Challenges to the design and testing of antimicrobial nanostructured surfaces
Denver Linklater A B C and Elena P. Ivanova A B *A School of Science, STEM College, RMIT University, Melbourne Vic. 3000, Australia.
B ARC Hub for Australian Steel Manufacturing, Wollongong, NSW, Australia.
C Department of Biomedical Engineering, The University of Melbourne, Parkville, Vic. 3010, Australia.
![]() Dr Denver Linklater is a McKenzie Postdoctoral Fellow in Biomedical Engineering at The University of Melbourne’s Faculty of Engineering and Information Technology. Her research interests are in design and synthesis of nanomaterials for novel anti-microbial technologies, stem cell culture and tissue re-generation. |
![]() Elena Ivanova is a Distinguished Professor of RMIT University. Her research interests are in design and fabrication of biomimetic antimicrobial micro- and nano-structured surfaces, materials biointerfaces and immobilisation of biomolecules and microorganisms in micro- and nano-environments. |
Microbiology Australia 44(2) 79-82 https://doi.org/10.1071/MA23023
Submitted: 31 March 2023 Accepted: 8 May 2023 Published: 26 May 2023
© 2023 The Author(s) (or their employer(s)). Published by CSIRO Publishing on behalf of the ASM. This is an open access article distributed under the Creative Commons Attribution-NonCommercial-NoDerivatives 4.0 International License (CC BY-NC-ND)
Abstract
Nanomaterials, specifically nano-topographies, have been explored for their antimicrobial activity toward bacteria, fungi and even viruses. A decade ago, we discovered that the nanopillar topography of insect wings such as cicadas, dragonflies and damselflies, were not repelling bacteria as previously surmised, but bacteria were attaching and consequently being killed. The nature of the bactericidal effect associated with nanostructured insect wings has been extended to include antimicrobial activity toward both to environmental and pathogenic fungi. Specifically, the antimicrobial nature is associated with the physical disintegration of attached microbes due to a mechanical stress imposed on the cell membrane, which stretches and breaks. This exciting new discovery implies that, if successfully replicated on the surface of biomaterials and implantable devices, systemic or local administration of antibiotics are no longer required to kill bacteria that attach on such surfaces.
Keywords: antibacterial surfaces, antimicrobial surfaces, biofilms, insect wing surfaces, printed antimicrobial surfaces.
Introduction
A new generation of antimicrobial materials
The engineering of materials at the nanoscale (<100 nm) often requires ultra-precision machining to take advantage of the specialised properties imparted to nanoscale materials relative to the bulk material.1–12 Enhanced optical, electrical and chemical reactivity are just some of the advantages of nanoscale materials. Although nanotechnology is a reasonably new field of science, historically, the nano-engineering of surfaces including thin film deposition and lithography processes, was an important advancement in the development of micro-electromechanical systems (MEMS) and computation.
In recent years, substantial research efforts have been employed to replicate the bactericidal patterns found in nature onto materials with broad commercial application and to upscale manufacturing of antimicrobial nanostructured topographies for an array of applications.11–16 As such, simple, facile methods for the large-scale fabrication of nanostructured topographies have taken centre stage for the development of mechano-bactericidal topographies. These nanofabrication technologies include wet and dry etching techniques, layer-by-layer assembly, electrochemical etching, embossing, among others.4,8 The nano-topographies achieved by these varied fabrication techniques often result in uncontrolled, self-organised structures such as pillars, spikes, pores, etc., where the features’ height and density can be tuned by etching time and etchant concentration.8 It is unlikely that high precision can be achieved using simple wet or dry etching and self-assembly techniques. For example, black silicon is a biomimetic nanopillar silicon topography inspired by the dragonfly wing surface topography that has been utilised heavily as a synthetic model to study the mechanism and efficacy of biomimetic nanostructures as antimicrobial surfaces.5–9,17,18 The maskless plasma etching of silicon creates a random organisation of high-aspect-ratio pillars that resembles the topography of dragonfly wings (Fig. 1). The surface is visibly black due to the light entrapping capability of the surface pillars, hence, black silicon. Although some black silicon patterns have achieved exceedingly high bactericidal efficiency,10 other, similar patterns with degrees of variation in the height, width, spacing or density of the nanofeatures have achieved varying antibacterial results.19,20 Despite a reduction in the bactericidal efficacy due to inherent pattern variation that result from facile fabrication approaches, we recently showed that mechanically injuring the cells that encounter the surface (although not killing them directly) may eventually lead to the programmed cell death of bacteria and fungi, regardless20 (Le et al., unpubl. data). This new finding is supported by other research groups.21 By contrast, highly regular, controlled nanofeatures that can be achieved using lithographic methods such as electron-beam, colloidal or nano-imprint lithography have been used to gain insight into the antimicrobial mechanism of nanostructured surfaces,1,2 but large-scale patterning remains out of reach.
Nanoscale topography of insect wing surfaces and their synthetic analogues. (a) Photograph of dragonfly wings and corresponding scanning electron microscopy (SEM) image of the wing surface nanotopography. (b) Graph of the dimensions (height, width and spacing) of different insect wing nanofeatures. (c) Graph showing the geometric parameters of bactericidal nanofeatures. (d–i) Representative SEM images of synthetic biomimetic mechano-bactericidal nanopatterns reported in the literature. Adapted with permission from Nature Publishing Group.4
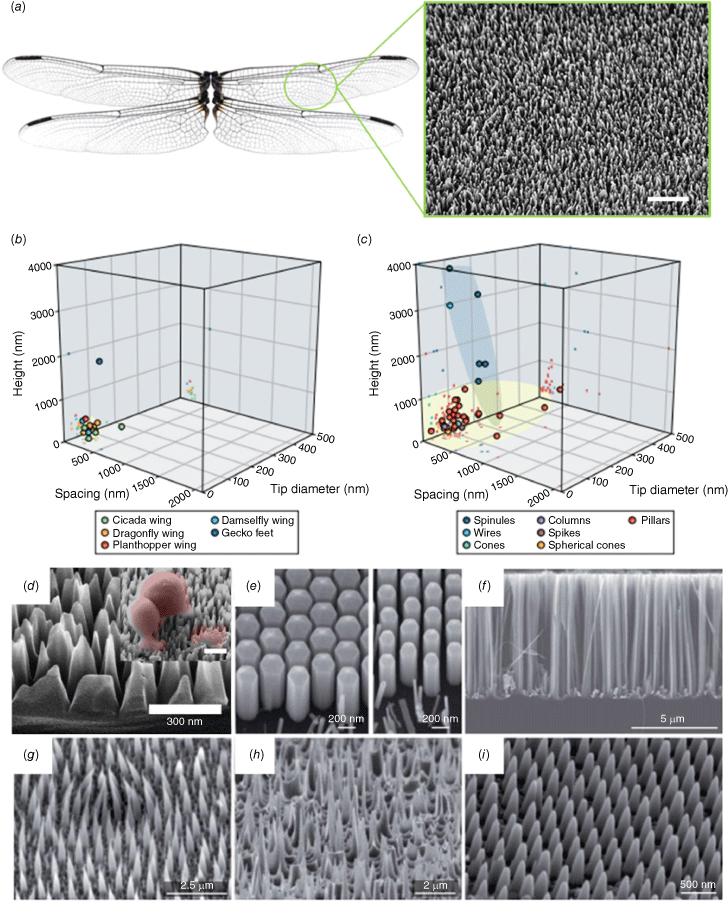
Characterisation of nanotopography and nanoarchitecture
Despite the increasing research efforts by different groups, there remains a lack of systematic and consistent analysis of surface characteristics, such as wettability, roughness, topography and architecture. A limited set of surface roughness parameters are commonly used to describe surface topography and architecture.22 Particularly, the arithmetical average height (Sa) and the root mean square of the height values (Sq) are common quantitative measures of surface roughness.23 For example, we recently investigated the influence of surface architecture on the attachment and morphogenesis of Candida cells.3 The Sa of polished titanium was in the same range as that of glass surfaces (10 nm), yet their surface nanoarchitecture differed drastically owing to differences in skewness and kurtosis values. These skewness and kurtosis values demonstrated that the surface nanoarchitecture contained sizable valleys that were favourable for Candida albicans attachment, whereas the surface profile of polished titanium revealed a spiky surface architecture, engendering low attachment density of fungal cells. In this vein, the standardisation of a set of experimental parameters to firstly, characterise nanopatterned surfaces and then to determine antimicrobial efficacy, and mechanism, of nanostructured surfaces is urgently required.
Thus, it has become clear that surface nanotopography and nanoarchitecture are keys to controlling the bactericidal efficacy; yet seemingly negligible changes in the pattern of disorganised features can lead to substantial changes in the rates of bacterial cell death.19 Research that has utilised random, uncontrolled nanofeatures to elucidate the mechanism of action of mechano-bactericidal surfaces may be susceptible to the incorrect interpretation of their data.24
Lack of standard approaches for testing antimicrobial properties of nanostructured surfaces
Unfortunately, as the material designs advance, it becomes more difficult to directly compare the bactericidal efficacy of dissimilar materials and surface patterns due to the lack of standard approaches for testing their performances. Therefore, a standard set of microbiological assays should be employed to assess the bactericidal efficiency of nanostructured surfaces. This will allow for comparison between the various respective works published. For example, two recent works assessing the role of nanopillar density on antibacterial performance of the nanostructured surface using patterns of similar nanopillar height and cap diameter achieved disparate results.25 The authors reported that the apparent discrepancy between the data could be due to the different experimental conditions used for viability assays, e.g. the use of different physiological buffer solutions. Changes to physicochemical conditions and ionic strength are known to have a significant effect on bacterial adhesion to solid substrata.23
In addition, whereas assays such as disc diffusion assays and zone of inhibition assays are common determinators of the antibacterial efficacy of antimicrobial compounds (antibiotics),26 they are not a good indicator of the bactericidal efficacy of nanostructured surfaces. Similarly, some groups report the use of spectroscopic means to determine bacterial growth in the presence of nanostructured surfaces; however, this method measures bacterial growth as a function of the turbidity of the bacterial suspension and does not discriminate between live, injured or dead cells, or surface attached cells. Preferably, direct visualisation of the surfaces by fluorescent labelling of attached cells and correlated with scanning electron microscopy (SEM), or transmission electron microscopy (TEM) would provide more accurate estimation of the ratio between live and dead surface attached cells. Standard plate count techniques can be used to provide quantification of the surface-attached non-viable cells as fluorescent labelling currently only allows for determination of membrane permeability (not cell viability directly). Since the materials kill or injure the cells upon contact, it is important to characterise cells that are both unattached (but may have encountered the surface) and attached on the surface.27 For example, for a given condition (such as measurement of the production of reactive oxygen species, membrane potential, cell respiration and more), the flow cytometry method enables high-throughput screening of bactericidal surface designs (with the ability to rapidly monitor killing rates and trajectories).20,27 The only down-side being that the removal of surface-attached bacteria should be done in the gentlest manner so as not to distress the cells. Mechanistic insights into the bactericidal action of various nanofeatures cannot be defined from SEM imaging only but higher resolution techniques that allow for visualisation of the cell–nanofeature interface or correlative microscopy techniques should be utilised. As such, the question of the role of external forces, such capillary forces, in the antibacterial action of nanostructured surfaces has arisen.28 Therefore, preparation of the surfaces for imaging should be done carefully to avoid the development of an air–liquid interface, resulting in capillary forces acting on the sample as it dehydrates.
Current interpretations of bactericidal mechanisms
The mechanisms of bactericidal action of nanostructured topographies has become hotly contested, despite robust research showing that bacteria die as a result of the stretching, and subsequent rupture, of the bacterial membrane upon surface adhesion.17 Some groups hypothesised the tearing of the bacterial membrane as the bacteria attempt to move on the nanopillar substrate9; others proposed that the bacterial membrane can be penetrated by sharp nanospikes.24 Indeed, the antibacterial mechanism of different nanopatterned topographies is multifaceted and the methods by which they achieve the physical rapturing of bacterial cells has been shown to be unique depending on the nanopattern.2,6
A thorough examination of the interactions between bacteria and carefully designed antibacterial nanoprotrusions is necessary for engineering surface topographies that exhibit close to 100% of antimicrobial efficacy. This can be achieved using multiple analytical approaches combining direct and indirect measurement techniques of bacterial cell health. There is an inherent danger to upscaling and commercialisation of surface patterns that exhibit low-to-medium antibacterial activity as it is not currently known whether bacteria can develop resistance mechanisms to mechanical disruption. In this vein, there is rapidly growing interest in the development of hybrid nanostructured surfaces that combine a bactericidal nanopattern with a surface-active antibacterial compound, such as metal ions, antimicrobial peptides and quaternary ammonium compounds.29 However, since bacteria can develop resistance mechanisms so readily to antimicrobial compounds there is also the risk of increasing bacterial resistance to common antimicrobial compounds if multifunctional nanostructured surfaces are not capable of achieving 100% bactericidal efficacy. Nevertheless, in light of the ever-growing rates of antibiotic resistance, nanostructured surfaces represent an exciting new development for the design and manufacture of anti-infective implantable devices.
Data availability
Data sharing is not applicable as no new data were generated or analysed during this study.
Declaration of funding
Funding from the Australian Research Council (ARC) Industrial Transformation Research Hubs Scheme (ARC Research Hub for Australian Steel, Manufacturing, Project Number IH130100017) and ARC893 Industrial Transformation Training Centre (ITTC) scheme (Project Number IC180100005) is gratefully acknowledged.
References
[1] Linklater, DP and Ivanova, EP (2022) Nanostructured antibacterial surfaces – what can be achieved? Nano Today 43, 101404.| Nanostructured antibacterial surfaces – what can be achieved?Crossref | GoogleScholarGoogle Scholar |
[2] Ivanova, EP et al. (2020) The multi-faceted mechano-bactericidal mechanism of nanostructured surfaces. Proc Natl Acad Sci USA 117, 12598–12605.
| The multi-faceted mechano-bactericidal mechanism of nanostructured surfaces.Crossref | GoogleScholarGoogle Scholar |
[3] Le, PH et al. (2020) Nanoscale surface roughness influences Candida albicans biofilm formation. ACS Appl Bio Mater 3, 8581–8591.
| Nanoscale surface roughness influences Candida albicans biofilm formation.Crossref | GoogleScholarGoogle Scholar |
[4] Linklater, DP et al. (2020) Mechano-bactericidal actions of nanostructured surfaces. Nat Rev Microbiol 19, 8–22.
| Mechano-bactericidal actions of nanostructured surfaces.Crossref | GoogleScholarGoogle Scholar |
[5] Linklater, DP et al. (2018) Mechano-bactericidal mechanism of graphene nanomaterials. Interface Focus 8, 20170060.
| Mechano-bactericidal mechanism of graphene nanomaterials.Crossref | GoogleScholarGoogle Scholar |
[6] Linklater, DP et al. (2018) High aspect ratio nanostructures kill bacteria via storage and release of mechanical energy. ACS Nano 12, 6657–6667.
| High aspect ratio nanostructures kill bacteria via storage and release of mechanical energy.Crossref | GoogleScholarGoogle Scholar |
[7] Linklater, DP et al. (2019) Mechanical inactivation of Staphylococcus aureus and Pseudomonas aeruginosa by titanium substrata with hierarchical surface structures. Materialia 5, 100197.
| Mechanical inactivation of Staphylococcus aureus and Pseudomonas aeruginosa by titanium substrata with hierarchical surface structures.Crossref | GoogleScholarGoogle Scholar |
[8] Linklater, DP et al. (2017) Nanofabrication of mechano-bactericidal surfaces. Nanoscale 9, 16564–16585.
| Nanofabrication of mechano-bactericidal surfaces.Crossref | GoogleScholarGoogle Scholar |
[9] Linklater, DP et al. (2017) Comment on “Bactericidal effects of natural nanotopography of dragonfly wing on Escherichia coli”. ACS Appl Mater Interfaces 9, 29387–29393.
| Comment on “Bactericidal effects of natural nanotopography of dragonfly wing on Escherichia coli”.Crossref | GoogleScholarGoogle Scholar |
[10] Linklater, DP et al. (2017) Influence of nanoscale topology on bactericidal efficiency of black silicon surfaces. Nanotechnology 28, 245301.
| Influence of nanoscale topology on bactericidal efficiency of black silicon surfaces.Crossref | GoogleScholarGoogle Scholar |
[11] Linklater, DP et al. (2022) Nanopillar polymer films as antibacterial packaging materials. ACS Appl Nano Mater 5, 2578–2591.
| Nanopillar polymer films as antibacterial packaging materials.Crossref | GoogleScholarGoogle Scholar |
[12] Wandiyanto, JV et al. (2020) Tunable morphological changes of asymmetric titanium nanosheets with bactericidal properties. J Colloid Interface Sci 560, 572–580.
| Tunable morphological changes of asymmetric titanium nanosheets with bactericidal properties.Crossref | GoogleScholarGoogle Scholar |
[13] Ivanova, EP et al. (2012) Natural bactericidal surfaces: mechanical rupture of Pseudomonas aeruginosa cells by cicada wings. Small 8, 2489–2494.
| Natural bactericidal surfaces: mechanical rupture of Pseudomonas aeruginosa cells by cicada wings.Crossref | GoogleScholarGoogle Scholar |
[14] Linklater, DP et al. (2023) Biomimetic nanopillar silicon surfaces rupture fungal spores. Int J Mol Sci 24, 1298.
| Biomimetic nanopillar silicon surfaces rupture fungal spores.Crossref | GoogleScholarGoogle Scholar |
[15] Ivanova, EP et al. (2021) Antifungal versus antibacterial defence of insect wings. J Colloid Interface Sci 603, 886–897.
| Antifungal versus antibacterial defence of insect wings.Crossref | GoogleScholarGoogle Scholar |
[16] Clainche, TL et al. (2020) Mechano-bactericidal titanium surfaces for bone tissue engineering. ACS Appl Mater Interfaces 12, 48272–48283.
| Mechano-bactericidal titanium surfaces for bone tissue engineering.Crossref | GoogleScholarGoogle Scholar |
[17] Pogodin, S et al. (2013) Biophysical model of bacterial cell interactions with nanopatterned cicada wing surfaces. Biophys J 104, 835–840.
| Biophysical model of bacterial cell interactions with nanopatterned cicada wing surfaces.Crossref | GoogleScholarGoogle Scholar |
[18] Hasan, J et al. (2013) Antibacterial surfaces: the quest for a new generation of biomaterials. Trends Biotechnol 31, 295–304.
| Antibacterial surfaces: the quest for a new generation of biomaterials.Crossref | GoogleScholarGoogle Scholar |
[19] Bhadra, CM et al. (2018) Subtle variations in surface properties of black silicon surfaces influence the degree of bactericidal efficiency. Nanomicro Lett 10, 36.
| Subtle variations in surface properties of black silicon surfaces influence the degree of bactericidal efficiency.Crossref | GoogleScholarGoogle Scholar |
[20] Zhao, S et al. (2022) Programmed death of injured Pseudomonas aeruginosa on mechano-bactericidal surfaces. Nano Lett 22, 1129–1137.
| Programmed death of injured Pseudomonas aeruginosa on mechano-bactericidal surfaces.Crossref | GoogleScholarGoogle Scholar |
[21] Daimon, M et al. (2023) Fluorescence intensity of liposomes and E. coli attached to nanopillar arrays: implications for bacterial death on nanostructures. ACS Appl Nano Mater 6, 1610–1619.
| Fluorescence intensity of liposomes and E. coli attached to nanopillar arrays: implications for bacterial death on nanostructures.Crossref | GoogleScholarGoogle Scholar |
[22] Wenzel, RN (1949) Surface roughness and contact angle. J Phys Colloid Chem 53, 1466–1467.
| Surface roughness and contact angle.Crossref | GoogleScholarGoogle Scholar |
[23] Crawford, RJ et al. (2012) Surface topographical factors influencing bacterial attachment. Adv Colloid Interface Sci 179–182, 142–149.
| Surface topographical factors influencing bacterial attachment.Crossref | GoogleScholarGoogle Scholar |
[24] Michalska, M et al. (2018) Tuning antimicrobial properties of biomimetic nanopatterned surfaces. Nanoscale 10, 6639–6650.
| Tuning antimicrobial properties of biomimetic nanopatterned surfaces.Crossref | GoogleScholarGoogle Scholar |
[25] Ishak, MI et al. (2021) Insights into complex nanopillar–bacteria interactions: roles of nanotopography and bacterial surface proteins. J Colloid Interface Sci 604, 91–103.
| Insights into complex nanopillar–bacteria interactions: roles of nanotopography and bacterial surface proteins.Crossref | GoogleScholarGoogle Scholar |
[26] Wang, M et al. (2015) Influence of surface chemistry on the release of an antibacterial drug from nanostructured porous silicon. Langmuir 31, 6179–6185.
| Influence of surface chemistry on the release of an antibacterial drug from nanostructured porous silicon.Crossref | GoogleScholarGoogle Scholar |
[27] Michalska, M et al. (2021) Antimicrobial properties of nanostructured surfaces – demonstrating the need for a standard testing methodology. Nanoscale 13, 17603–17614.
| Antimicrobial properties of nanostructured surfaces – demonstrating the need for a standard testing methodology.Crossref | GoogleScholarGoogle Scholar |
[28] Valiei, A et al. (2020) Hydrophilic mechano-bactericidal nanopillars require external forces to rapidly kill bacteria. Nano Lett 20, 5720–5727.
| Hydrophilic mechano-bactericidal nanopillars require external forces to rapidly kill bacteria.Crossref | GoogleScholarGoogle Scholar |
[29] Maher, S et al. (2022) Advancing of 3D-printed titanium implants with combined antibacterial protection using ultrasharp nanostructured surface and gallium-releasing agents. ACS Biomater Sci Eng 8, 314–327.
| Advancing of 3D-printed titanium implants with combined antibacterial protection using ultrasharp nanostructured surface and gallium-releasing agents.Crossref | GoogleScholarGoogle Scholar |