber and Brown 2009). Currently, Malheur Lake is likely highly resilient to any restoration actions due to feedback loops that have been strengthened by decades-long persistence of the turbid state, in which wind fetches have extended, rooted vegetation has disappeared, and easily re-suspendable fine sediment has accumulated (Pearson 2020; T. Wood and C. Smith, US Geological Survey, pers. comm.). Thus, a single small-scale perturbation – for example, the commercial harvest of adult carp – will likely be absorbed by the system with little to no change, therefore a large-scale perturbation will likely be necessary to overcome the system’s resilence (Bachmann et al. 1999). A comprehensive approach to controlling carp and achieving the recovery biomass threshold may be a prerequisite for a phase shift in Malheur Lake, but additional non-biotic interventions – e.g. hydrologic manipulation, reduction of wind fetch, chemical treatments to facilitate sedimentation – may be necessary to help managers overcome the existing momentum that maintains the turbid-water state (Griffiths 1978; Pearson 2020). Our results also highlight that systems currently in a clear state could benefit from management actions that maintain or strengthen the positive reinforcing feedback loops – encouraging growth and spread of native rooted vegetation that is less susceptible to damage from bethivorous fishes, creating areas protected from wind re-suspension of fine sediments, ensuring high quality water inputs – that enable these systems to be more resistant to impacts from non-native species.
Supplementary material
Supplementary material is available online.
Data availability
The data that support this study will be shared upon reasonable request to the corresponding author.
Conflicts of interest
The authors declare that they have no conflicts of interest.
Declaration of funding
Primary funding was provided by the Oregon Watershed Enhancement Board (Project number 216-8202-12981) administered by the Harney County Watershed Council. Supplementary funding was provided by Abernathy Fish Technology Center and Malheur NWR.
Acknowledgements
Linda Beck (Malheur NWR) first recognised the research need and the potential study site and helped secure funding to begin the study, and Joseph Barnett (Malheur NWR) helped coordinate construction of enclosures and removal of carp from the study site. We thank Karen Moon (Harney County Watershed Council), Steve Dyer (Abernathy Fish Technology Center), and Suzanne McConnell (Malheur NWR) for administrative support. We thank the dozens of staff from Abernathy Fish Technology Center, USFWS Columbia River Fish and Wildlife Conservation Office, Malheur National Wildlife Refuge, High Desert Partnership, and Friends of Malheur National Wildlife Refuge who helped build enclosures and collect data, including James Archibald, Ashley Carr, Ben Cates, Mark Chowning, Norman Clippinger, Chanice Davies, Robert Esquivel, Sean Fitzmaurice, Christine Ford, J. P. Freerichsen, Brianna Goehring, Rachel Gugler, Nicole Hams, Kelli Hawke, Racheal Headley, Benjamen Kennedy, Alexa Martinez, Alina Nestjorkina, Nicole Pauley, Shelley Pickett, Jamie Sprando, Rikeem Sholes, Ed Sparks, Katey Strailey, and others whose names we may have overlooked. Ben Cate (High Desert Partnership) and David Banks (Oregon Department of Fish and Wildlife) coordinated capture of carp used in the study. Liz Cruz (USFWS, Pacific Region Refuges) made the site map that appears as Fig. 1, Dan Craver (USFWS, Pacific Region Refuges) helped with the GIS exercise to select enclosure locations, and Jeena Koenig (USFWS, Midwest Fisheries Center) provided additional GIS support. Nicole Pauley (USFWS, Directorate Fellow) developed the workflow and processing routines in ArcMap, ENVI, and Metashape that converted the UAS (drone) imagery to spatial data layers, which allowed estimation of water surface coverage by SAV; she also produced the orthomosaic base maps that were used for Fig. 2. Richard Erickson and Brian Gray (US Geological Survey) provided helpful suggestions on statistical analyses. Ron Twibell (Abernathy Fish Technology Center) provided helpful comments on an earlier version of the manuscript.
References
Angeler, DG, Álvarez-Cobelas, M, Sánchez-Carrillo, S, and Rodrigo, MA (2002). Assessment of exotic fish impacts on water quality and zooplankton in a degraded semi-arid floodplain wetland. Aquatic Sciences 64, 76–86.| Assessment of exotic fish impacts on water quality and zooplankton in a degraded semi-arid floodplain wetland.Crossref | GoogleScholarGoogle Scholar |
Bachmann, RW, Hoyer, MV, and Canfield, DE (1999). The restoration of Lake Apopka in relation to alternative stable states. Hydrobiologia 394, 219–232.
| The restoration of Lake Apopka in relation to alternative stable states.Crossref | GoogleScholarGoogle Scholar |
Badiou, PHJ, and Goldsborough, LG (2010). Ecological impacts of an exotic benthivorous fish in large experimental wetlands, Delta Marsh, Canada. Wetlands 30, 657–667.
| Ecological impacts of an exotic benthivorous fish in large experimental wetlands, Delta Marsh, Canada.Crossref | GoogleScholarGoogle Scholar |
Badiou, PHJ, and Goldsborough, LG (2015). Ecological impacts of an exotic benthivorous fish, the common carp (Cyprinus carpio L.), on water quality, sedimentation, and submerged macrophyte biomass in wetland mesocosms. Hydrobiologia 755, 107–121.
| Ecological impacts of an exotic benthivorous fish, the common carp (Cyprinus carpio L.), on water quality, sedimentation, and submerged macrophyte biomass in wetland mesocosms.Crossref | GoogleScholarGoogle Scholar |
Bajer, PG, and Sorensen, PW (2010). Recruitment and abundance of an invasive fish, the common carp, is driven by its propensity to invade and reproduce in basins that experience winter-time hypoxia in interconnected lakes. Biological Invasions 12, 1101–1112.
| Recruitment and abundance of an invasive fish, the common carp, is driven by its propensity to invade and reproduce in basins that experience winter-time hypoxia in interconnected lakes.Crossref | GoogleScholarGoogle Scholar |
Bajer, PG, Sullivan, G, and Sorensen, PW (2009). Effects of a rapidly increasing population of common carp on vegetative cover and waterfowl in a recently restored Midwestern shallow lake. Hydrobiologia 632, 235–245.
| Effects of a rapidly increasing population of common carp on vegetative cover and waterfowl in a recently restored Midwestern shallow lake.Crossref | GoogleScholarGoogle Scholar |
Bajer, PG, Chizinski, CJ, and Sorensen, PW (2011). Using the Judas technique to locate and remove wintertime aggregations of invasive common carp. Fisheries Management and Ecology 18, 497–505.
| Using the Judas technique to locate and remove wintertime aggregations of invasive common carp.Crossref | GoogleScholarGoogle Scholar |
Bates, D, Mächler, M, Bolker, B, and Walker, S (2015). Fitting linear mixed-effects models using lme4. Journal of Statistical Software 67, 1–48.
| Fitting linear mixed-effects models using lme4.Crossref | GoogleScholarGoogle Scholar |
Brown, P, and Gilligan, D (2014). Optimising an integrated pest-management strategy for a spatially structured population of common carp (Cyprinus carpio) using meta-population modelling. Marine and Freshwater Research 65, 538–550.
| Optimising an integrated pest-management strategy for a spatially structured population of common carp (Cyprinus carpio) using meta-population modelling.Crossref | GoogleScholarGoogle Scholar |
Brown, P, and Walker, TI (2004). CARPSIM: stochastic simulation modelling of wild carp (Cyprinus carpio L.) population dynamics, with applications to pest control. Ecological Modelling 176, 83–97.
| CARPSIM: stochastic simulation modelling of wild carp (Cyprinus carpio L.) population dynamics, with applications to pest control.Crossref | GoogleScholarGoogle Scholar |
Carpenter, SR, Elser, JJ, and Olson, KM (1983). Effects of roots of Myriophyllum verticillatum L. on sediment redox conditions. Aquatic Botany 17, 243–249.
| Effects of roots of Myriophyllum verticillatum L. on sediment redox conditions.Crossref | GoogleScholarGoogle Scholar |
Chumchal, MM, Nowlin, WH, and Drenner, RW (2005). Biomass-dependent effects of common carp on water quality in shallow ponds. Hydrobiologia 545, 271–277.
| Biomass-dependent effects of common carp on water quality in shallow ponds.Crossref | GoogleScholarGoogle Scholar |
Cornely JE (1982) Waterfowl production at Malheur National Wildlife Refuge, 1942–1980. US Fish and Wildlife Publications 38, US Fish and Wildlife Service, Washington, DC, USA.
Crooks, JA (2002). Characterizing ecosystem-level consequences of biological invasions: the role of ecosystem engineers. Oikos 97, 153–166.
| Characterizing ecosystem-level consequences of biological invasions: the role of ecosystem engineers.Crossref | GoogleScholarGoogle Scholar |
Drenner, RW, Gallo, KL, Edwards, CM, Rieger, KE, and Dibble, ED (1997). Common carp affect turbidity and angler catch rates of largemouth bass in ponds. North American Journal of Fisheries Management 17, 1010–1013.
| Common carp affect turbidity and angler catch rates of largemouth bass in ponds.Crossref | GoogleScholarGoogle Scholar |
Drenner, RW, Gallo, KL, Baca, RM, and Smith, JD (1998). Synergistic effects of nutrient loading and omnivorous fish on phytoplankton biomass. Canadian Journal of Fisheries and Aquatic Sciences 55, 2087–2096.
| Synergistic effects of nutrient loading and omnivorous fish on phytoplankton biomass.Crossref | GoogleScholarGoogle Scholar |
Driver, PD, Closs, GP, and Koen, T (2005). The effects of size and density of carp (Cyprinus carpio L.) on water quality in an experimental pond. Archiv für Hydrobiologie 163, 117–131.
| The effects of size and density of carp (Cyprinus carpio L.) on water quality in an experimental pond.Crossref | GoogleScholarGoogle Scholar |
Duebbert HF (1969) The ecology of Malheur Lake and management implications. Refuge Leaflet 412, US Department of Interior, US Fish and Wildlife Service, Bureau of Sport Fisheries and Wildlife, Washington, DC, USA.
Evelsizer, VD, and Turner, AM (2006). Species-specific responses of aquatic macrophytes to fish exclusion in a prairie marsh: a manipulative experiment. Wetlands 26, 430–437.
| Species-specific responses of aquatic macrophytes to fish exclusion in a prairie marsh: a manipulative experiment.Crossref | GoogleScholarGoogle Scholar |
Fletcher, AR, Morison, AK, and Hume, DJ (1985). Effects of carp, Cyprinus carpio L., on communities of aquatic vegetation and turbidity of waterbodies in the Lower Goulburn River basin. Australian Journal of Marine and Freshwater Research 36, 311–327.
| Effects of carp, Cyprinus carpio L., on communities of aquatic vegetation and turbidity of waterbodies in the Lower Goulburn River basin.Crossref | GoogleScholarGoogle Scholar |
Gordon LI, Jennings JC Jr, Ross AA, Krest JM (1993) A suggested protocol for continuous automated analysis of seawater nutrients (phosphate, nitrate, nitrite and silicic acid) on the WOCE Hydrographic Program and the Joint Global Ocean Fluxes Study, Technical Report 93-1. WOCE Hydrographic Program Office, Methods Manual WHPO 91-1, Oregon State University, College of Oceanography and Atmospheric Sciences, Corvallis, OR, USA.
Green, SJ, and Grosholz, ED (2021). Functional eradication as a framework for invasive species control. Frontiers in Ecology and the Environment 19, 98–107.
| Functional eradication as a framework for invasive species control.Crossref | GoogleScholarGoogle Scholar |
Griffiths FR (1978) Carp control study – control methods and their application to Malheur National Wildlife Refuge, Project number MLH-15. US Fish and Wildlife Service, Malheur National Wildlife Refuge, Burns, OR, USA.
Güneralp, B, and Barlas, Y (2003). Dynamic modelling of a shallow freshwater lake for ecological and economic sustainability. Ecological Modelling 167, 115–138.
| Dynamic modelling of a shallow freshwater lake for ecological and economic sustainability.Crossref | GoogleScholarGoogle Scholar |
Hargeby, A, Blindow, I, and Hansson, L-A (2004). Shifts between clear and turbid states in a shallow lake: multi-causal stress from climate, nutrients and biotic interactions. Archiv für Hydrobiologie 161, 433–454.
| Shifts between clear and turbid states in a shallow lake: multi-causal stress from climate, nutrients and biotic interactions.Crossref | GoogleScholarGoogle Scholar |
Hill, MS, Zydlewski, GB, Zydlewski, JD, and Gasvoda, JM (2006). Development and evaluation of portable PIT tag detection units: PITpacks. Fisheries Research 77, 102–109.
| Development and evaluation of portable PIT tag detection units: PITpacks.Crossref | GoogleScholarGoogle Scholar |
Hillyard, KA, Smith, BB, Conallin, AJ, and Gillanders, BM (2010). Optimising exclusion screens to control exotic carp in an Australian lowland river. Marine and Freshwater Research 61, 418–429.
| Optimising exclusion screens to control exotic carp in an Australian lowland river.Crossref | GoogleScholarGoogle Scholar |
Holm, S (1979). A simple sequentially rejective multiple test procedure. Scandinavian Journal of Statistics 6, 65–70.
Horppila, J, Kaitaranta, J, Joensuu, L, and Nurminen, L (2013). Influence of emergent macrophyte (Phragmites australis) density on water turbulence and erosion of organic-rich sediment. Journal of Hydrodynamics 25, 288–293.
| Influence of emergent macrophyte (Phragmites australis) density on water turbulence and erosion of organic-rich sediment.Crossref | GoogleScholarGoogle Scholar |
Hubbard LL (1975) Hydrology of Malheur Lake, Harney County, southeastern Oregon, Water Resources Inventory 21-75. US Geological Survey, Portland, OR, USA.
Huser, BJ, Bajer, PG, Chizinski, CJ, and Sorensen, PW (2016). Effects of common carp (Cyprinus carpio) on sediment mixing depth and mobile phosphorus mass in the active sediment layer of a shallow lake. Hydrobiologia 763, 23–33.
| Effects of common carp (Cyprinus carpio) on sediment mixing depth and mobile phosphorus mass in the active sediment layer of a shallow lake.Crossref | GoogleScholarGoogle Scholar |
Huser, BJ, Bajer, PG, Kittelson, S, Christenson, S, and Menken, K (2022). Changes to water quality and sediment phosphorus forms in a shallow, eutrophic lake after removal of common carp (Cyprinus carpio). Inland Waters 12, 33–46.
| Changes to water quality and sediment phosphorus forms in a shallow, eutrophic lake after removal of common carp (Cyprinus carpio).Crossref | GoogleScholarGoogle Scholar |
Ivey GL, Cornely JE, Ehlers BD (1998) Carp impacts on waterfowl at Malheur National Wildlife Refuge, Oregon. In ‘Transactions of the 63rd North American Wildlife and Natural Resources conference’, 20–24 March 1998, Orlando, FL, USA. pp. 66–74. (Wildlife Management Institute: Washington, DC, USA)
Janssen, ABG, Teurlincx, S, An, S, Janse, JH, Paerl, HW, and Mooij, WM (2014). Alternative stable states in large shallow lakes? Journal of Great Lakes Research 40, 813–826.
| Alternative stable states in large shallow lakes?Crossref | GoogleScholarGoogle Scholar |
Jones, CG, Lawton, JH, and Shachak, M (1994). Organisms as ecosystem engineers. Oikos 69, 373–386.
| Organisms as ecosystem engineers.Crossref | GoogleScholarGoogle Scholar |
Kaemingk, MA, Jolley, JC, Paukert, CP, Willis, DW, Henderson, K, Holland, RS, Wanner, GA, and Lindvall, ML (2017). Common carp disrupt ecosystem structure and function through middle-out effects. Marine and Freshwater Research 68, 718–731.
| Common carp disrupt ecosystem structure and function through middle-out effects.Crossref | GoogleScholarGoogle Scholar |
King, DR, and Hunt, GS (1967). Effect of carp on vegetation in a Lake Erie marsh. The Journal of Wildlife Management 31, 181–188.
| Effect of carp on vegetation in a Lake Erie marsh.Crossref | GoogleScholarGoogle Scholar |
Kloskowski, J (2011). Differential effects of age-structured common carp (Cyprinus carpio) stocks on pond invertebrate communities: implications for recreational and wildlife use of farm ponds. Aquaculture International 19, 1151–1164.
| Differential effects of age-structured common carp (Cyprinus carpio) stocks on pond invertebrate communities: implications for recreational and wildlife use of farm ponds.Crossref | GoogleScholarGoogle Scholar |
Koehn J, Brumley A, Gehrke P (2000) ‘Managing the impacts of carp.’ (Bureau of Rural Sciences, Department of Agriculture, Fisheries and Forestry: Canberra, ACT, Australia)
Kopf, RK, Humphries, P, Bond, NR, Sims, NC, Watts, RJ, Thompson, RM, Hladyz, S, Koehn, JD, King, AJ, McCasker, N, and McDonald, S (2019). Macroecology of fish community biomass–size structure: effects of invasive species and river regulation. Canadian Journal of Fisheries and Aquatic Sciences 76, 109–122.
| Macroecology of fish community biomass–size structure: effects of invasive species and river regulation.Crossref | GoogleScholarGoogle Scholar |
Kuznetsova, A, Brockhoff, PB, and Christensen, RHB (2017). lmerTest package: tests in linear mixed effects models. Journal of Statistical Software 82, 1–26.
| lmerTest package: tests in linear mixed effects models.Crossref | GoogleScholarGoogle Scholar |
Longhurst, A (2002). Murphy’s law revisited: longevity as a factor in recruitment to fish populations. Fisheries Research 56, 125–131.
| Murphy’s law revisited: longevity as a factor in recruitment to fish populations.Crossref | GoogleScholarGoogle Scholar |
Lougheed, VL, Crosbie, B, and Chow-Fraser, P (1998). Predictions on the effect of common carp (Cyprinus carpio) exclusion on water quality, zooplankton, and submergent macrophytes in a Great Lakes wetland. Canadian Journal of Fisheries and Aquatic Sciences 55, 1189–1197.
| Predictions on the effect of common carp (Cyprinus carpio) exclusion on water quality, zooplankton, and submergent macrophytes in a Great Lakes wetland.Crossref | GoogleScholarGoogle Scholar |
Lowe S, Boudjelas S, De Poorter M (2000) 100 of the world’s worst invasive alien species: a selection from the Global Invasive Species Database. (IUCN Invasive Species Specialist Group, ISSG) Available at http://www.issg.org/pdf/publications/worst_100/english_100_worst.pdf [Verified 15 March 2021]
Maceda-Veiga, A, López, R, and Green, AJ (2017). Dramatic impact of alien carp Cyprinus carpio on globally threatened diving ducks and other waterbirds in Mediterranean shallow lakes. Biological Conservation 212, 74–85.
| Dramatic impact of alien carp Cyprinus carpio on globally threatened diving ducks and other waterbirds in Mediterranean shallow lakes.Crossref | GoogleScholarGoogle Scholar |
Maiztegui, T, Baigún, CRM, Garcia de Souza, JR, Weyl, OLF, and Colautti, DC (2019). Population responses of common carp Cyprinus carpio to floods and droughts in the Pampean wetlands of South America. NeoBiota 48, 25–44.
| Population responses of common carp Cyprinus carpio to floods and droughts in the Pampean wetlands of South America.Crossref | GoogleScholarGoogle Scholar |
Marshall, JC, Blessing, JJ, Clifford, SE, Hodges, KM, Negus, PM, and Steward, AL (2019). Ecological impacts of invasive carp in Australian dryland rivers. Aquatic Conservation: Marine and Freshwater Ecosystems 29, 1870–1889.
| Ecological impacts of invasive carp in Australian dryland rivers.Crossref | GoogleScholarGoogle Scholar |
Matsuzaki, SS, Usio, N, Takamura, N, and Washitani, I (2007). Effects of common carp on nutrient dynamics and littoral community composition: roles of excretion and bioturbation. Fundamental and Applied Limnology Archiv für Hydrobiology 168, 27–38.
| Effects of common carp on nutrient dynamics and littoral community composition: roles of excretion and bioturbation.Crossref | GoogleScholarGoogle Scholar |
Matsuzaki, SS, Usio, N, Takamura, N, and Washitani, I (2009). Contrasting impacts of invasive engineers on freshwater ecosystems: an experiment and meta-analysis. Oecologia 158, 673–686.
| Contrasting impacts of invasive engineers on freshwater ecosystems: an experiment and meta-analysis.Crossref | GoogleScholarGoogle Scholar | 18941787PubMed |
McColl, KA, Cooke, BD, and Sunarto, A (2014). Viral biocontrol of invasive vertebrates: lessons from the past applied to cyprinid herpesvirus-3 and carp (Cyprinus carpio) control in Australia. Biological Control 72, 109–117.
| Viral biocontrol of invasive vertebrates: lessons from the past applied to cyprinid herpesvirus-3 and carp (Cyprinus carpio) control in Australia.Crossref | GoogleScholarGoogle Scholar |
Miller, SA, and Crowl, TA (2006). Effects of common carp (Cyprinus carpio) on macrophytes and invertebrate communities in a shallow lake. Freshwater Biology 51, 85–94.
| Effects of common carp (Cyprinus carpio) on macrophytes and invertebrate communities in a shallow lake.Crossref | GoogleScholarGoogle Scholar |
Morgan, DKJ, and Hicks, BJ (2013). A metabolic theory of ecology applied to temperature and mass dependence of N and P excretion by common carp. Hydrobiologia 705, 135–145.
| A metabolic theory of ecology applied to temperature and mass dependence of N and P excretion by common carp.Crossref | GoogleScholarGoogle Scholar |
Ozimek, T, van Donk, E, and Gulati, RD (1993). Growth and nutrient uptake by two species of Elodea in experimental conditions and their role in nutrient accumulation in a macrophyte-dominated lake. Hydrobiologia 251, 13–18.
| Growth and nutrient uptake by two species of Elodea in experimental conditions and their role in nutrient accumulation in a macrophyte-dominated lake.Crossref | GoogleScholarGoogle Scholar |
Parkos, JJ, Santucci, VJ, and Wahl, DH (2003). Effects of adult common carp (Cyprinus carpio) on multiple trophic levels in shallow mesocosms. Canadian Journal of Fisheries and Aquatic Sciences 60, 182–192.
| Effects of adult common carp (Cyprinus carpio) on multiple trophic levels in shallow mesocosms.Crossref | GoogleScholarGoogle Scholar |
Pearson JB (2020) Carp, climate, and water quality: applying systems modeling to ecosystem recovery in Malheur Lake. PhD thesis, Oregon State University, Corvallis, OR, USA.
Pearson, JB, Dunham, JB, Bellmore, JR, and Lyons, D (2019). Modeling control of common carp (Cyprinus carpio) in a shallow lake–wetland system. Wetlands Ecology and Management 27, 663–682.
| Modeling control of common carp (Cyprinus carpio) in a shallow lake–wetland system.Crossref | GoogleScholarGoogle Scholar |
Pearson, JB, Bellmore, JR, and Dunham, JB (2022). Controlling invasive fish in fluctuating environments: model analysis of common carp (Cyprinus carpio) in a shallow lake. Ecoshpere 13, e3985.
| Controlling invasive fish in fluctuating environments: model analysis of common carp (Cyprinus carpio) in a shallow lake.Crossref | GoogleScholarGoogle Scholar |
Pine, WE, Martell, SJD, Walters, CJ, and Kitchell, JF (2009). Counterintuitive responses of fish populations to management actions. Fisheries 34, 165–180.
| Counterintuitive responses of fish populations to management actions.Crossref | GoogleScholarGoogle Scholar |
Qiu, X, Mei, X, Razlutskij, V, Rudstam, LG, Liu, Z, Tong, C, and Zhang, X (2019). Effects of common carp (Cyprinus carpio) on water quality in aquatic ecosystems dominated by submerged plants: a mesocosm study. Knowledge & Management of Aquatic Ecosystems 420, 28.
| Effects of common carp (Cyprinus carpio) on water quality in aquatic ecosystems dominated by submerged plants: a mesocosm study.Crossref | GoogleScholarGoogle Scholar |
Rice EB, Baird RB, Eaton AD, Clescerei LS (Eds) (2012) ‘Standard methods for the examination of water and wastewater’, 22nd edn. (American Public Health Association (APHA), American Water Works Association (AWWA), and Water Environment Federation (WEF): Washington, DC, USA)
Ritvo, G, Kochba, M, and Avnimelech, Y (2004). The effects of common carp bioturbation on fishpond bottom soil. Aquaculture 242, 345–356.
| The effects of common carp bioturbation on fishpond bottom soil.Crossref | GoogleScholarGoogle Scholar |
Roberts, J, Chick, A, Oswald, L, and Thompson, P (1995). Effect of carp, Cyprinus carpio L., an exotic benthivorous fish, on aquatic plants and water quality in experimental ponds. Marine and Freshwater Research 46, 1171–1180.
| Effect of carp, Cyprinus carpio L., an exotic benthivorous fish, on aquatic plants and water quality in experimental ponds.Crossref | GoogleScholarGoogle Scholar |
Rytwinski, T, Taylor, JJ, Donaldson, LA, Britton, JR, Browne, DR, Gresswell, RE, Lintermans, M, Prior, KA, Pellatt, MG, Vis, C, and Cooke, SJ (2019). The effectiveness of non-native fish removal techniques in freshwater ecosystems: a systematic review. Environmental Reviews 27, 71–94.
| The effectiveness of non-native fish removal techniques in freshwater ecosystems: a systematic review.Crossref | GoogleScholarGoogle Scholar |
Scheffer M (1998) ‘The ecology of shallow lakes.’ (Chapman and Hall: London, UK)
Scheffer, M, and Carpenter, SR (2003). Catastrophic regime shifts in ecosystems: linking theory to observation. Trends in Ecology & Evolution 18, 648–656.
| Catastrophic regime shifts in ecosystems: linking theory to observation.Crossref | GoogleScholarGoogle Scholar |
Scheffer, M, Hosper, SH, Meijer, M-L, Moss, B, and Jeppesen, E (1993). Alternative equilibria in shallow lakes. Trends in Ecology & Evolution 8, 275–279.
| Alternative equilibria in shallow lakes.Crossref | GoogleScholarGoogle Scholar |
Shields, JT (1958). Experimental control of carp reproduction through water drawdowns in Fort Randall Reservoir, South Dakota. Transactions of the American Fisheries Society 87, 23–33.
| Experimental control of carp reproduction through water drawdowns in Fort Randall Reservoir, South Dakota.Crossref | GoogleScholarGoogle Scholar |
Simpson, WG, Peterson, DP, Steinke, K, and Beck, L (2018). The efficacy of killing developing common carp embryos with electricity: using a laboratory evaluation to assess a potential means of reducing the recruitment of an invasive fish. Management of Biological Invasions 9, 279–290.
| The efficacy of killing developing common carp embryos with electricity: using a laboratory evaluation to assess a potential means of reducing the recruitment of an invasive fish.Crossref | GoogleScholarGoogle Scholar |
Stuart, IG, and Conallin, AJ (2018). Control of globally invasive common carp: an 11-year commercial trial of the Williams’ cage. North American Journal of Fisheries Management 38, 1160–1169.
| Control of globally invasive common carp: an 11-year commercial trial of the Williams’ cage.Crossref | GoogleScholarGoogle Scholar |
Taylor, AH, Tracey, SR, Hartmann, K, and Patil, JG (2012). Exploiting seasonal habitat use of the common carp, Cyprinus carpio, in a lacustrine system for management and eradication. Marine and Freshwater Research 63, 587–597.
| Exploiting seasonal habitat use of the common carp, Cyprinus carpio, in a lacustrine system for management and eradication.Crossref | GoogleScholarGoogle Scholar |
Thresher, RE, Hayes, K, Bax, NJ, Teem, J, Benfey, TJ, and Gould, F (2014). Genetic control of invasive fish: technological options and its role in integrated pest management. Biological Invasions 16, 1201–1216.
| Genetic control of invasive fish: technological options and its role in integrated pest management.Crossref | GoogleScholarGoogle Scholar |
Timms, RM, and Moss, B (1984). Prevention of growth of potentially dense phytoplankton populations by zooplankton grazing, in the presence of zooplanktivorous fish, in a shallow wetland ecosystem. Limnology and Oceanography 29, 472–486.
| Prevention of growth of potentially dense phytoplankton populations by zooplankton grazing, in the presence of zooplanktivorous fish, in a shallow wetland ecosystem.Crossref | GoogleScholarGoogle Scholar |
US Department of the Interior (2018) ‘Development of a framework applicable for managed wetlands: state-and-transition models for wetland habitat assessment and management – procedures manual’, 4th edn. (US https://doi.org/ Lima, MT, USA)
US Fish and Wildlife Service (2013) ‘Comprehensive conservation plan for Malheur National Wildlife Refuge.’ (USFWS: Princeton, OR, USA)
Use of Fishes in Research Committee (2014) ‘Guidelines for the use of fishes in research.’ (American Fisheries Society: Bethesda, MD, USA)
van Donk, E, and van de Bund, WJ (2002). Impact of submerged macrophytes including charophytes on phyto- and zooplankton communities: allelopathy versus other mechanisms. Aquatic Botany 72, 261–274.
| Impact of submerged macrophytes including charophytes on phyto- and zooplankton communities: allelopathy versus other mechanisms.Crossref | GoogleScholarGoogle Scholar |
Vilizzi, L, Thwaites, LA, Smith, BB, Nicol, JM, and Madden, CP (2014). Ecological effects of common carp (Cyprinus carpio) in a semi-arid floodplain wetland. Marine and Freshwater Research 65, 802–817.
| Ecological effects of common carp (Cyprinus carpio) in a semi-arid floodplain wetland.Crossref | GoogleScholarGoogle Scholar |
Vilizzi, L, Tarkan, AS, and Copp, GH (2015). Experimental evidence from causal criteria analysis for the effects of common carp Cyprinus carpio on freshwater ecosystems: a global perspective. Reviews in Fisheries Science & Aquaculture 23, 253–290.
| Experimental evidence from causal criteria analysis for the effects of common carp Cyprinus carpio on freshwater ecosystems: a global perspective.Crossref | GoogleScholarGoogle Scholar |
Weber, MJ, and Brown, ML (2009). Effects of common carp on aquatic ecosystems 80 years after “carp as a dominant”: ecological insights for fisheries management. Reviews in Fisheries Science 17, 524–537.
| Effects of common carp on aquatic ecosystems 80 years after “carp as a dominant”: ecological insights for fisheries management.Crossref | GoogleScholarGoogle Scholar |
Weber, MJ, Hennen, MJ, and Brown, ML (2011). Simulated population responses of common carp to commercial exploitation. North American Journal of Fisheries Management 31, 269–279.
| Simulated population responses of common carp to commercial exploitation.Crossref | GoogleScholarGoogle Scholar |
Weber, MJ, Hennen, MJ, Brown, ML, Lucchesi, DO, and St Sauver, TR (2016). Compensatory response of invasive common carp Cyprinus carpio to harvest. Fisheries Research 179, 168–178.
| Compensatory response of invasive common carp Cyprinus carpio to harvest.Crossref | GoogleScholarGoogle Scholar |
Werner, EE, and Anholt, BR (1993). Ecological consequences of the trade-off between growth and mortality rates mediated by foraging activity. The American Naturalist 142, 242–272.
| Ecological consequences of the trade-off between growth and mortality rates mediated by foraging activity.Crossref | GoogleScholarGoogle Scholar | 19425978PubMed |
Williams AE (2005) The theory of alternative stable states in shallow lake ecosystems. In ‘Water encyclopedia. Vol. 3’. (Eds JH Lehr, J Keeley) pp. 272–274. (Wiley: Hoboken, NJ, USA)
Wium-Andersen, S, Anthoni, U, Christophersen, C, and Houen, G (1982). Allelopathic effects on phytoplankton by substances isolated from aquatic macrophytes (Charales). Oikos 39, 187–190.
| Allelopathic effects on phytoplankton by substances isolated from aquatic macrophytes (Charales).Crossref | GoogleScholarGoogle Scholar |
Yick, JL, Wisniewski, C, Diggle, J, and Patil, JG (2021). Eradication of the invasive common carp, Cyprinus carpio from a large lake: lessons and insights from the Tasmanian experience. Fishes 6, 6.
| Eradication of the invasive common carp, Cyprinus carpio from a large lake: lessons and insights from the Tasmanian experience.Crossref | GoogleScholarGoogle Scholar |
Zambrano, L, and Hinojosa, D (1999). Direct and indirect effects of carp (Cyprinus carpio L.) on macrophyte and benthic communities in experimental shallow ponds in central Mexico. Hydrobiologia 408–409, 131–138.
| Direct and indirect effects of carp (Cyprinus carpio L.) on macrophyte and benthic communities in experimental shallow ponds in central Mexico.Crossref | GoogleScholarGoogle Scholar |
Zambrano, L, Scheffer, M, and Martínez-Ramos, M (2001). Catastrophic response of lakes to benthivorous fish introduction. Oikos 94, 344–350.
| Catastrophic response of lakes to benthivorous fish introduction.Crossref | GoogleScholarGoogle Scholar |
Zhang, X, Liu, Z, Gulati, RD, and Jeppesen, E (2013). The effect of benthic algae on phosphorus exchange between sediment and overlying water in shallow lakes: a microcosm study using 32P as a tracer. Hydrobiologia 710, 109–116.
| The effect of benthic algae on phosphorus exchange between sediment and overlying water in shallow lakes: a microcosm study using 32P as a tracer.Crossref | GoogleScholarGoogle Scholar |
1 Any use of trade, firm, or product names is for descriptive purposes only and does not imply endorsement by the US Government. The findings and conclusions in this article are those of the authors and do not necessarily represent the views of the US Fish and Wildlife Service.