Cerium oxide nanoparticles promoted lateral root formation in Arabidopsis by modulating reactive oxygen species and Ca2+ level
Guangjing Li A B , Quanlong Gao A B , Ashadu Nyande A B , Zihao Dong A B , Ehtisham Hassan Khan A B , Yuqian Han A B and Honghong Wu
A
B
C
D
Abstract
Roots play an important role in plant growth, including providing essential mechanical support, water uptake, and nutrient absorption. Nanomaterials play a positive role in improving plant root development, but there is limited knowledge of how nanomaterials affect lateral root (LR) formation. Poly (acrylic) acid coated nanoceria (cerium oxide nanoparticles, PNC) are commonly used to improve plant stress tolerance due to their ability to scavenge reactive oxygen species (ROS). However, its impact on LR formation remains unclear. In this study, we investigated the effects of PNC on LR formation in Arabidopsis thaliana by monitoring ROS levels and Ca2+ distribution in roots. Our results demonstrate that PNC significantly promote LR formation, increasing LR numbers by 26.2%. Compared to controls, PNC-treated Arabidopsis seedlings exhibited reduced H2O2 levels by 18.9% in primary roots (PRs) and 40.6% in LRs, as well as decreased levels by 47.7% in PRs and 88.5% in LRs. When compared with control plants, Ca2+ levels were reduced by 35.7% in PRs and 22.7% in LRs of PNC-treated plants. Overall, these results indicate that PNC could enhance LR development by modulating ROS and Ca2+ levels in roots.
Keywords: Ca2+, histochemical staining, laser confocal microscopy, lateral root formation, nanoceria, qPCR, ROS, transmission electron microscopy.
Introduction
The plant root system is essential for healthy growth, development, and high crop yields. It plays a crucial role in adapting to unfavourable environments by efficiently absorbing water, nutrients, and organic salts from the soil. Lateral roots (LRs), which originated from the primary root (PR), significantly increase the root surface area, aiding in anchorage, nutrient uptake, and water absorption (Ji et al. 2022; Singh et al. 2022; Zhang et al. 2023). Continuous production of LRs enhances plant fitness by expanding soil contact, thereby improving nutrient absorption and survival in nutrient-deficient or water-limited soils (Ji et al. 2022; Chieb and Gachomo 2023). Previous studies indicate that altering LR development patterns can enhance plant plasticity and survival under abiotic stress conditions, such as excessive nitrate levels, by optimising nutrient uptake (Ji et al. 2022).
Developmental biology investigates how plants utilise Ca2+ signals to regulate diverse cellular and developmental processes, particularly in LR formation. Studies have shown that the calcium-binding protein calmodulin IQ-motif containing protein (CaM-IQM) plays a crucial role by suppressing indole-3-acetic acid (IAA) inducible responses, thereby modulating auxin effects and promoting LR initiation (Zhang et al. 2022). Furthermore, gradients of calcium within the cytosol influence the activity of the PIN2 protein in Arabidopsis thaliana, impacting root architecture and LR development (Nisar et al. 2020). Additionally, calcium signals originating from mitochondria through nuclear pores affect primary root establishment, meristem development, and auxin distribution in Arabidopsis, underscoring the pivotal role of Ca2+ signalling in root system modulation (Leitão et al. 2019).
Reactive oxygen species (ROS) are also critical signalling molecules in the regulation of root architecture, influencing the development of root tissues, cells, and LRs (Pasternak et al. 2023). They maintain a delicate balance between cell division and differentiation within the meristem, with a particular emphasis on genes associated with root growth (Mase and Tsukagoshi 2021). ROS also interact with various signalling pathways, serving as a central hub for pathway integration (Prakash et al. 2020; Pasternak et al. 2023). Nanomaterials have emerged as promising candidates not only for reducing ROS accumulation but also for regulating plant root systems (Yan et al. 2013; Wang et al. 2021). This dual capability positions them as potential alternatives to traditional plant antioxidants for managing ROS levels in plant cells.
Cerium oxide nanoparticles (nanoceria) are potent ROS scavengers (Karakoti et al. 2008) and are widely used across different fields such as medical research, industrial application as catalysers, and plant science (Wu et al. 2017; Fu et al. 2022). It should be noted that soil enrichment of cerium is similar to copper. Even a low concentration of cerium could stimulate plant growth. Thus, owing to its ROS scavenging ability, nanoceria are widely used in improving plant tolerance to stresses, such as salinity (An et al. 2020), drought (Djanaguiraman et al. 2018), cold (Wu et al. 2017), and heat (Wu et al. 2017). Also, nanoceria improved plant root systems are commonly observed. For example, under salt stress, cerium oxide nanoparticles can improve the salt tolerance of cotton (Gossypium hirsutum) and cucumber (Cucumis sativus) and significantly enhance root development under salt stress (Liu et al. 2021; Peng et al. 2022). However, whether nanoceria can improve LR formation is relatively unknown, and there is limited understanding of how nanoceria improve LR formation. Previous studies showed that ammonia borane-loaded hollow mesoporous silica nanoparticles can improve LR formation in tomato plants by modulating nitric oxide levels (Wang et al. 2021). To investigate whether nanoeria could promote LR formation and the behind mechanisms is the main goal of this study.
In this study, poly (acrylic) acid coated nanoceria (PNC) were used. The synthesised PNC were characterised by zeta (ζ)-sizer and transmission electron microscopy (TEM). Its biological role in promoting LR formation in Arabidopsis was then investigated. The distribution and levels of ROS in both PR and LR through tissue determination and histochemical staining were studied. Furthermore, the distribution and levels of Ca2+ in PR and LR were monitored with laser confocal microscopy.
Materials and methods
Plant growth
Arabidopsis thaliana L. (Col-0) seeds were sterilised with 70% ethanol for 10 s, followed by two rinses in sterile water, treated with 5% NaClO for 10 min, and washed three times with sterile water. The seeds were then plated on Murashige and Skoog (MS) medium, vernalised at 4°C in darkness for 3 days, and subsequently grown under light conditions (23°C, 14/10 h light/dark cycle, 200 μmol m−2 s−1) for 5 days. Seedlings with uniform growth and root lengths were selected and transferred to either poly (acrylic) acid coated nanoceria (PNC)-treated or control MS medium (six seedlings per plate, four plates per treatment). After 7 days of treatment, seedlings were photographed, and root parameters were quantified using Image J software (National Institutes of Health, Bethesda, MD, USA).
Synthesis and characterisation of PNC
PNC were synthesised following a methodology adapted from previous publications (Wu et al. 2017). Cerium nitrate (1.08 g, 99%, Sigma-Aldrich) was dissolved in 2.5 mL of deionised water (solution A), while poly acrylic acid (4.5 g, 1800 MW, Sigma-Aldrich) was dissolved in 5.0 mL of deionised water (solution B). Solutions A and B were thoroughly mixed at 224g for 15 min using a vortexer and then added dropwise into a 50 mL beaker containing 15 mL of a 30% ammonium hydroxide solution. The mixture was stirred at room temperature (500 rpm) for 24 h. Subsequently, it was aliquoted into 1.5 mL centrifuge tubes and spun at 1920g for 1 h, with approximately 100 μL of liquid discarded from the bottom of each tube. The supernatant was transferred to an ultrafiltration tube (MWCO 30K, Millipore Inc.) and centrifuged at 1920g for six cycles (one cycle every 45 min). The resulting supernatant constituted the PNC solution, which was stored at 4°C.
The absorbance of the PNC solution was measured using an ultraviolet-visible spectrophotometer (UV-1800, AOE). PNC concentration was determined using the Beer-Lambert law (A = εCL), where A is the absorbance at 271 nm, ε is the molar absorption coefficient of PNC (3 cm−1 mM−1), L is the optical path length (cuvette width, 1 cm), and C is the molar concentration of PNC. The zeta (ζ) potential was evaluated using a 90 Plus PALS instrument (Brookhaven Instruments Corporation, USA). For transmission electron microscopy (TEM) imaging, 20 μL of PNC solution was deposited onto a holey carbon-coated copper grid and imaged using a FEI Talos microscope operating at 300 kV. The size of PNC in TEM images was measured using Image J software.
H2O2 and content
Quantification of H2O2 and levels was performed using commercial kits. H2O2 levels were measured with a kit from Nanjing Jiancheng Bioengineering Institute (Product No. A064-1-1). levels were determined using a kit from Solarbio Life Sciences (Product No. BC1295).
Diaminobenzidine (DAB) and nitro blue tetrazolium (NBT) staining
DAB and NBT staining followed the method described in a previous study (Kumar et al. 2013). Arabidopsis roots were stained with 1 mg mL−1 DAB (pH 5.5, 50 mM Tris-HCl) at 37°C for 6 h and 0.5 mg mL−1 NBT (pH 7.8, 50 mM phosphoric acid buffer) at 37°C for 1 h. Seedlings were subsequently washed rapidly with 70% ethanol and observed under an Olympus IX71 microscope. Quantitative analysis of staining intensity was conducted using Image J software.
Antioxidant enzyme activity assay
The activity levels of superoxide dismutase (SOD), peroxidase (POD), and catalase (CAT) were quantified using assay kits from Solarbio Life Sciences. Specifically, SOD activity was measured with Product No. BC5165, POD activity with Product No. BC0090, and CAT activity with Product No. BC0205. Each experiment included six replicates.
Imaging of Ca2+ distribution in roots
Fluo-3 AM (Thermo Fisher Scientific) and FM 4–64 (Thermo Fisher Scientific) were used as fluorescent dyes for Ca2+ and plasma membrane staining, respectively. Fluo-3 AM and FM 4–64 dyes were dissolved in pure dimethyl sulfoxide (DMSO) and diluted to 20 μM with TES buffer (10 mmol L−1, pH 6.1). For root staining, 5-day-old Arabidopsis plants were further root treated with PNC for 7 days. The roots were then stained with a mixture of 20 μM Fluo-3 AM and 20 μM FM 4–64, incubated in the dark for 2 h, and subsequently washed three to five times with TES buffer. Fluorescent signals from the roots were captured using a confocal laser scanning microscope (SP8, Leica, Germany) with the following settings: 40× objective; 488 nm excitation light; PMT1, 510−580 nm (Ca2+ fluorescence); and PMT2, 610−630 nm (plasma membrane fluorescence). Each experiment included 8–10 replicates, and Ca2+ fluorescence intensities were analysed using LAS AF Lite software as described in our previous studies (Wu et al. 2017; Liu et al. 2021).
Real time quantitative PCR
Total RNA was extracted using the EASYspin Plus Complex Plant RNA kit (RN53, Aidlab, Beijing, China), and 1 μg of total RNA was reverse transcribed into complementary DNA (cDNA) using the TRUEscript RT MasterMix kit (PC7002, Aidlab). Real time quantatitive PCR (qPCR) was performed using a 25-μL reaction mixture containing SYBR Green qPCR Mix (PC3302, Aidlab) following the manufacturer’s protocol. The qPCR analysis was conducted on a Bio-Rad CFX Connect Real-Time PCR System (Bio-Rad, CA, USA). Each gene was analysed with four biological replicates and three technical replicates. Relative gene expression was calculated using the 2-ΔΔCt method as mentioned elsewhere. For a list of primers used for qPCR, see Supplementary Table S1.
Statistical analysis
The statistical analysis was conducted using Excel 2019 and SPSS 23.0. Data are presented as mean ± s.e. (n = biological replicates). Comparisons were performed using an independent sample t-test (two-tailed) or one-way ANOVA followed by Duncan’s multiple range test (two-tailed). Statistical significance was denoted as: *P < 0.05; **P < 0.01; and ***P < 0.001. Different lowercase letters indicate significant differences.
Results and discussion
PNC characterisation
TEM analysis revealed that PNC exhibited good dispersion with an average particle size of 10.88 nm (Fig. 1a), consistent with previous studies (Wu et al. 2017). UV-Vis spectra showed absorption peaks of PNC at 271 nm (Fig. 1b). Zeta (ζ)-potential measurements showed that PNC have a negative charge of −41.7 ± 0.2 mV (Fig. 1c). These results are similar to previous studies (Wu et al. 2017; Liu et al. 2021; Li et al. 2022).
Screening of application concentration of PNC for LR formation
Previous studies showed that engineered nanomaterials promoted early root growth (Andersen et al. 2016). Here, we conducted a screening experiment to determine the optimal concentration of PNC applied to Arabidopsis roots. Arabidopsis seedlings (5 days old) were exposed to varying concentrations of PNC (0.05, 0.07, 0.1, 0.15, 0.2 mM), and the subsequent effects on LR development were investigated after 7 days (Fig. 2a). Increasing concentrations of PNC resulted in a gradual increase in LR density (LRD) in Arabidopsis, with enhancements of 3.5%, 22.9%, 17.9%, 27.5%, and 61.1% observed from the lowest to highest concentrations of PNC, respectively (Fig. 2b). However, at 0.15 mM, PNC significantly reduced the length of the primary root from 7.1 ± 0.05 cm to 6.68 ± 0.06 cm (Fig. 2c). Similarly, previous studies demonstrated that elevated concentrations of nanoceria decreased pea (Pisum sativum) root elongation while increasing LRD (Skiba et al. 2020). This suggests a general trend where higher concentrations of nanomaterials may inhibit PR growth while stimulating LR formation and altering root morphology. Determining the optimal concentration of nanomaterials that balances main root growth with LR development is crucial for their practical application in agriculture. In this study, to minimise potential interference from high nanoparticle concentrations in investigating LR development mechanisms, we identified 0.1 mM PNC as the optimal concentration for promoting LR formation in Arabidopsis without reducing the length of the primary root.
PNC concentration screening on Arabidopsis roots. (a) Phenotype, (b) average emerged lateral root density, and (c) root length of Arabidopsis seedlings where LR length is the total length of lateral roots for each Arabidopsis plant. Scale bar, 1 cm. Mean ± s.e. (n = 24). Different lowercase letters indicate the significance difference at P < 0.05.
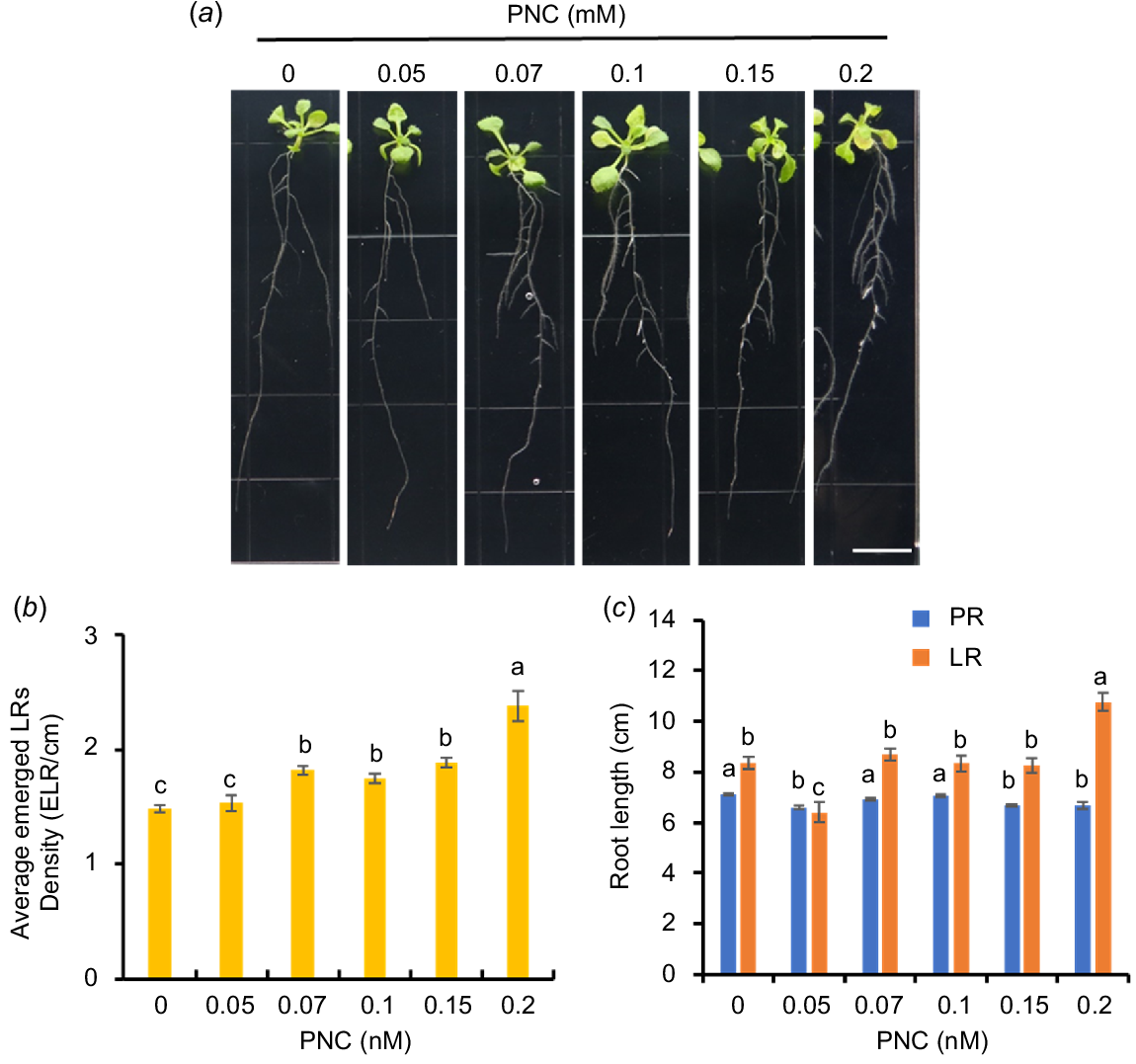
PNC promotes LR formation in Arabidopsis
Previous studies have extensively demonstrated the beneficial effects of nanoparticles on plant growth and development (Li et al. 2023; Huang et al. 2024). However, the less known is whether nanoparticles can promote LR formation, and the underlying mechanisms. In this study, we found a significant increase in the number of LRs in PNC-treated (0.1 mM) plants compared to the control (15.04 ± 0.46 vs 11.92 ± 0.37). Additionally, the LRD in PNC-treated plants also showed a significant increase (2.17 ± 0.05 vs 1.72 ± 0.05) than control plants, indicating enhanced LR formation. The length of both primary (6.91 ± 0.08 vs 6.93 ± 0.08) and lateral roots (8.05 ± 0.43 vs 8.41 ± 0.43) did not exhibit significant changes (Fig. 3a, b). Previous studies showed that PNC can enhance growth and salt tolerance in cucumbers by stimulating early antioxidant responses (Chen et al. 2022). Additionally, PNC has been shown to elevate salicylic acid levels and enhance ROS scavenging during seed germination, thereby improving salt tolerance in rapeseed (Brassica napus) (Khan et al. 2022). PNC also mitigates membrane oxidation damage and enhances salt tolerance in rapeseed through modulation of Cu-Zn SOD and lipid oxidase-IV isoenzyme activities (Li et al. 2022), and maintains cytoplasmic K+/Na+ ratios, thereby improving salt tolerance in cotton (Liu et al. 2021). In this work, we showed that PNC can improve LR formation in Arabidopsis, underscoring its potential in agricultural applications.
PNC reduce ROS levels in primary and lateral roots
ROS play crucial roles in root growth and the initiation of LRs (Orman-Ligeza et al. 2016; Pasternak et al. 2023). During LR emergence, both H2O2 and accumulate in the vascular zone and epidermis/cortex of the mature root zone (Pasternak et al. 2023). H2O2 regulates auxin distribution by modulating polar auxin transport, thereby promoting LR formation and influencing root system architecture (Su et al. 2016). In this study, we assessed the impact of PNC application on Arabidopsis root ROS content (Fig. 4a, b). Our results show that roots treated with PNC exhibited reduced levels of H2O2 and , compared to control plants. Specifically, H2O2 levels decreased in both PRs (0.43 ± 0.04 vs 0.53 ± 0.02) and LRs (0.41 ± 0.05 vs 0.64 ± 0.10) after PNC treatment (Fig. 4a). Furthermore, content decreased by 47.7% in PRs (1.74 ± 0.21 vs 3.65 ± 0.15) and by 88.5% in LRs (0.12 ± 0.01 vs 1.04 ± 0.20) after PNC treatment (Fig. 4b).
Contents of (a) H2O2 and (b) in Arabidopsis primary root (PR) and lateral root (LR) after 7 days of treatment with PNC. Mean ± s.e. (n = 8–10). *P < 0.05; **P < 0.01; ***P < 0.001.
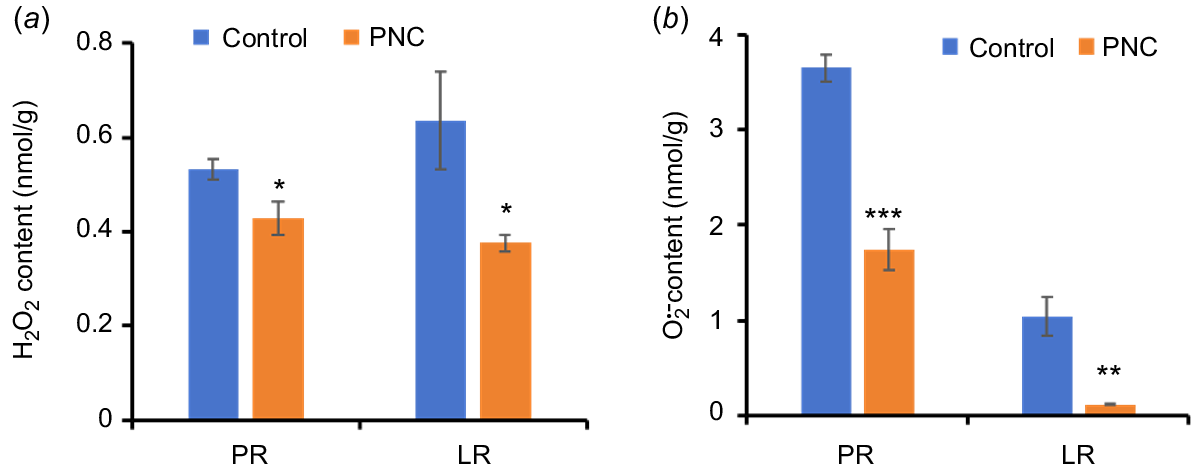
To confirm the ROS findings, in situ histochemical staining for H2O2 (Fig. 5a) and (Fig. 5b) was conducted within the root system. Roots of control plants exhibited strong intensity with 3,3′-DAB (dark brown) and NBT (purple-blue). In contrast, Arabidopsis roots treated with PNC showed reduced staining intensity for both H2O2 and compared to control plants. Specifically, DAB staining intensity decreased by 3.3% in the PRs (201.14 ± 1.15 vs 208.73 ± 1.28) and by 7.2% in LRs (165.59 ± 6.36 vs 178.43 ± 1.92) (Fig. 5c). NBT staining intensity decreased by 5.8% in the PRs (218.07 ± 3.09 vs 231.61 ± 3.30) and by 10.1% in LRs (150.95 ± 3.47 vs 167.91 ± 2.67) (Fig. 5d). These results demonstrate that PNC reduces ROS levels in both primary and lateral roots during the promotion of LR formation in Arabidopsis. Interestingly, a previous study on cucumber plants indicated that PNC application increased NBT intensity in the root apex (Chen et al. 2022), suggesting species-specific variations in ROS responses to PNC. Factors such as root exudates, root anatomy, and uptake efficiency may contribute to these observed differences. These findings underscore the intricate relationship between ROS regulation and PNC effects on root physiology, providing insights into potential mechanisms underlying root development modulation. Mechanistic insights gained from this study highlight how PNC affects root development through ROS modulation. Specifically, the PNC-induced reduction in ROS levels likely influences auxin distribution and transport, which are critical for LR initiation and elongation (Su et al. 2016). Further investigation into the specific pathways that PNC interacts with ROS signalling cascades could provide deeper insights into its mode of action in root development. These findings underscore potential applications of PNC and similar compounds in agriculture. By manipulating ROS levels through chemical treatments, researchers and farmers could potentially enhance root growth, nutrient uptake efficiency, and stress tolerance in crops.
Staining of Arabidopsis roots with (a, c) DAB and (b, d) NBT. Scale bar, 100 μm. Mean ± s.e. (n = 8–10). *P < 0.05; **P < 0.01; ***P < 0.001.
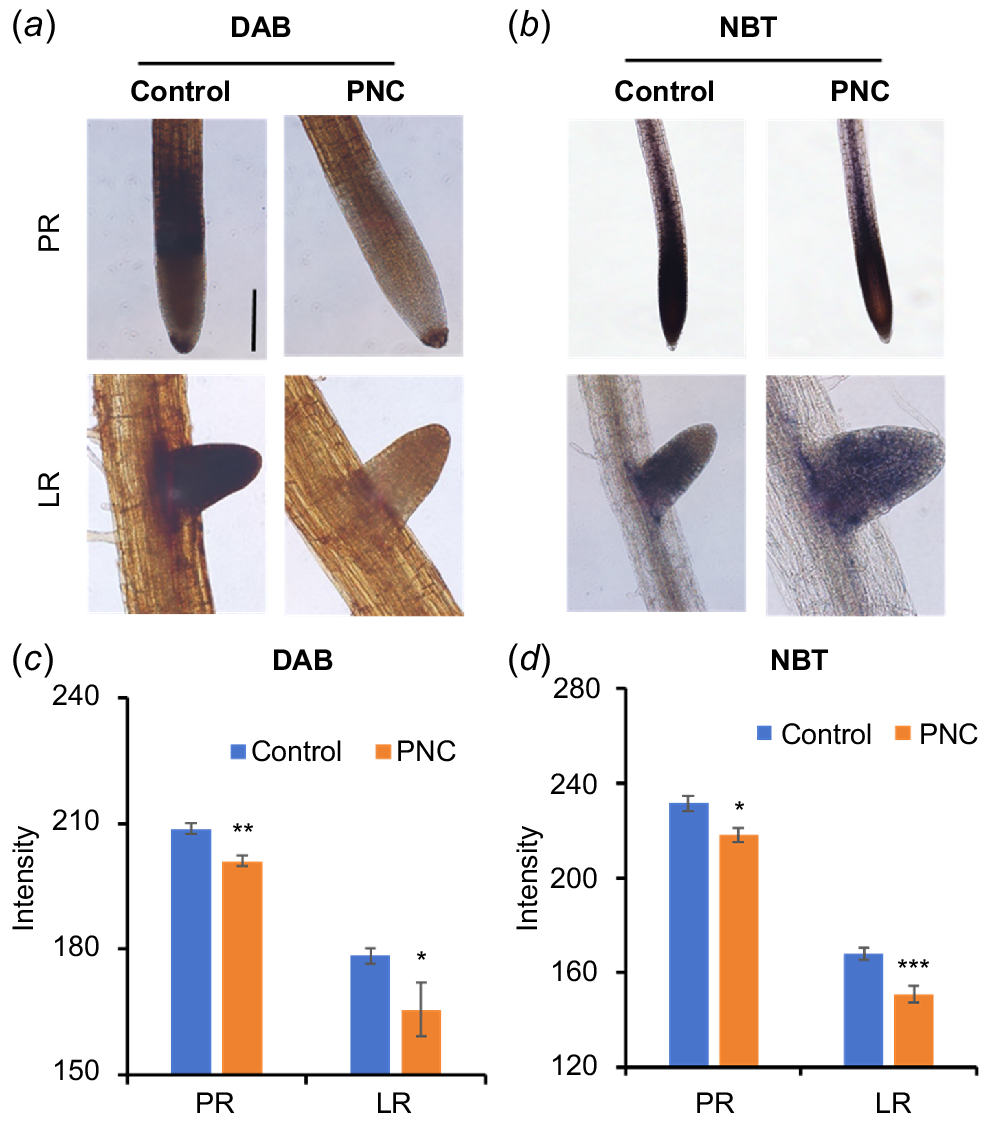
Antioxidant enzymes such as POD, SOD, and CAT, play a crucial role in degrading ROS, thereby reducing their accumulation in cells (Dumanović et al. 2021). The observed decrease in H2O2 and in the PR and LR of Arabidopsis may be associated with increased antioxidant enzyme activity. When compared to the control group after 7 days of PNC treatment, POD and SOD activities in the roots were significantly enhanced by 29.4% (100,124.29 ± 6681.17 vs 77,374.16 ± 3023.43) and 25.5% (289.91 ± 3.52 vs 230.36 ± 23.67), respectively (Fig. 6a, b). No significant difference in CAT activity was observed between the control and PNC treatment groups (Fig. 6c). The increased POD and SOD activities effectively neutralised H2O2 and , which contributed to their reduced levels in the root system.
PNC reduces Ca2+ levels in Arabidopsis roots
Previous studies showed that Ca2+ is a crucial signalling molecule in the process of LR formation in plants (Zhang et al. 2022). To investigate the impact of PNC on Ca2+ signalling during LR development, we utilised the Ca2+-specific fluorescent dye Fluo-3AM to monitor Ca2+ levels in Arabidopsis roots. Ca2+ fluorescence signals were observed in both the PR tip and LRs of Arabidopsis plants (Fig. 7a). Our results reveal that treatment with PNC resulted in reduced Ca2+ fluorescence levels in both the primary and lateral roots. Specifically, compared to the control group, Ca2+ fluorescence intensity decreased by 35.7% in the primary root (13.75 vs 21.40) and by 22.7% in the lateral roots (10.40 vs 13.46) (Fig. 7b). Changes in Ca2+ dynamics can significantly affect various cellular processes, including root growth and development. The reduced Ca2+ fluorescence intensity observed in both PR and LR suggests that PNC may disrupt processes such as Ca2+ influx, efflux, or intracellular compartmentalisation. Such disruptions could alter the activation of Ca2+-dependent proteins and downstream signalling cascades critical for LR initiation and elongation. These findings are consistent with previous research highlighting the regulatory role of Ca2+ in root architecture. For example, fluctuations in Ca2+ levels have been associated with changes in root meristem activity, cell differentiation, and responses to environmental stimuli (Zhang et al. 2022). Our study suggested the important role of Ca2+ in mediating PNC effects on LR development in Arabidopsis.
Ca2+ distribution in Arabidopsis roots with (a) confocal images of Fluo-3/AM fluorescence showing Ca2+ accumulation, and (b) fluorescence intensity of Fluo-3/AM. Scale bar, 100 μm. Mean ± s.e. (n = 8–10). **P < 0.01; ***P < 0.001.
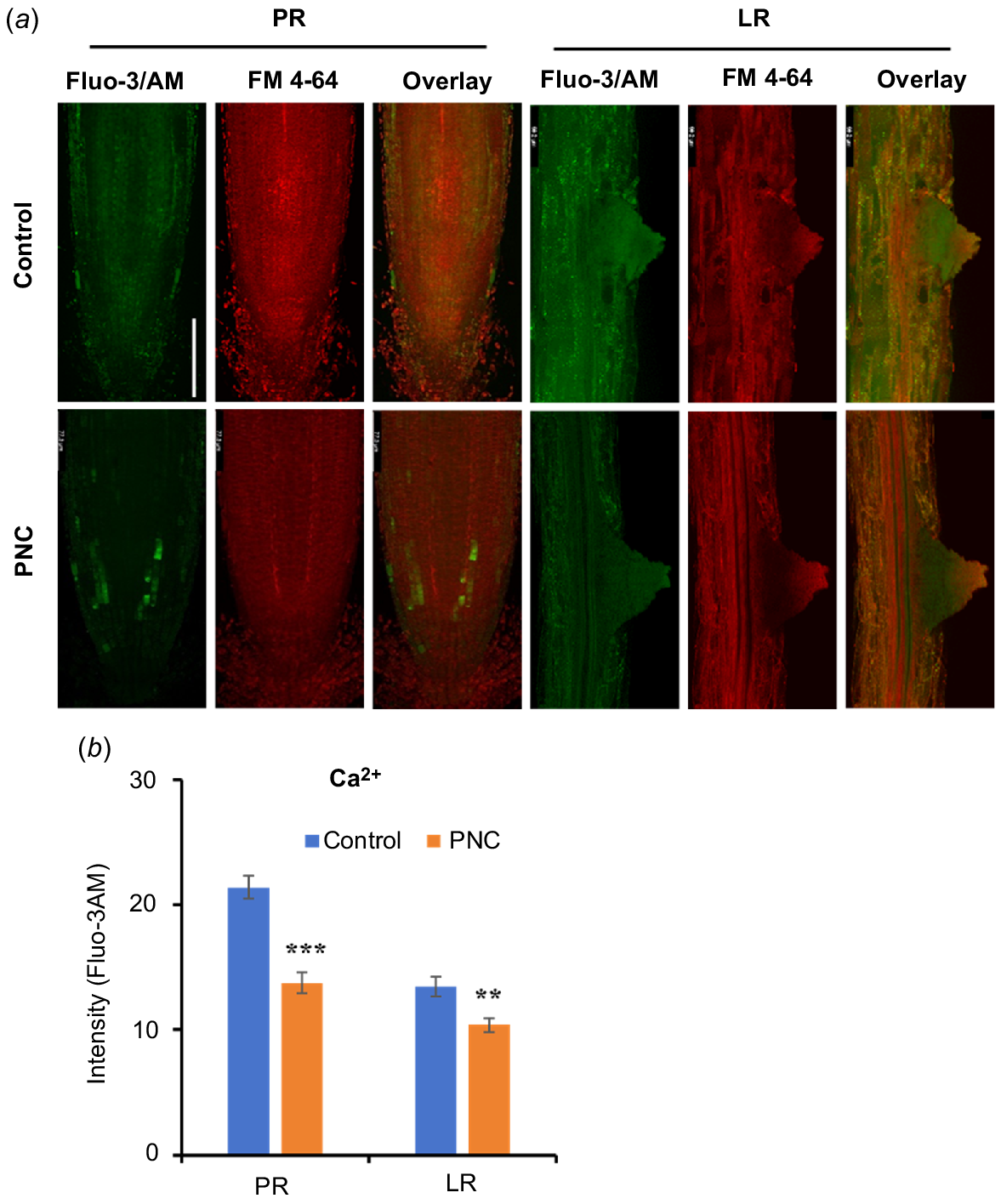
The ACA gene family (autoinhibited Ca2+-ATPase) is a critical component of calcium regulation in plants, and is responsible for maintaining intracellular calcium balance through the active transport of Ca2+. This family of calcium pumps plays essential roles in various physiological processes, including cell signalling, environmental stress responses, root development, and overall plant growth (Rahmati Ishka et al. 2021). In our study, Arabidopsis roots treated with PNC exhibited a decrease in intracellular calcium concentration. Concurrently, the expression levels of ACA1 and ACA2 genes were significantly upregulated, whereas ACA8 and ACA10 showed no substantial changes in expression (Fig. 8). These observations suggest that ACA1 and ACA2 are predominantly involved in regulating calcium distribution under PNC treatment. The observed upregulation of ACA1 and ACA2 likely enhances the activity of these calcium pumps, facilitating increased calcium ion transport or excretion, thereby contributing to the reduction of intracellular calcium levels. Conversely, the stable expression of ACA8 and ACA10 indicates that these genes have a minimal impact on calcium concentration regulation in this specific context. This differential expression pattern underscores the distinct roles and regulatory mechanisms of various ACA family members in response to PNC treatment, highlighting the specialised functions of ACA1 and ACA2 in managing calcium dynamics in plant roots.
Conclusions
In this study, PNC (10.88 nm, −41.7 ± 0.2 mV) was used on Arabidopsis roots to investigate its effects on ROS and Ca2+ distribution in both primary and lateral roots of seedlings, focusing on enhancing LR formation. Our results demonstrate that PNC treatment leads to a reduction in ROS levels in root by enhancing the activities of POD and SOD. Additionally, PNC treatment upregulated the expression of ACA1 and ACA2 genes in the roots, which contributed to a decrease in intracellular Ca2+ concentration. These results provide foundational support for the application of PNC in agricultural practices aimed at improving root architecture and overall crop productivity. Further exploration of PNC’s mechanisms and long-term effects on plant growth will be crucial for harnessing its full potential in sustainable agriculture.
Data availability
The data that support this study are available in the article and accompanying online supplementary material.
Conflicts of interest
Honghong Wu is an Associate Editor of Functional Plant Biology. To mitigate this potential conflict of interest, they had no editor-level access to this manuscript during peer review. The authors declare no other known competing financial interests or personal relationships that could have appeared to influence the work reported in this paper.
Declaration of funding
This work was supported by the National Key Research and Development Program of China (2023YFD19101700-3), the NSFC grant (No. 32071971), Fundamental Research Funds for the Central Universities (2662024JC011), the HZAU-AGIS Cooperation Fund (SZYJY2021008), the Key Research and Development Projects of Henan Province (231111113000), and the Hubei Agricultural Science and Technology Innovation Center Program (2021-620-000-001-032) to H.W.
Author contributions
Guangjing Li: Conceptualisation, Data curation, Formal analysis, Investigation, Methodology, Validation, Visualisation, Writing – original draft, Writing – review and editing. Quanlong Gao and Ashadu Nyande: Writing – original draft, Writing – review and editing. Zihao Dong: Writing – original draft, Writing – review and editing. Ehtisham Hassan Khan: Writing – review and editing. Yuqian Han: Writing – review and editing. Honghong Wu: Supervision, Conceptualisation, Funding acquisition, Writing – review and editing.
Acknowledgements
The authors thank A/Prof. Mr. Jianbo Cao and Ms. Limin He for their help in TEM imaging at the Public Laboratory of Electron Microscopy, Huazhong Agricultural University.
References
An J, Hu P, Li F, Wu H, Shen Y, White JC, Tian X, Li Z, Giraldo JP (2020) Emerging investigator series: molecular mechanisms of plant salinity stress tolerance improvement by seed priming with cerium oxide nanoparticles. Environmental Science: Nano 7, 2214-2228.
| Crossref | Google Scholar |
Andersen CP, King G, Plocher M, Storm M, Pokhrel LR, Johnson MG, Rygiewicz PT (2016) Germination and early plant development of ten plant species exposed to titanium dioxide and cerium oxide nanoparticles. Environmental Toxicology and Chemistry 35, 2223-2229.
| Crossref | Google Scholar | PubMed |
Chen L, Peng Y, Zhu L, Huang Y, Bie Z, Wu H (2022) CeO2 nanoparticles improved cucumber salt tolerance is associated with its induced early stimulation on antioxidant system. Chemosphere 299, 134474.
| Crossref | Google Scholar | PubMed |
Chieb M, Gachomo EW (2023) The role of plant growth promoting rhizobacteria in plant drought stress responses. BMC Plant Biology 23, 407.
| Crossref | Google Scholar | PubMed |
Djanaguiraman M, Nair R, Giraldo JP, Prasad PVV (2018) Cerium oxide nanoparticles decrease drought-induced oxidative damage in sorghum leading to higher photosynthesis and grain yield. ACS Omega 3, 14406-14416.
| Crossref | Google Scholar | PubMed |
Dumanović J, Nepovimova E, Natić M, Kuča K, Jaćević V (2021) The significance of reactive oxygen species and antioxidant defense system in plants: a concise overview. Frontiers in Plant Science 11, 552969.
| Crossref | Google Scholar | PubMed |
Fu C, Khan MN, Yan J, Hong X, Zhao F, Chen L, Ma H, Li Y, Li J, Wu H (2022) Mechanisms of nanomaterials for improving plant salt tolerance. Crop and Environment 2, 92-99.
| Crossref | Google Scholar |
Huang Q, Ayyaz A, Farooq MA, Zhang K, Chen W, Hannan F (2024) Silicon dioxide nanoparticles enhance plant growth, photosynthetic performance, and antioxidants defence machinery through suppressing chromium uptake in Brassica napus L. Environmental Pollution 342, 123013.
| Crossref | Google Scholar | PubMed |
Ji R, Min J, Wang Y, Kronzucker HJ, Shi W (2022) The role of plant growth regulators in modulating root architecture and tolerance to high-nitrate stress in tomato. Frontiers in Plant Science 13, 864285.
| Crossref | Google Scholar | PubMed |
Karakoti AS, Monteiro-Riviere NA, Aggarwal R, Davis JP, Narayan RJ, Self WT, McGinnis J, Seal S (2008) Nanoceria as antioxidant: synthesis and biomedical applications. JOM (1989) 60, 33-37.
| Crossref | Google Scholar | PubMed |
Khan MN, Li Y, Fu C, Hu J, Chen L, Yan J, Khan Z, Wu H, Li Z (2022) CeO2 nanoparticles seed priming increases salicylic acid level and ROS scavenging ability to improve rapeseed salt tolerance. Global Challenges 6, 2200025.
| Crossref | Google Scholar | PubMed |
Kumar D, Yusuf MA, Singh P, Sardar M, Sarin NB (2013) Modulation of antioxidant machinery in α-tocopherol-enriched transgenic Brassica juncea plants tolerant to abiotic stress conditions. Protoplasma 250, 1079-1089.
| Crossref | Google Scholar | PubMed |
Leitão N, Dangeville P, Carter R, Charpentier M (2019) Nuclear calcium signatures are associated with root development. Nature Communications 10, 4865.
| Crossref | Google Scholar | PubMed |
Li Y, Liu J, Fu C, Khan MN, Hu J, Zhao F, Wu H, Li Z (2022) CeO2 nanoparticles modulate Cu-Zn superoxide dismutase and lipoxygenase-IV isozyme activities to alleviate membrane oxidative damage to improve rapeseed salt tolerance. Environmental Science: Nano 9, 1116-1132.
| Crossref | Google Scholar |
Li Y, Xi K, Liu X, Han S, Han X, Li G, Yang L, Ma D, Fang Z, Gong S, Yin J, Zhu Y (2023) Silica nanoparticles promote wheat growth by mediating hormones and sugar metabolism. Journal of Nanobiotechnology 21, 2.
| Crossref | Google Scholar | PubMed |
Liu J, Li G, Chen L, Gu J, Wu H, Li Z (2021) Cerium oxide nanoparticles improve cotton salt tolerance by enabling better ability to maintain cytosolic K+/Na+ ratio. Journal of Nanobiotechnology 19, 153.
| Crossref | Google Scholar | PubMed |
Mase K, Tsukagoshi H (2021) Reactive oxygen species link gene regulatory networks during Arabidopsis root development. Frontiers in Plant Science 12, 660274.
| Crossref | Google Scholar | PubMed |
Nisar M, Ali Z, Ali A, Aman R, Park HJ, Ullah I, Ullah A, Yun DJ (2020) CaСl2 salt signaling in primary root architecture and lateral root emergence in Arabidopsis thaliana. Russian Journal of Plant Physiology 67, 515-520.
| Crossref | Google Scholar |
Orman-Ligeza B, Parizot B, de Rycke R, Fernandez A, Himschoot E, Van Breusegem F (2016) RBOH-mediated ROS production facilitates lateral root emergence in Arabidopsis. Development 143, 3328-3339.
| Google Scholar | PubMed |
Pasternak T, Palme K, Pérez-Pérez JM (2023) Role of reactive oxygen species in the modulation of auxin flux and root development in Arabidopsis thaliana. The Plant Journal 114, 83-95.
| Crossref | Google Scholar | PubMed |
Peng Y, Chen L, Zhu L, Cui L, Yang L, Wu H, Bie Z (2022) CsAKT1 is a key gene for the CeO2 nanoparticle’s improved cucumber salt tolerance: a validation from CRISPR-Cas9 lines. Environmental Science: Nano 9, 4367-4381.
| Crossref | Google Scholar |
Prakash V, Vishwakarma K, Singh VP, Rai P, Ramawat N, Tripathi DK, Sharma S (2020) NO and ROS implications in the organization of root system architecture. Physiologia Plantarum 168, 473-489.
| Crossref | Google Scholar | PubMed |
Rahmati Ishka M, Brown E, Rosenberg A, Romanowsky S, Davis JA, Choi W-G, Harper JF (2021) Arabidopsis Ca2+-ATPases 1, 2, and 7 in the endoplasmic reticulum contribute to growth and pollen fitness. Plant Physiology 185, 1966-1985.
| Crossref | Google Scholar |
Singh P, Singh RK, Zhou Y, Wang J, Jiang Y, Shen N, Wang Y, Yang L, Jiang M (2022) Unlocking the strength of plant growth promoting Pseudomonas in improving crop productivity in normal and challenging environments: a review. Journal of Plant Interactions 17, 220-238.
| Crossref | Google Scholar |
Skiba E, Pietrzak M, Gapinska M, Wolf WM (2020) Metal homeostasis and gas exchange dynamics in Pisum sativum L. exposed to cerium oxide nanoparticles. International Journal of Molecular Sciences 21, 8497.
| Crossref | Google Scholar | PubMed |
Su C, Liu L, Liu H, Ferguson BJ, Zou Y, Zhao Y, Wang T, Wang Y, Li X (2016) H2O2 regulates root system architecture by modulating the polar transport and redistribution of auxin. Journal of Plant Biology 59, 260-270.
| Crossref | Google Scholar |
Wang Y, Lv P, Kong L, Shen W, He Q (2021) Nanomaterial-mediated sustainable hydrogen supply induces lateral root formation via nitrate reductase-dependent nitric oxide. Chemical Engineering Journal 405, 126905.
| Crossref | Google Scholar |
Wu H, Tito N, Giraldo JP (2017) Anionic cerium oxide nanoparticles protect plant photosynthesis from abiotic stress by scavenging reactive oxygen species. ACS Nano 11, 11283-11297.
| Crossref | Google Scholar | PubMed |
Yan S, Zhao L, Li H, Zhang Q, Tan J, Huang M, He S, Li L (2013) Single-walled carbon nanotubes selectively influence maize root tissue development accompanied by the change in the related gene expression. Journal of Hazardous Materials 246–247, 110-118.
| Crossref | Google Scholar | PubMed |
Zhang S, Yu R, Yu D, Chang P, Guo S, Yang X, Liu X, Xu C, Hu Y (2022) The calcium signaling module CaM–IQM destabilizes IAA–ARF interaction to regulate callus and lateral root formation. Proceedings of the National Academy of Sciences 119, e2202669119.
| Crossref | Google Scholar |
Zhang W, Fang D, Dong K, Hu F, Ye Z, Cao J (2023) Insights into the environmental factors shaping lateral root development. Physiologia Plantarum 175, e13878.
| Crossref | Google Scholar | PubMed |