Viewing biofilm formation through a multifocal lens of physics and biology
Binu Kundukad A # , James C. S. Ho A B # , Sudarsan Mugunthan A , Lan Li Wong A , Scott A. Rice C , Atul N. Parikh A B D , Thomas Seviour A E , Jamie Hinks H and Staffan Kjelleberg A F G *A Singapore Centre for Environmental Life Sciences Engineering, Nanyang Technological University, Singapore.
B Institute for Digital Molecular Analytics and Science, Nanyang Technological University, Singapore.
C CSIRO, Agriculture and Food, Microbiomes for One Systems Health, Canberra, ACT, Australia.
D Department of Biomedical Engineering, University of California, Davis, CA, USA.
E WATEC Aarhus University Centre for Water Technology, Aarhus, Denmark.
F School of Biological Sciences, Nanyang Technological University, Singapore.
G School of Biological, Earth and Environmental Sciences, University of New South Wales, NSW, Australia.
H Deceased. Formerly at Singapore Centre for Environmental Life Sciences Engineering, Nanyang Technological University, Singapore.
![]() Binu Kundukad is a senior research fellow at the Singapore Centre for Environmental Life Sciences Engineering (SCELSE), Nanyang Technological University, Singapore. With a background in physics, her research interest is in developing and using biophysical tools and methods to understand biological problems. She is particularly interested in understanding how the mechanical properties of biofilm matrix, such as viscosity and elasticity, influence bacterial behaviour and biofilm formation. |
![]() James C. S. Ho is a senior research fellow at SCELSE. His research focuses on the assembly of functional, multi-length scale biomolecular complexes and using them to understand complex biological and biophysical processes. |
![]() Sudarsan Mugunthan is a graduate student at SCELSE. His research focuses on the role of extracellular DNA/RNA in Pseudomonas biofilms and the regulation of eDNA and eRNA secretion. |
![]() Lan Li Wong is a graduate student at SCELSE. She works on structure–function characterisation of extracellular polymeric substances forming anammox granule biofilms, with a focus on surface layer proteins. |
![]() Scott A. Rice is the director of Microbiomes for One Systems Health at CSIRO, Australia. His research focuses on microbial cell–cell interactions and mechanisms of adaptation to environmental conditions. The goal has been to develop a deep understanding of the mechanisms by which bacteria mediate these responses and the impacts of those responses on the behaviour and function of bacteria. |
![]() Atul N. Parikh is a professor at the University of California, Davis. He is also serving as a Visiting Professor at SCELSE. His current research includes fundamental studies of dynamic self-assembly, active interfaces, and physical compartmentalisation in soft and living material systems. |
![]() Thomas Seviour is an associate professor at the Aarhus University, Denmark. His research aims to elucidate structure–function relationships of microbial biointerfaces in biofilms and bioprocesses of industrial and environmental significance. This understanding will lead to more precise biofilm and bioprocess control strategies, and may inform new approaches towards resource recovery. |
![]() Jamie Hinks was a senior principal research fellow at SCELSE. Jamie was an environmental microbiologist. He was interested in how small molecules, either native or exogenous, interact with the microbial envelope and the reactions these mediate. |
![]() Staffan Kjelleberg is an adviser to SCELSE and Distinguished University Professor at NTU. He has made major contributions to microbial ecology. His studies on bacterial adaptive responses and biofilm biology have received strong international recognition and have illuminated biofilm as the predominant mode of bacterial life in the environment. |
Microbiology Australia 44(2) 69-74 https://doi.org/10.1071/MA23021
Submitted: 22 March 2023 Accepted: 9 May 2023 Published: 24 May 2023
© 2023 The Author(s) (or their employer(s)). Published by CSIRO Publishing on behalf of the ASM. This is an open access article distributed under the Creative Commons Attribution-NonCommercial-NoDerivatives 4.0 International License (CC BY-NC-ND)
Abstract
Recent studies on the formation, organisation and dynamics of biofilms highlight the interplay between physical forces and biological programs. Two complementary generalised pathways that explain the mechanisms driving biofilm formation have emerged. In the first pathway, where physical forces precede the biological program, the initial expansion of cells leads to cell clustering or aggregation prior to the production of extracellular polymeric substances (EPS). The second pathway describes an initial biologically prompted production of EPS, which introduces new biophysical interactions within the EPS, such as by phase separation, macromolecular crowding, excluded volume interactions and intermolecular cross-linking. In practice, which of the two pathways is adopted is ultimately determined by the specificities of the biofilm and the local microenvironment, each leading to the formation of robust, viscoelastic biofilm. Within this framework, we further highlight here recent findings on the role of higher-order structures in matrix gelation and phase separation of EPS in promoting the clustering of bacteria. We assert that examining biofilms through the combined lens of physics and biology promises new and significant methodological and conceptual advancements in our understanding of biofilms.
Keywords: biofilms, EPS, extracellular polymeric substances, matrix, phase separation, viscoelasticity.
To survive and thrive in disparate environmental conditions, microorganisms adopt a biofilm lifestyle in which aggregates of microorganisms are embedded within a three-dimensional matrix of self-secreted biopolymers, collectively termed extracellular polymeric substances (EPS).1,2 This contrasts with the free-swimming planktonic lifestyle, where each microbial cell exists as an isolated entity. The biofilm lifestyle is highly pervasive and adopted by a variety of microorganisms in a broad diversity of habitats and environments. They span from anaerobic ammonium oxidation (anammox) bacteria forming beneficial polymicrobial biofilms that account for substantial nitrogen recycling in water bodies and global nitrogen sinks,3 to Pseudomonas aeruginosa cells co-existing with Staphylococcus aureus in the lungs of people with cystic fibrosis, giving rise to pathogenic biofilms that are mechanically robust and hard to eradicate.4
The initiation of the biofilm lifestyle is orchestrated by a complex set of tightly regulated biological mechanisms – including genetic program, gene regulation and post-translational modifications – as the cells respond to external cues (e.g. temperature, pH, osmolarity, nutrient availability, chemicals and attachment surfaces).2 The secreted biofilm matrix comprises a cocktail of chemically and structurally distinct EPS molecules (mainly polysaccharides, proteins, nucleic acid and lipids),5 whose interactions with each other are also influenced by environmental factors. This amounts to physically distinct and locally different environments around clusters of cells, which in turn exert emergent physicochemical forces on the embedded microorganisms, thereby forming a well-regulated, homeostatic feedback control system. Thus, biological and physical forces work together iteratively and synergistically to provide the matrix with the emergent physical, chemical and functional properties that set biofilm bacteria apart from their planktonic counterparts.6 The overall fitness of the embedded microorganisms is enhanced through emergent behaviours, which include efficient resource capture and retention, synergism of consortia and stress tolerance, as well as enhanced resistance to antimicrobial agents.2,7 Many of these emergent properties are attributed to the manipulation of the spatial organisation of matrix components that affect biopolymer interactions in the matrix and alter biofilm viscoelasticity.8
Understanding how this physical–biological interplay within the matrix provides biofilms with their emergent properties requires a cross-disciplinary approach that goes beyond classical microbiological methods. Quantitative biophysical approaches, when paired with qualitative biological observations, enable nuances of the underlying mechanisms to be described and contribute to establishing a holistic view of the biofilm. Recent studies are beginning to shed light on the importance of this interplay for establishing the emergent properties of biofilms.9–11 Here, we provide a selection of examples that highlight how biophysics can be used to explain certain phenomena in biofilms and how insights into the physical factors influencing biofilm formation can be exploited to understand and engineer biofilm emergent properties and functionalities.
Biofilm formation – an interplay between biology and physics
A key definition in the adoption of the biofilm lifestyle is the transition of the initially soluble bacterial biopolymers into an insoluble, gel-like state, which acts to immobilise the bacterial cells and generate interactive bacterial consortia. This transformation is mediated by the secreted EPS molecules that provide a distinct habitat for the bacteria, create a localised scaffold and establish emergent properties. Therefore, understanding how the EPS contributes to the formation of this physically distinct environment is the first step towards identifying the mechanisms of the unique biofilm emergent properties. The view of biofilm formation through the lens of biophysics has thus identified at least three fundamental biophysical concepts that contribute to this process (Fig. 1). These are (i) aggregation of bacteria due to reduced bacterial motility,11,12 (ii) reorganisation of the matrix by phase separation13 and (iii) gelation of the matrix biopolymers.10,14
Two proposed mechanisms of biofilm formation. Upper boxes (physics drives biology): a motility-induced transition driven by fluctuations in cell densities produces larger aggregates of immobile cells and highly motile smaller aggregates of swarming cells (red arrows). The high-density clusters then initiate EPS production and biofilm formation. Lower boxes (biology drives physics): the production of EPS is first activated by environmental cues. Cell–cell adhesion and biofilm formation are facilitated by the EPS. At low concentrations of EPS, where there is no crosslinking, the biofilms have a viscous behaviour. The crosslinking density increases with elevated EPS production, leading to an elastic biofilm matrix. The image is from Wong et al. with modification6 (© 2022, Wiley).
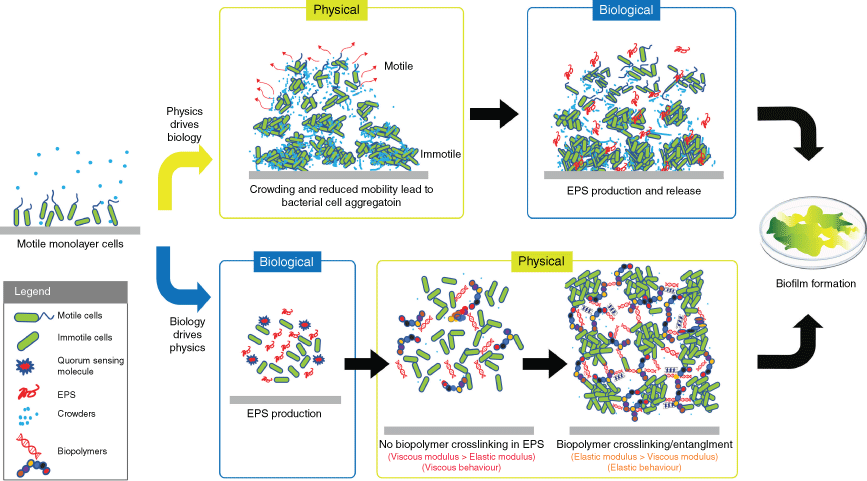
Aggregation of bacteria due to reduced bacterial motility
As bacteria multiply, motile cells move collectively in dynamic clusters, following two scenarios.11 In the first scenario, cell crowding precedes EPS production. Fluctuations in cell density randomly produce high-cell-density clusters in which movement of the cells is slowed down due to space constraints (crowding). Subsequent cell division increases crowding, leading to a further limitation in cell movement, generating areas with high-density clusters comprising immobilised cells, and areas with low-density of swarming cells. This scenario is thought to activate biological programs that produce EPS, which drives the progression to a mature biofilm state. In the second scenario, EPS production precedes the clustering of bacteria. The production of EPS (e.g. quorum sensing activates exopolysaccharide succinoglycan in Sinorhizobium meliloti) crowds the space around the bacterial cells and physically pushes them to form high-density clusters, in order to increase their own entropy.15,16 Both motile and immotile bacteria aggregate in response to the increase in macromolecule concentrations.
Iceberg perspective of the complex properties of biofilms. The study of biofilms requires a cross-disciplinary approach and collaborative effort to uncover new frontiers. The interface between biology and physics is highlighted in this perspective, with several examples of emerging biofilm formation mechanisms that connect the two inter-dependent forces, building on established knowledge of mechanisms. Methodological and conceptual advancements will inevitably change in the coming years, with progress in examining biofilms through the lenses of both physics and biology.
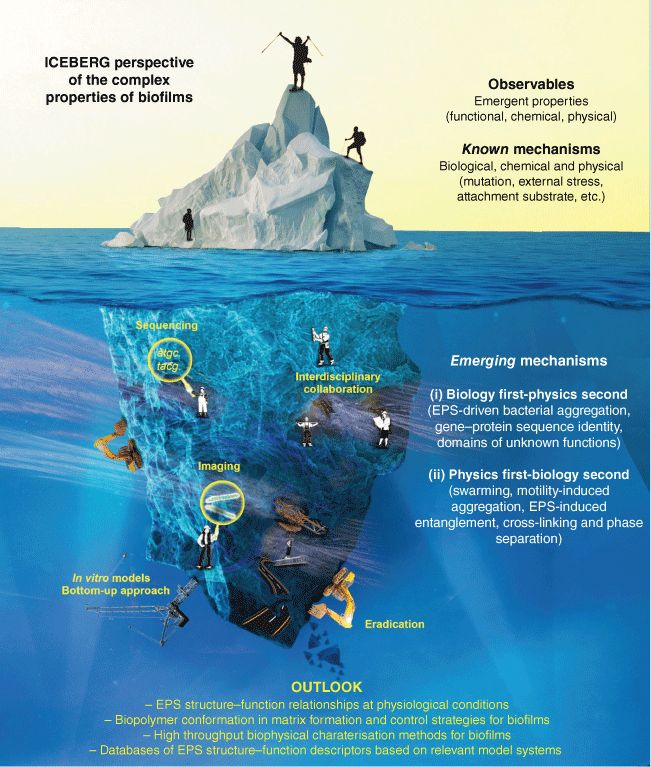
Reorganisation of the matrix by phase separation
Continued production of EPS crowds the biofilm matrix, where the biomolecules engage in physical interactions, and reorganise the matrix into co-existing phases (liquid–liquid phase separation, LLPS, or liquid–solid phase separation, LSPS).17 These phase behaviours are also commonly found in eukaryotic cell biology,18 suggesting it is a highly conserved and ancient biological process. For example, the amyotrophic lateral sclerosis (ALS)-associated FUS protein undergoes a transition into solid aggregates through an intermediate liquid droplet state.19 This transition to solid state is driven by the changes in the concentration of proteins in the cell. It is thought to be a key step in the progression of ALS pathology, as these aggregates can disrupt normal cellular processes. Similarly, in biofilms, the extracellular matrix produced by bacteria may go through an intermediate LLPS state before transitioning into a gel-like state.
A commonality among LLPS-inducing molecules is the presence of low-complexity, primary repeat sequences that adopt disordered conformations, which now appear to be an evolutionarily conserved process.20 Many of the proteins in biofilm matrices are also characterised by such sequences and behaviour, suggesting that LLPS-inducing biofilm proteins may have a functionally important role in driving biofilm formation. Some examples of proteins that promote biofilm formation include the biofilm-associated protein (BAP)21 in S. aureus and curli22 in Escherichia coli, which adopt amyloid-like structures and function as matrix scaffold biopolymers. In another example, the S-layer protein of nitrogen cycling wastewater bacteria form droplets that promote wetting and clustering of cells,13,23 in addition to its native function as self-assembled paracrystalline 2-D lattice on the bacterial surface. The latter example suggests that the S-layer protein is expressed extracellularly for cell–cell adhesion, microcolony formation and matrix assembly, using liquid droplets as an intermediate for biofilm formation.
Gelation of the matrix biopolymers
The material composition of the EPS, as mixtures of high molecular weight biopolymers at high concentrations, seeds conditions propitious for the formation of the gel-like EPS matrix. Matrix biopolymers can become physically entangled beyond a threshold concentration, or chemically crosslinked through non-covalent linkages.24,25 These crosslinks and entanglements result in a mechanically robust viscoelastic matrix, i.e. one having both viscous (flow-like) and elastic (solid-like) properties. These allow the microorganisms to withstand external physical stresses through the distinct organisation or reorganisation of the biofilm structure.8 In biofilms, the degree of crosslinking between the polymers of the matrix governs the viscoelasticity and can be quantified in physical terms such as elastic storage (G′) and viscous loss (G″) moduli. These physical properties yield critical insights into the mechanical behaviour of the biofilms and how they respond to the environment.
This is best exemplified by comparing mature and nascent biofilms. Mature biofilm matrices have higher crosslinking densities (larger elastic modulus) than their immature counterparts26 (Fig. 1). Crosslinking can either reflect a concentration-dependent physical entanglement or chemical crosslinking. For example, at sufficiently high concentrations, the Pel exopolysaccharide crosslinks with eDNA in P. aeruginosa biofilms,27,28 whereas the polysaccharide intercellular adhesins self-assemble by associative interactions below the entanglement threshold concentration in Staphylococcus epidermis biofilms.14 Structural exopolysaccharides modulate the viscoelastic properties of the matrix, offering different functionalities. For example, Pel confers malleability (characterised by smaller elastic moduli) to the matrix, allowing spreading and streamer formation, whereas Psl confers stiffness (larger elastic moduli) to the matrix allowing lateral biofilm growth.29 Apart from these physical interactions, some matrix components, such as eDNA, undergo a transition into higher-order structures (e.g. G-quadruplex and Holliday junctions10), facilitating gelation. In this way, the nucleotide structure plays an important role in G-quadruplex formation, suggesting that the establishment of these higher-order matrix structures is part of the fundamental genetic code. Filamentous Pf bacteriophage promote self-assembly of P. aeruginosa matrix biopolymer into liquid crystals, thereby enhancing biofilm functions such as adhesion, moisture retention and antibiotic tolerance.16 Filamentation of cells provides additional protection to biofilm bacteria and is a serious challenge in treating clinical infections.30 Filamentous hyphae may significantly enhance the mechanical strength of fungal biofilms as compared to bacterial biofilms.31
Taken together, the examples above illustrate how physical properties of the biofilm matrix play important roles in determining their functions, now a rapidly expanding area of biofilm research. Apart from the biophysical processes discussed here, other physical properties such as ecomechanics2 and electrogenic32 properties also play a role in shaping different biofilms. We assert that a thorough understanding of the role of physics in determining the emergent properties and functions of the matrix will yield a comprehensive understanding of biofilm formation, growth, and homeostasis.
Reflection and outlook
Although the emergent biofilm properties are potential targets for controlling and engineering biofilms, the principles and origins of these unique biofilm behaviours remain largely unknown (Fig. 2). Notably, the biological code for the emergent properties could be embedded in the chemical structures of EPS and the corresponding higher-order structures. However, to decipher these codes requires a holistic understanding of EPS structure–function relationships under physiological conditions that reflect biofilm microenvironments (e.g. crowding), and the means by which microorganisms utilise EPS to regulate and shape their local environments.
The conformation of matrix biopolymers is an important factor that facilitates crosslinking and entanglements in matrix formation. For example, DNA undergoes conformational changes to facilitate protein binding. The way these molecules interact with one another is critical to biofilm formation and stability. Methods to detect conformational changes are yet to be achieved as a key step towards understanding matrix formation and enabling matrix behaviour to be modelled. Understanding the role of biopolymer conformation is not just a matter of scientific curiosity, but has implications for the development of strategies for controlling biofilm growth in preventing associated challenges such as infection and biofouling or promoting biofilms for bioremediation and wastewater treatment.
Although resolving biological behaviour through next-generation sequencing is fast and reliable across biofilms in a range of contexts, there are no high-throughput approaches to characterise the exopolysaccharides, owing to their diversity and complex stereochemistry. Nonetheless, with technological advances, the ability to readily characterise biophysical properties will inevitably be realised in the coming years, thus enabling biofilms to be examined through the lenses of both physics and biology. In the meantime, it is important to identify suitable model systems that ideally represent ‘typical’ matrix structures and processes. Such model systems can form the foundation of holistic databases of EPS structure–function traits, which incorporate the principles of biology, physics and chemistry.
Acknowledgements
This article is dedicated to the memory of Dr Jamie Hinks, a key member of SCELSE, a colleague and a friend.
References
[1] Flemming, H-C et al. (2022) The biofilm matrix: multitasking in a shared space. Nat Rev Microbiol 21, 70–86.| The biofilm matrix: multitasking in a shared space.Crossref | GoogleScholarGoogle Scholar |
[2] Flemming, H-C et al. (2016) Biofilms: an emergent form of bacterial life. Nat Rev Microbiol 14, 563–575.
| Biofilms: an emergent form of bacterial life.Crossref | GoogleScholarGoogle Scholar |
[3] Arrigo, KR (2005) Marine microorganisms and global nutrient cycles. Nature 437, 349–355.
| Marine microorganisms and global nutrient cycles.Crossref | GoogleScholarGoogle Scholar |
[4] Yung, DBY et al. (2021) Friends or enemies? The complicated relationship between Pseudomonas aeruginosa and Staphylococcus aureus. Mol Microbiol 116, 1–15.
| Friends or enemies? The complicated relationship between Pseudomonas aeruginosa and Staphylococcus aureus.Crossref | GoogleScholarGoogle Scholar |
[5] Karygianni, L et al. (2020) Biofilm matrixome: extracellular components in structured microbial communities. Trends Microbiol 28, 668–681.
| Biofilm matrixome: extracellular components in structured microbial communities.Crossref | GoogleScholarGoogle Scholar |
[6] Wong, LL et al. (2023) Microbial biofilms are shaped by the constant dialogue between biological and physical forces in the extracellular matrix. Environ Microbiol 25, 199–208.
| Microbial biofilms are shaped by the constant dialogue between biological and physical forces in the extracellular matrix.Crossref | GoogleScholarGoogle Scholar |
[7] Costerton, JW et al. (1995) Microbial biofilms. Annu Rev Microbiol 49, 711–745.
| Microbial biofilms.Crossref | GoogleScholarGoogle Scholar |
[8] Peterson, BW et al. (2015) Viscoelasticity of biofilms and their recalcitrance to mechanical and chemical challenges. FEMS Microbiol Rev 39, 234–245.
| Viscoelasticity of biofilms and their recalcitrance to mechanical and chemical challenges.Crossref | GoogleScholarGoogle Scholar |
[9] Ren, Z et al. (2022) Interkingdom assemblages in human saliva display group-level surface mobility and disease-promoting emergent functions. Proc Natl Acad Sci USA 119, e2209699119.
| Interkingdom assemblages in human saliva display group-level surface mobility and disease-promoting emergent functions.Crossref | GoogleScholarGoogle Scholar |
[10] Seviour, T et al. (2021) The biofilm matrix scaffold of Pseudomonas aeruginosa contains G-quadruplex extracellular DNA structures. NPJ Biofilms Microbiomes 7, 27.
| The biofilm matrix scaffold of Pseudomonas aeruginosa contains G-quadruplex extracellular DNA structures.Crossref | GoogleScholarGoogle Scholar |
[11] Grobas, I et al. (2021) Swarming bacteria undergo localized dynamic phase transition to form stress-induced biofilms. eLife 10, e62632.
| Swarming bacteria undergo localized dynamic phase transition to form stress-induced biofilms.Crossref | GoogleScholarGoogle Scholar |
[12] Srinivasan, S et al. (2019) A multiphase theory for spreading microbial swarms and films. eLife 8, e42697.
| A multiphase theory for spreading microbial swarms and films.Crossref | GoogleScholarGoogle Scholar |
[13] Seviour, T et al. (2020) Phase transitions by an abundant protein in the anammox extracellular matrix mediate cell-to-cell aggregation and biofilm formation. mBio 11, e02052-20.
| Phase transitions by an abundant protein in the anammox extracellular matrix mediate cell-to-cell aggregation and biofilm formation.Crossref | GoogleScholarGoogle Scholar |
[14] Ganesan, M et al. (2016) Associative and entanglement contributions to the solution rheology of a bacterial polysaccharide. Macromolecules 49, 8313–8321.
| Associative and entanglement contributions to the solution rheology of a bacterial polysaccharide.Crossref | GoogleScholarGoogle Scholar |
[15] Ghosh, P et al. (2015) Mechanically driven phase separation in a growing bacterial colony. Proc Natl Acad Sci USA 112, E2166–E2173.
| Mechanically driven phase separation in a growing bacterial colony.Crossref | GoogleScholarGoogle Scholar |
[16] Secor, PR et al. (2015) Filamentous bacteriophage promote biofilm assembly and function. Cell Host Microbe 18, 549–559.
| Filamentous bacteriophage promote biofilm assembly and function.Crossref | GoogleScholarGoogle Scholar |
[17] Brangwynne, CP et al. (2015) Polymer physics of intracellular phase transitions. Nat Phys 11, 899–904.
| Polymer physics of intracellular phase transitions.Crossref | GoogleScholarGoogle Scholar |
[18] Hyman, AA et al. (2014) Liquid–liquid phase separation in biology. Annu Rev Cell Dev Biol 30, 39–58.
| Liquid–liquid phase separation in biology.Crossref | GoogleScholarGoogle Scholar |
[19] Patel, A et al. (2015) A liquid-to-solid phase transition of the ALS protein FUS accelerated by disease mutation. Cell 162, 1066–1077.
| A liquid-to-solid phase transition of the ALS protein FUS accelerated by disease mutation.Crossref | GoogleScholarGoogle Scholar |
[20] Dyson, HJ and Wright, PE (2005) Intrinsically unstructured proteins and their functions. Nat Rev Mol Cell Biol 6, 197–208.
| Intrinsically unstructured proteins and their functions.Crossref | GoogleScholarGoogle Scholar |
[21] Taglialegna, A et al. (2016) Staphylococcal Bap proteins build amyloid scaffold biofilm matrices in response to environmental signals. PLoS Pathog 12, e1005711.
| Staphylococcal Bap proteins build amyloid scaffold biofilm matrices in response to environmental signals.Crossref | GoogleScholarGoogle Scholar |
[22] Van Gerven, N et al. (2015) Bacterial amyloid formation: structural insights into curli biogensis. Trends Microbiol 23, 693–706.
| Bacterial amyloid formation: structural insights into curli biogensis.Crossref | GoogleScholarGoogle Scholar |
[23] Wong, LL et al. (2020) Extracellular protein isolation from the matrix of anammox biofilm using ionic liquid extraction. Appl Microbiol Biotechnol 104, 3643–3654.
| Extracellular protein isolation from the matrix of anammox biofilm using ionic liquid extraction.Crossref | GoogleScholarGoogle Scholar |
[24] Kim, YS et al. (2013) Gelation of the genome by topoisomerase II targeting anticancer agents. Soft Matter 9, 1656–1663.
| Gelation of the genome by topoisomerase II targeting anticancer agents.Crossref | GoogleScholarGoogle Scholar |
[25] Dumitriu S (ed.) (2004) Polysaccharides: Structural Diversity and Functional Versatility. 2nd edn. CRC Press.
| Crossref |
[26] Kundukad, B et al. (2016) Mechanical properties of the superficial biofilm layer determine the architecture of biofilms. Soft Matter 12, 5718–5726.
| Mechanical properties of the superficial biofilm layer determine the architecture of biofilms.Crossref | GoogleScholarGoogle Scholar |
[27] Wang, S et al. (2015) The exopolysaccharide Psl-eDNA interaction enables the formation of a biofilm skeleton in Pseudomonas aeruginosa. Environ Microbiol Rep 7, 330–340.
| The exopolysaccharide Psl-eDNA interaction enables the formation of a biofilm skeleton in Pseudomonas aeruginosa.Crossref | GoogleScholarGoogle Scholar |
[28] Jennings, LK et al. (2015) Pel is a cationic exopolysaccharide that cross-links extracellular DNA in the Pseudomonas aeruginosa biofilm matrix. Proc Natl Acad Sci USA 112, 11353–11358.
| Pel is a cationic exopolysaccharide that cross-links extracellular DNA in the Pseudomonas aeruginosa biofilm matrix.Crossref | GoogleScholarGoogle Scholar |
[29] Chew, SC et al. (2014) Dynamic remodeling of microbial biofilms by functionally distinct exopolysaccharides. mBio 5, e01536-14.
| Dynamic remodeling of microbial biofilms by functionally distinct exopolysaccharides.Crossref | GoogleScholarGoogle Scholar |
[30] Khan, F et al. (2022) Filamentous morphology of bacterial pathogens: regulatory factors and control strategies. Appl Microbiol Biotechnol 106, 5835–5862.
| Filamentous morphology of bacterial pathogens: regulatory factors and control strategies.Crossref | GoogleScholarGoogle Scholar |
[31] Beckwith, JK et al. (2022) Rheology of Candida albicans fungal biofilms. J Rheol 66, 683–697.
| Rheology of Candida albicans fungal biofilms.Crossref | GoogleScholarGoogle Scholar |
[32] Hemdan, BA et al. (2023) Bacterial community structure of electrogenic biofilm developed on modified graphite anode in microbial fuel cell. Sci Rep 13, 1255.
| Bacterial community structure of electrogenic biofilm developed on modified graphite anode in microbial fuel cell.Crossref | GoogleScholarGoogle Scholar |