Glycoside hydrolases reveals their differential role in response to drought and salt stress in potato (Solanum tuberosum)
Aiana A , Hanny Chauhan A and Kashmir Singh
A
Abstract
Glycoside hydrolases (GHs) are important in metabolic processes involving diverse carbohydrate-based substances found inside plant tissues. Potatoes (Solanum tuberosum) are rich in starchy carbohydrates, suggesting the role of GHs in their metabolic pathways. In this study, we examine the GH superfamily in potato where 366 potential GHs were identified using a similarity search method. Genes were subjected to further characterisation to gain insights into their structural composition, functional properties and distribution patterns across tissue types. Several in silico methodologies were also employed to investigate the physicochemical features, conserved motifs, chromosomal mapping, duplication events, syntenic links with tomato (Solanum lycopersicum), subcellular localisations, secondary structures and phylogenetic relationships. Cis-elements in StGHs revealed that the promoters of StGHs contain cis-elements that are responsive to phytohormones that are involved in plant growth and development, and are associated with stress responses. RNA-seq data identified significant changes in expression levels of GH16, GH17, GH18, GH19 and GH28 members under stress conditions. Expression patterns of several GHs were confirmed using real time quantitative PCR in response to stress. StGH16.24 expression increased after 3 days of drought stress, whereas StGH16.30 continuously increased under salt stress. Potential interactions between potato miRNAs and StGH revealed 393 and 627 interactions under drought and salt stress, respectively. Our findings offer insights into specific functions of GHs in diverse developmental stages and stress-related challenges in potato and other plants.
Keywords: β-galactosidases, carbohydrate metabolism, drought stress, expression analysis, glycoside hydrolases, microRNAs, salt stress, Solanum tuberosum.
Introduction
Carbohydrates constitute the predominant proportion of organic materials found on Earth. A broad and heterogeneous array of enzymes participate in the facilitation of both the production and breakdown processes of these sugars. The process of carbohydrate hydrolysis is primarily catalysed by a specific class of enzymes known as glycoside hydrolases (GHs) (EC 3.2.1.x) (Henrissat, 1991; Coutinho and Henrissat 1999). The primary function of these enzymes is to catalyse the hydrolysis of glycosidic linkages, which occur between two carbohydrate molecules or between a carbohydrate and a protein or lipid molecule. Genes that encode GHs are present in a wide range of species, spanning from archaea and bacteria to mammals and plants. Plants possess a considerable abundance of these genes, primarily attributed to their photosynthetic capacity and the amount of carbohydrates within their cell walls. According to the Carbohydrate-Active enZymes (CAZy) database, GHs have been categorised into 180 families based on similarities in their amino acid sequences. Additionally, they have been grouped into 18 clans based on similarities in their structures and mechanisms. Nevertheless, discerning the precise physiological functions of different GHs solely based on their respective enzyme families poses challenges, as enzymes belonging to distinct families might exhibit identical substrate and product specificity.
GHs are recognised as being implicated in a range of plant processes, encompassing starch hydrolysis, cell signalling, plant defence mechanisms and cell wall metabolism (Henrissat et al. 2001). The processes of cell wall synthesis and polysaccharide metabolism in plants are known to require GH enzymes (Cosgrove 2005; Minic and Jouanin 2006). GHs can carry out cell wall polysaccharide modifications in an efficient manner, utilising a relatively small number of enzymes (Minic et al. 2004, 2006). In addition to their role in other biological processes, these enzymes possess the ability to regulate the relaxation of the plant cell wall, govern growth and development, facilitate abscission, promote seed germination, regulate cell adhesion and facilitate fruit ripening (Lashbrook et al. 1994; Atkinson et al. 2002; Cosgrove et al. 2002; Lashbrook 2005). Vacuolar invertases, classified as members of the GH32 family, play a crucial role in facilitating the mobilisation of sucrose and regulating the sugar composition in storage organs and fruits. Additionally, these enzymes have a role in processes such as cell expansion, osmoregulation, cold sweetening, wound healing and interaction reactions (Roitsch et al. 2003; Sherson et al. 2003).
Solanum tuberosum (potato) belongs to the Solanaceae family, that includes many economically significant species and is part of the Petota section of the Solanum genus (Spooner et al. 2014). Recently various genome-wide analysis studies of gene families such as GPXL (glutathione peroxidase-like enzyme) gene family (Wang et al. 2023), C3H Zinc Finger family (Deng et al. 2023), 2ODD (2-Oxoglutatrate and Fe(II)-dependent dioxygenases) gene family (Chauhan et al. 2023) among many others have been carried out in potato. Downregulation of the potato gene MAL1, encoding α-glucosidase II-like activity, led to severe growth inhibition and reduced tuber yield in transgenic plants (Taylor et al. 2000). In S. tuberosum, specific GH3 genes, such as StGH3.12, showed significant responses to methyl jasmonate treatment, suggesting their involvement in jasmonic acid (JA) adenylation processes (Zhang et al. 2018).
Expression pattern analyses in Beta vulgaris showed differential regulation of XTHs (xyloglucan endotransglucosylase/hydrolases) in response to multiple phytohormones such as abscisic acid (ABA), JA, gibberellic acid (GA) and brassinosteroids (Gao et al. 2024). Multiple GH families, including GH2, GH3, GH18, GH35, GH38 and GH79 have been implicated in the probable involvement of N- and O-glycan processing in plant secretory proteins (Minic et al. 2007). It was shown that GH13 members play significant roles in stress responses and seed germination in Triticum aestivum (Yin et al. 2024). Multiple GHs, including β-D-galactosidase, α-L-arabinofuranosidase and β-D-glucuronidase collaborate in the process of breaking down the carbohydrate components of arabinogalactan proteins (Kotake et al. 2006). Kong et al. (2024) revealed that GH1 β-glucosidases in Medicago sativa were involved in abiotic and biotic stress tolerance. ZmXTH30, a Zea mays xyloglucan endotransglucosylase/hydrolase (GH16) protein was found to confer drought tolerance in Arabidopsis thaliana (Fu et al. 2024). TaXTH17, a wheat xyloglucan endotransglucosylase/hydrolase gene, localised in the secretory pathway and cell wall, was found to play a potential role in stress responses as it was induced by abiotic stresses and phytohormone treatments. It was observed that TaXTH17 negatively impacted plant tolerance to salt and drought stresses (Bi et al. 2024). Chandrasekar et al. (2022) revealed that the barley (Hordeum vulgare) β-1,3-endoglucanase HvBGLUII (GH17) releases a β-1,3;1,6-glucan decasaccharide (β-GD) from the extracellular polysaccharide (EPS) matrices of various fungi, which helps fungi evade plant immunity. Certain pathogenesis-related proteins have been found to be associated withGHs that have a role in plant pathogen responses. Chitinases found in many plant tissues and organs are classified within the GHs families 18 and 19. The overexpression of chitinase and β-1,3-glucanase in transgenic plants has been found to result in heightened resistance to oomycete or fungal infections (Brogue et al. 1991; Lin et al. 1995; Grison et al. 1996). This resistance is attributed to the production of oligosaccharides by the hydrolysis of fungal cell walls, which subsequently triggers the activation of defence mechanisms in plants. Studies using activity-based protein profiling (ABPP) have demonstrated that Pseudomas syringae infections cause a suppression of plant chitinase activity (Sueldo et al. 2024). VdEPG1, a GH28 family endopolygalacturonase, was observed to act as a virulence factor in Verticillium dahliae infection. Its expression significantly increased in V. dahliae-infected root of cotton (Gossypium hirsutum), and its knockout markedly reduced the virulence of the pathogen (Liu et al. 2023). Sueldo et al. (2024) found that a neo-functionalised pathogenesis-related NbPR3 (GH19) exhibited antibacterial activity when overexpressed, suggesting a role in plant immunity against bacteria. GH35 family proteins AtBGAL8 and NbBGAL1 increased resistance to P. syringae. pv. tabaci 6605 (Pta6605) when heterologously expressed in Nicotiana benthamiana (Buscaill et al. 2021). GHs have the potential to influence the glycosylation state and hormonal activity of phytohormones.
There is a lack of information regarding GHs in potato and other members of the Solanaceae family, their potential involvement in conferring resistance to abiotic and biotic stresses, and their participation in phytohormone signalling. In this study, we performed genome-wide identification of the GH gene superfamily in S. tuberosum and classified the identified genes into different families on the basis of domains present in all GHs. The physicochemical properties, chromosomal distribution, gene structure, conserved motifs, gene duplication, synteny and phylogenetic analysis of the putative GHs were performed. Protein–protein interaction, cis-regulatory elements and interaction of stu-miRNAs with StGHs was also analysed. We also studied the expression patterns of GHs in different tissues in potato and the differential expression of these genes under various abiotic and biotic stresses. The expression of some of the differentially expressed genes under drought and salt stress was analysed using real time quantitative PCR (qPCR). The results obtained could be valuable in substantiating the involvement of some of the GHs in stress tolerance, hormonal responses and cell metabolism.
Materials and methods
Identification and analysis of glycoside hydrolases (GH) genes in potato
For the identification of Solanum tuberosum L. GH proteins, a standalone BLASTp search was conducted with an e-value cut-off (≤e − 5) using previously identified GH protein sequences of Arabidopsis thaliana L., Nicotiana tabacum L., Solanum lycopersicum L., Capsicum annus L., Zea mays L. and Triticum aestivum L. downloaded from Uniprot (https://www.uniprot.org/) as query against the proteome of S. tuberosum. The resulting sequences were confirmed for the presence of GH domain using Pfam (http://pfam.xfam.org/) (Mistry et al. 2021), NCBI protein Batch CD-Search database (https://www.ncbi.nlm.nih.gov/Structure/bwrpsb/bwrpsb.cgi) (Lu et al. 2020), InterProScan (https://www.ebi.ac.uk/interpro/) (Paysan-Lafosse et al. 2023) and SMART (http://smart.embl-heidelberg.de/) (Letunic et al. 2021). All the resulting genes were then classified into separate families on the basis of domains present in the proteins. The physico-chemical properties of StGH proteins including the number of amino acid residues, molecular weight, isoelectric point, instability index, flex and GRAVY were analysed using the ProtParam tool (https://web.expasy.org/protparam/) (Gasteiger et al. 2005). Subcellular localisation of all proteins was predicted using the DeepLoc-2.0 online server (https://services.healthtech.dtu.dk/services/DeepLoc-2.0/) (Thumuluri et al. 2022). The secondary structure of StGH proteins was estimated using SOPMA (https://npsa-prabi.ibcp.fr/cgi-bin/npsa_automat.pl?page=/NPSA/npsa_sopma.html) (Geourjon and Deléage 1995).
Chromosomal mapping, nomenclature, gene duplication events and synteny analysis
The sequences and chromosomal locations of all StGHs were retrieved from Potato Genome Sequencing Consortium (PGSC; http://spuddb.uga.edu/). The positions of all genes on their respective chromosomes were mapped using TBTools software (Chen et al. 2020). All genes were renamed according to their respective families and location on the chromosomes with the prefix ‘StGH’. Gene duplication events in StGHs were predicted by performing bidirectional blast (e-value 10−10) and the genes showing >80% similarity were considered to be duplicated. The duplicated genes located within 5 Mb of the same chromosome were considered tandem duplications, while those located beyond 5 Mb were considered to be segmentally duplicated (Shumayla et al. 2016). To investigate the evolutionary significance of gene duplications, Ka/Ks ratio was calculated using TBTools software (Chen et al. 2020). Syntenic relation was explored in potato and tomato by retrieving syntenic genes from Ensembl Plants (https://plants.ensembl.org/index.html). Both gene duplication and syntenic analysis were visualised using TBTools (Chen et al. 2020).
Analysis of gene structure and conserved motifs
Exon-intron organisation of StGHs was analysed using Gene Structure Display Server software (ver. 2.0, http://gsds.gao-lab.org/) by comparing the genomic and coding sequences of all StGHs (Hu et al. 2015). The conserved motifs in StGH proteins were evaluated using MEME Suite (ver. 5.4.1, https://meme-suite.org/meme/index.html) using all default parameters except the maximum number of motifs, which was set to identify 10 motifs and the optimum width of motifs was set from 10 to 100 amino acids (Bailey et al. 2015). The conserved motifs were visualised using TBTools software (Chen et al. 2020).
Multiple sequence alignment and phylogenetic analysis
MUSCLE algorithm (Edgar 2004) was used to perform multiple sequence alignment of StGH and Arabidopsis GH proteins extracted from TAIR (https://www.arabidopsis.org/browse/genefamily/GlycosideHydrolase.jsp). The phylogenetic tree was constructed using Mega-X with the neighbour joining method with 1000 bootstrap replicates using p-distance method and pairwise deletion (Kumar et al. 2018). The phylogenetic tree was visualised using i-TOL (https://itol.embl.de/) (Letunic et al. 2021).
Analysis of cis-acting elements
Upstream sequences (2000 bp) of the transcription start site of StGHs were retrieved from PGSC and were analysed using PlantCare database (http://bioinformatics.psb.ugent.be/webtools/plantcare/html/) (Lescot et al. 2002). The cis-element distribution was then visualised using TBTools software (Chen et al. 2020).
Prediction of StGH protein–protein interaction network
All StGH protein sequences were added to the online server STRING (https://string-db.org/) (Szklarczyk et al. 2023) to predict protein–protein interactions using a confidence score of 0.700 (high confidence) and no more than 20 interactors. A separate network was created using only those proteins that showed co-expression using the same parameters but with no more than 50 interactors. The networks were visualised using Cytoscape software (Shannon et al. 2003).
Gene ontology analysis of StGHs
The OmicsBox software package (ver. 2.2.4, Biobam 2019) was used to annotate the coding regions of StGHs across three categories: (1) biological processes; (2) molecular function; and (3) cellular component.
Expression profiling analysis of GH genes
RNA-seq data of various developmental stages of potato (ERP000527) and in response to abiotic stresses, namely salt stress (SRP237987), drought stress (SRP056128), cold stress (SRP223879) and biotic stress (black scurf caused by Rhizoctonia solani) (SRP106262) were downloaded from NCBI-Sequence Read Archive (SRA). The differential expression analysis of the available data was done using the Trinity package (ver. 2.03) (Haas et al. 2013). Fragments per kb of transcript per million mapped (FPKM) reads was calculated using RSEM to evaluate the expression of different genes. EdgeR was used to check the differential expression levels of StGHs using two parameters: 4-fold change and a P-value of <0.001. Expression data under hormone treatments (IAA-10 μM, BAP-10 μM, GA3-50 μM, ABA-50 μM), biotic stress (Phytophthora infestans) and SAR elicitors (acibenzolar-S-methyl, BTH; DL-b-amino-n-butyric acid, BABA) were obtained from the RNA-Seq database of the Potato Genome Sequencing Consortium (2011). The differential expression results were visualised as heat maps using TBTools (Chen et al. 2020).
Prediction of miRNA targeting StGHs
The coding sequences of StGHs that were differentially expressed in salt and drought stress were used to predict putative target miRNAs using psRNATarget server (https://www.zhaolab.org/psRNATarget/analysis) (Dai and Zhao 2011) with default parameters. The interaction networks between StGHs and the miRNAs were created using Cytoscape software (Chen et al. 2020).
Plant materials and real time quantitatative PCR (qPCR) validation
S. tuberosum cv. Kufri jyoti plants were grown in a plant growth chamber (Percival Scientific, USA) at 25°C and 16/8 h (light/dark) at the Department of Biotechnology, Panjab University, Chandigarh, India. To verify the expression pattern of StGHs under drought stress, plants were grown in three sets with each set containing three biological replicates with the first set being treated as control under normal watering conditions, the second set was exposed to severe drought stress treatment for 3 days, and the third set was exposed to severe drought stress treatment for 3 days, after which they were rewatered to the same level as the control group and grown for an additional 3 days. For salt stress conditions, plants were grown in five sets containing three biological replicates each with the first set being treated as control while the other four sets were treated with 500 mM NaCl for 24 h, 48 h, 72 h and 96 h, respectively. Leaf tissues from these plants were excised at the described time points and were used for RNA isolation (Ghawana et al. 2011). Superscript III cDNA synthesis kit (Invitrogen USA) was used for cDNA synthesis. Primers for real time qPCR were designed using Primer 3 Plus (https://www.primer3plus.com/), followed by primer Tm standardisation. Elongation Factor 1-α (EF1-α) was used as the reference gene (Tang et al. 2017). Real time qPCR was performed in triplicates with control and stressed samples using Bio-Rad CFX96 Real-Time PCR detection system. PCR conditions used were 95°C for 7 min, followed by 40 cycles of 95°C for 20 s, Tm for 20 s, and 72°C for 20 s. The 2−ΔΔCT method was used to calculate the relative expression (Livak and Schmittgen 2001).
Statistical analysis
Data were analysed with multiple unpaired t-tests using GraphPad Prism (ver 2.2.2, GraphPad Software, Boston, MA, USA, www.graphpad.com). P-values of less than 0.05, 0.01 or 0.001 were considered significant differences from the control. All data were collected from three replicates.
Results
Identification and in silico characterisation of GH superfamily genes in S. tuberosum
A total of 366 GH genes were identified in the genome of S. tuberosum and were classified into 31 families based on the GH domains in the sequences. The genes were named according to their position on the chromosomes and the family they belong to (see Supplementary Table S1). Among them, GH17 family was the largest containing 68 genes, while GH2, GH37, GH39, GH43, GH77, GH81 and GH89 families consisted of only one gene each. The resulting genes varied substantially in CDS and protein lengths. The CDS length varied from 168 bp in StGH17.68 to 3456 bp in StGH38.2, while the number of amino acid residues varied from 55 in StGH17.68 to 1151 in StGH38.2 (Table S1). The molecular weight of the proteins varied from 9.85 kDa in StGH17.68 to 13.22 kDa in StGH38.2. The isoelectric point (pI) ranged from 3.74 in StGH32.9 to 9.81 in StGH28.42. Approximately 61.2% of the proteins were found to have the predicted pI less than 7.0 indicating that the majority of the proteins are most likely acidic. GRAVY values of the majority of the proteins were negative indicating strong hydrophilicity. The instability index of 71.5% proteins was less than 40%, suggesting that most of the proteins are stable (Table S2). The secondary structure of StGH proteins was made up of 26.37% α helix, 22.74% extended strand, 6.15% β turn and 44.73% random coil. StGHs (45.62%) were predicted to be located in extracellular space (Table S3). Gene ontology (GO) functional analysis revealed that the StGH gene superfamily was seen to include 178 GO categories across biological process, cellular component and molecular function. Main GO terms included ‘metabolic process (GO:0008152)’, ‘membrane (GO:0016020)’ and ‘catalytic activity (GO:0003824)’ under ‘Biological Process’, ‘Cellular Component’ and ‘Molecular Function’, respectively. The results were in accordance with the fact that GHs hydrolyse glycosidic linkages and are involved in cell wall polysaccharide metabolism. Various other GO terms like ‘cell wall biogenesis’, ‘response to stress’, ‘glucosidase activity’ and ‘polygalacturonase activity’ also conform with the already established roles of GHs according to previous studies (Supplementary Fig. S2).
Chromosomal mapping, gene duplication and syntenic analysis
The chromosomal coordinates of StGHs were retrieved from the PGSC database and were physically mapped on the chromosomes (Fig. 1). StGHs were unevenly located on 12 chromosomes (Chr) while eight genes (GH17.67, GH17.68, GH17.69, GH19.8, GH19.9, GH19.10, GH19.11 and GH28.50) were located on unplaced scaffolds. Chr1 contained the maximum number of 51 genes followed by Chr7 and Chr12 with a respective number of 39 and 38 genes. Chr3 and Chr4 contain 34 StGHs each, while Chr11, Chr6, Chr10 and Chr8 contained 33, 30, 23 and 22 StGHs, respectively. In addition, Chr6 and Chr5 contained 18 and 24 StGHs, respectively. The least number of StGHs was present on Chr9 with only 18 genes and Chr2 and Chr5 with 19 genes each. The length of chromosomes was not seen to be related to the number of genes present on them, even though Chr1 is the largest chromosome having the highest number of genes.
Distribution of glycoside hydrolase genes on S. tuberosum chromosomes. Chromosome numbers are shown at the left of each bar with StGHs on the right side represented in blue colour. The vertical scale is the size of chromosomes in megabases (Mb).
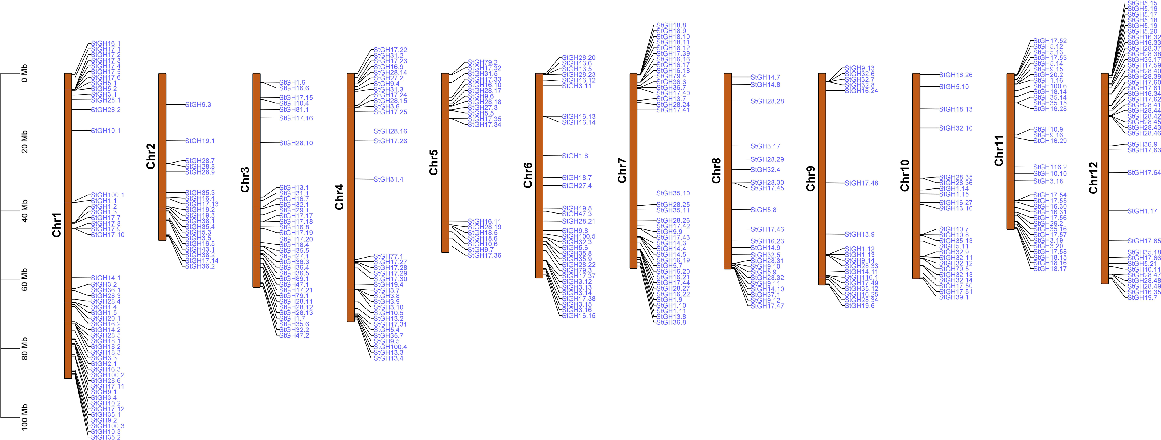
Gene duplication also accounted for the expansion of the StGH superfamily, as suggested by the significant amount of genetic variety within a family and genetic similarities between different families. According to our findings, 82 gene pairs were found to be duplicated among the 366 GHs in potato (Fig. 2a). It was also observed that a single gene was duplicated more than once. A total of 42 out of the 82 duplicated gene pairs were detected to be tandem duplications, which accounted for 51.21% of the duplication events, while the remaining 40 gene pairs were segmentally duplicated. The ratio of number of non-synonymous substitutions per non-synonymous site (Ka) to the number of synonymous substitutions per synonymous site (Ks) was calculated for the 82 gene pairs to analyse the selection pressure and adaptive evolution of GH gene family in potato. The results revealed that most of the duplicated genes had a Ka/Ks ratio of less than one indicating that most of the genes underwent purifying selection. However, one gene pair (StGH28.50 and StGH28.30) showed a Ka/Ks ratio of 0, indicating neutral selection and three gene pairs (StGH17.58 and StGH17.19, StGH18.10 and StGH18.11, StGH5.18 and StGH5.19) had a Ka/Ks ratio >1 demonstrating positive selection (Table S4). Out of 366 StGHs, 309 were found to have syntenic gene pairs in S. lycopersicum with two genes (StGH1.3 and StGH19.11) having more than one syntenic gene pair (Table S5). Different StGHs were seen to have a syntenic relation with the same tomato gene(StGH17.3, StGH17.4, StGH17.5 and StGH17.6) showed synteny with Solyc01g008620.3 (glucan endo-1,3-β-glucosidase A) (Fig. 2b).
Gene structure and protein motif analysis
Intron-exon structures were analysed to understand the structural features of StGHs (Supplementary Fig. S3). Potato GH gene family members demonstrated great variety in terms of both intron number and length. StGH17 has the least number of introns with zero introns in 13 coding sequences and a maximum of five introns in one member despite being the gene family with the highest members. However, this family still exhibited the maximum intron length with StGH17.3 (Class II 1,3-β-glucanase) having only one intron comprising 8792 bp. The highest number of introns was found to be 28 in StGH38.1 followed by StGH35 with 18 introns in three of its members (StGH35.4, StGH35.6 and StGH35.16). A total of 8.74% of the sequences have zero introns, 65.02% have 1–5 introns, 16.39% sequences have 6–10 introns, and 9.83% have more than 10 introns (Table S1). Protein sequences of all GH families containing three or more members were used for motif prediction (Supplementary Fig. S3). Motifs were found to be conserved within separate families. For instance, GH1 contained Motif 1 (Glyco_hydro_1) in 15 out of its 17 members, GH16 contained Motifs 1, 2, 3, 4 (Glyco_hydro_16) and Motifs 5, 8 (XET_C) in most of its members. All GH17 members had Glyco_hydro_17 and X8 motifs. GH 36 has raffinose synthase motifs in all its members. Every GH family was observed to contain motifs specific to that particular family (Table S6).
Phylogenetic analysis
We performed phylogenetic analysis of 366 GH proteins to understand the inter-family and intra-family evolutionary relationships among StGH proteins and their evolutionary links with Arabidopsis GHs. The multiple sequence alignment of GH proteins from potato and Arabidopsis was used to construct a neighbour-joining phylogenetic tree. Protein sequences of potato of most gene families such as GH1, GH16, GH17 and GH28 each shared the same clades with Arabidopsis proteins of the same family with a few exceptions like StGH1.12, which shared the same clade with GH17 members even though it has a glycoside hydrolase 1 domain (PF00232) and some GH17 members that share a closer evolutionary relationship with GH19 members as both families contain endo-hydrolases. However, not all the GH families were present on the same clade in both Arabidopsis and potato as the GH superfamily consists of a very diverse group of enzymes which makes it difficult for every family to remain confined to single and separate clades. This indicates evolutionary conservation between GHs of both potato and Arabidopsis as well as increasing evolutionary diversity, possibly due to gene duplication and divergence. Compared with Arabidopsis, S. tuberosum lacked sequences from GH51, GH63 and GH85. In contrast, Arabidopsis did not contain any sequences from GH29, GH39, GH89, GH100 and GH116, which were in potato (Fig. 3).
Analysis of cis-elements in potato GH genes
The probable cis-elements in the promoter region of 366 potato GHs were predicted using PlantCare. Our study revealed various cis-elements including stress-responsive regulatory elements found in StGHs included WUN-motif (wound-responsive), W box (wound-responsive, fungal elicitor responsive), WRE3 (wounding and pathogen response), TC-rich repeats (defense response), MYB (drought response), MYC (drought response), STRE (heat, osmotic stress, low pH, nutrient starvation stresses response), DRE (drought response), MBS (drought response), LTR (low temperature responsive), as-1 (drought response), ARE (anoxic response), GC-motif (anoxic response) and CCAAT-box (MYBHv1 binding). Phytohormone-responsive elements included TGA-element (auxin responsive), AuxRR-core (auxin responsive), AuxRE (auxin responsive), TATC-box (GA-responsive), P-box (GA-responsive), GARE-motif (GA-responsive), ABRE (ABA-responsive), ABRE3a (ABA-responsive), ERE (ethylene-responsive), TGACG-motif (MeJA-responsive), CGTCA-motif (MeJA-responsive), TCA-element (SA responsive) and SARE (SA responsive). Plant growth related elements included circadian (circadian control), HD-Zip 1 (differentiation of palisade mesophyll cells), GCN4_motif (endosperm expression), AAGAA-motif (secondary xylem development), O2-site (zein metabolism) and RY-element (seed-specific regulation) and light-responsive elements (Box4, ATCT-motif, ATC-motif, I-box, chs-CMA1a, GA-motif, TCT-motif, LAMP-element, BOX II, Sp1, GATA-motif, Gap-box, CAG-motif, TCCC-motif, G-box, MRE, AT1-motif, AAAC-motif, GT1-motif, ACE, AE-box). Almost all the StGHs were observed to have at least one light-responsive element, making them the most abundant (3940) cis-element, followed by MYC (1502), MYB (1411), MeJA-responsive (775) and ABA-responsive (723) elements, respectively (Fig. 4).
Analysis of tissue-specific expression of StGH family genes
StGH expression levels were analysed in 16 potato tissues (flower, leaf, petiole, shoot apex, stem, stolon, young tuber, mature tuber, root, stamen, water stressed leaf, tuber pith, tuber peel, whole in vitro plant, tuber sprout and tuber cortex). Almost all StGHs were revealed to be expressed in at least one of the tissues except 10 StGHs (StGH3.16, StGH6.14, StGH16.31, StGH17.18, StGH17.26, StGH28.9, StGH28.25, StGH28.35, StGH28.43 and StGH32.4), which showed no expression at all in any of the tissues. Out of the expressing families, 12 families (StGH2, StGH13, StGH20, StGH27, StGH37, StGH39, StGH47, StGH77, StGH79, StGH81, StGH100 and StGH116) showed expression in all 16 tissues of potato. Among the observed genes, GH13 had a higher overall level of expression in nearly all tissues, whereas GH10 showed a lower expression level across all tissues. The maximum expression levels in tuber pith, tuber peel and tuber sprout were observed in StGH9.6, whereas StGH13.4 showed the highest expression in mature tuber and tuber cortex. The genes StGH16.3, StGH16.30, StGH16.5, StGH14.1, and StGH19.2 were expressed the most in young tuber, root, leaf, whole in vitro plant and water stressed leaf, respectively. StGH16.18 showed the highest expression level in shoot apex and stolon. StGH19.6 exhibited the highest expression levels in petiole and stem, while StGH35.13 exhibited the highest expression levels in flower and stamen (Supplementary Fig. S4).
Analysis of StGH expression levels under various abiotic stresses
Expression levels of StGHs were studied using the SRA data of S. tuberosum under the abiotic stresses of drought, salt and cold. According to the SRA data, 84 out of 366 StGHs were found to have at least 4-fold differential expression under drought stress, where 25 genes were upregulated after 3 days of drought stress, out of which 24 genes were downregulated 3 days after rewatering except StGH13.4, which was observed to be even more upregulated after rewatering (Fig. 5a). Interestingly, some of the downregulated genes were not able to fully recover their expression levels even 3 days after rewatering. StGH35.17 and StGH17.25 showed a significant 2-fold and 4.88-fold increase in expression levels when exposed to 3 days of drought stress. StGH28.17 and StGH28.19 were seen to show 2.9-fold and 2.01-fold downregulation in expression levels when subjected to 3 days of drought stress. In the case of salt stress, 121 StGHs were revealed to be differentially expressed and the overall expression of genes showed a trend of initial upregulation in response to salt stress with 80 genes being upregulated, followed by a decrease in expression after 48 h of salt stress. However, some genes continued to be upregulated even after 72 h of salt stress, indicating their importance in response to prolonged salt stress. Interestingly, 69 StGHs showed higher expression levels even after 96 h of salt stress when compared to the control expression levels (Fig. 5b). A total of 27 StGHs were identified to be differentially expressed under cold stress exposure after three months, where 17 genes were seen to be upregulated while the other 10 genes were downregulated (Supplementary Fig. S5).
Transcriptome analysis of StGHs under abiotic stresses. (a) Expression pattern of StGHs differentially expressed under drought stress. DRT, drought stress treatment for 3 days; RWT, rewatering for 3 days. (b) Expression pattern of StGHs differentially expressed under salt stress. (c) Venn diagram showing common differentially expressed StGHs under both drought and salt stress.
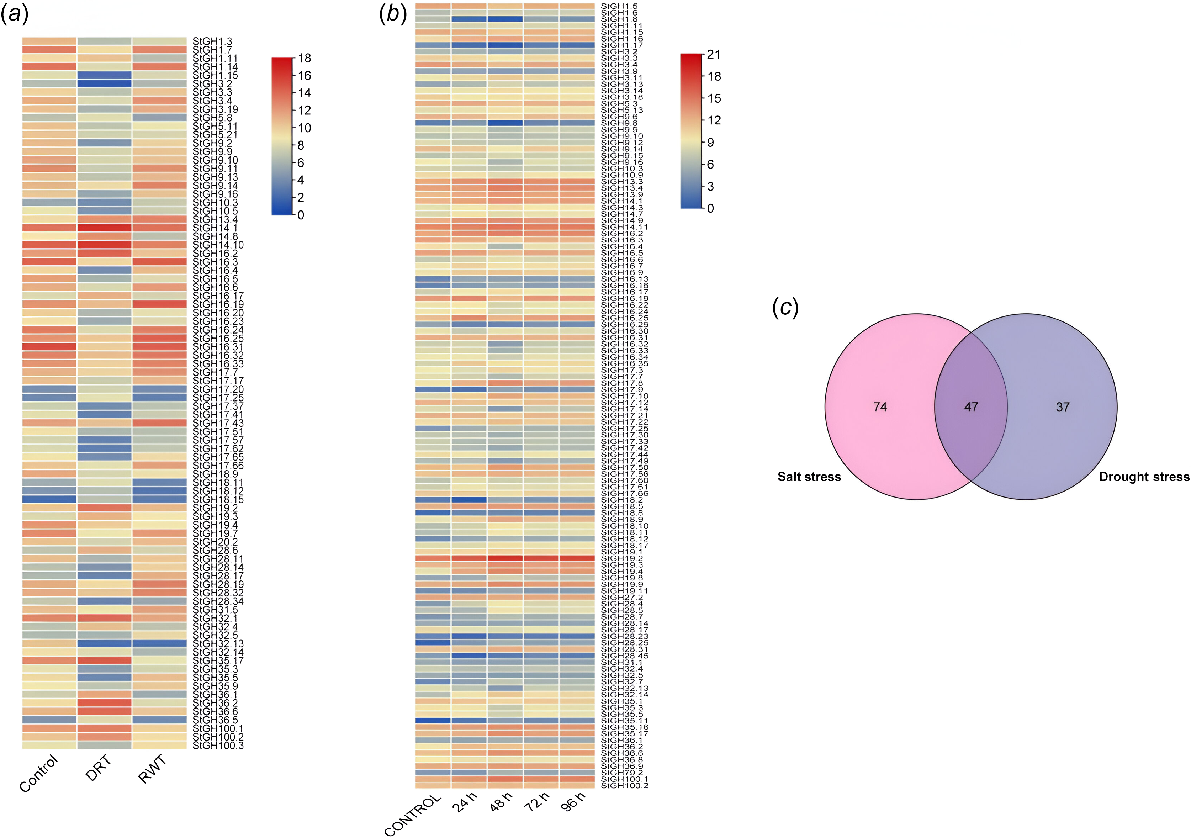
Analysis of StGH expression levels under various biotic stresses and hormone treatments
Expression levels of StGHs were studied using the SRA data of S. tuberosum under biotic stresses (black scurf caused by R. solani and late blight caused by P. infestans) and hormone treatment (6-benzylaminopurine, BAP; abscisic acid, ABA; indole-3-acetic acid, IAA; and gibberellic acid, GA3). Fifty out of 366 StGHs showed difference in expression levels when infected by R. solani, where 17 genes were upregulated after 3 days of R. solani infection and 23 genes were upregulated even after 8 days of R. solani infection when compared to plants with no infection (Supplementary Fig. S5). In the case of the other dataset, only the genes with FPKM values greater than 1 were selected. The number of downregulated StGHs was markedly higher than that of upregulated ones when subjected to hormone treatments. Different numbers of StGHs were found to have at least 1.2-fold upregulation when exposed to hormone stress conditions: BAP (11 StGHs); ABA (23 StGHs); IAA (five StGHs); and GA3 (six StGHs). Notably, StGH9.3 showed a 3.76-fold upregulation when treated with BAP and 2.4-fold upregulation under GA3 treatment, while StGH19.2 and StGH36.2 showed a 3.54-fold and 4.84-fold upregulation when treated with ABA, respectively. Additionally, 6, 13 and one StGHs had at least 1.2-fold upregulation when exposed to P. infestans, BABA and BTH, respectively. StGHs with at least 1.2-fold change in expression levels are depicted as heatmaps (Supplementary Fig. S6). The expression levels of all genes under hormone treatment, P. infestans infection and SAR elicitors were quite low.
Real time qPCR analysis of salt and drought stress-responsive StGHs
To validate the modulated expression of StGHs under drought and salt stress conditions, a total of six differentially expressed genes were selected for each stress (Table S7). In the case of drought stress, the expression levels of StGH16.24, StGH17.25, StGH28.17, StGH28.19, StGH35.17 and StGH36.2 genes were analysed. The results suggested differential response of each gene during drought stress and rewatering. StGH17.25 and StGH16.24 were upregulated in response to drought stress and remained upregulated even after rewatering, indicating their potential role in drought tolerance. In contrast, the expression of StGH36.2, StGH28.19 and StGH28.17 was downregulated in response to drought stress and remain downregulated after rewatering, suggesting their potential role in drought susceptibility. StGH35.17 showed a significantly higher expression level when the plants were subjected to 3 days of drought stress but the levels decreased substantially when rewatered (Fig. 6a).
Expression profiles of StGHs differentially expressed under abiotic stress as analysed by real time qPCR. (a) Relative expression of StGHs under drought stress with control, drought stress for 3 days (DRT_3d), and rewatering for 3 days (RWT_3d). (b) Relative expression of StGHs under salt stress with control, 24 h, 48 h, 72 h and 96 h of 500 mM NaCl treatment. Error bars result from three biological replicates. *P < 0.05; **P < 0.01; ***P < 0.001; ****P < 0.0001.
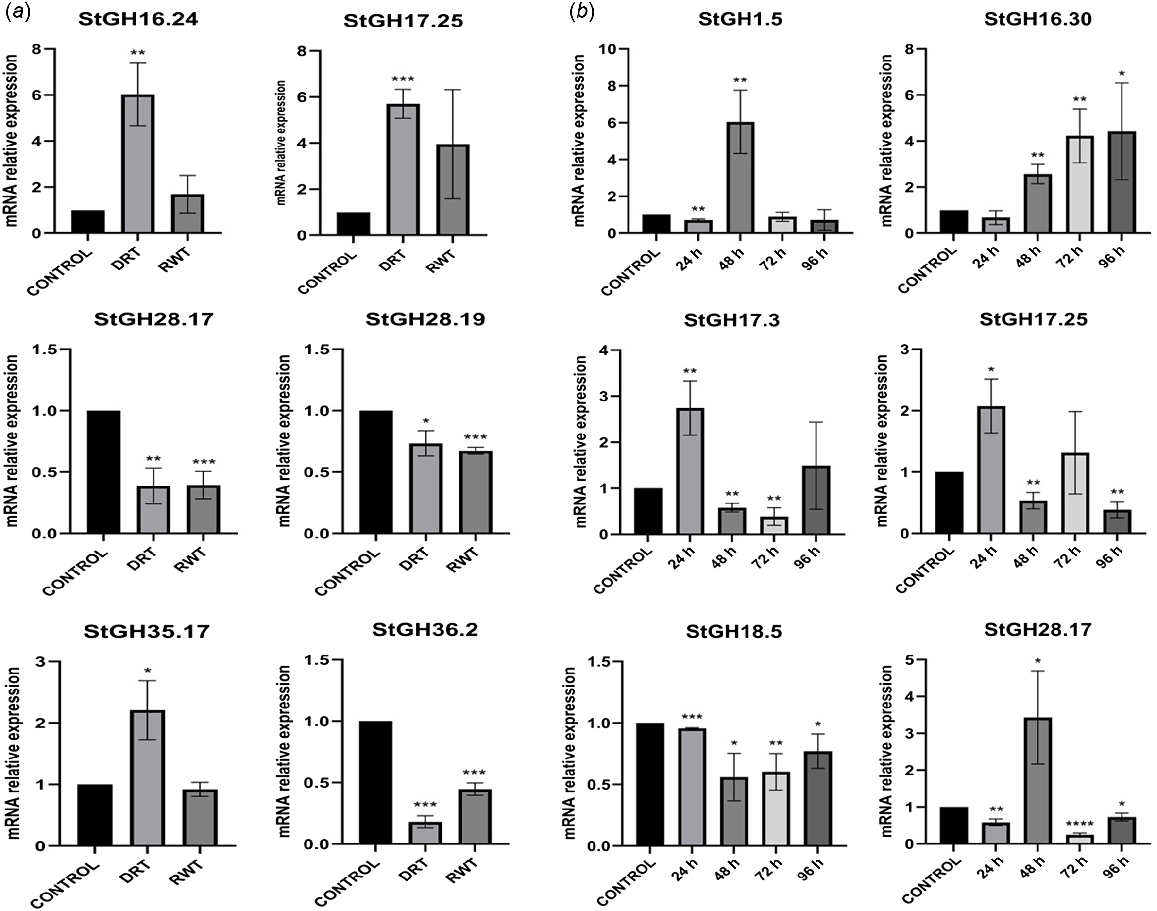
In the case of salt stress, StGH1.5, StGH17.3, StGH16.30, StGH17.25, StGH18.5 and StGH28.17 were selected for real time qPCR (Fig. 6b). StGH17.3 and StGH17.25 showed significant upregulation at 24 h. However, this upregulation was not sustained and decreased sharply at 48 h. StGH1.5 showed a slight downregulation at 24 h followed by a very significant upregulation at 48 h. StGH16.30 showed a continuous increase in expression levels after 24 h until 96 h of salt stress exposure. The data revealed that different genes have distinct patterns of expression under salt stress, with some showing an initial increase followed by a decrease, others showing a continuous increase, and yet others showing a decrease followed by a partial recovery. Further studies are needed to understand the specific functions of these StGHs in the plant response to drought and salt stress.
Protein–protein interaction analysis
A protein regulatory network was created among StGH proteins and with other potato proteins to understand the genetic interaction relationship (Fig. 7a). A total of 395 pairwise interactions were found among the StGH proteins and 181 additional interactions were found when other proteins from potato were taken into consideration. StGH31.3 (α-glucosidase) was observed to have 49 interactions with other StGH proteins and other potato proteins. StGH16.27 (xyloglucan endotransglycosylase) shows interaction with 16 non-GH potato proteins. StGH20.1 (β-hexosaminidase 1) and StGH20.2 (β-hexosaminidase 2) interact with each other and 31 other GH proteins. All GH13 members except GH13.8 were found to be interacting with GlgP (α-1,4 glucan phosphorylase L-1 isozyme), which plays a vital role in maintaining glucose homeostasis. In addition, the same proteins were checked for co-expressing proteins, which resulted in 559 interactions of StGH proteins with other potato GH and non-GH proteins (Fig. 7b). StGH16.27 showed co-expression with 32 non-GH proteins of potato, all of which also exhibited co-expression among themselves. Two StGH5 members (StGH5.14 and StGH5.21) were also found to be co-expressing with five members of the StGH18 family (Class III and V chitinases).
Protein–protein interaction network of StGH proteins with a high confidence score of 0.700. (a) Interaction network of StGH proteins with no more than 20 interactors within S. tuberosum. (b) Co-expression network of StGH proteins with no more than 50 interactors within S. tuberosum. Green nodes, StGH proteins; purple nodes, other non-GH potato proteins.
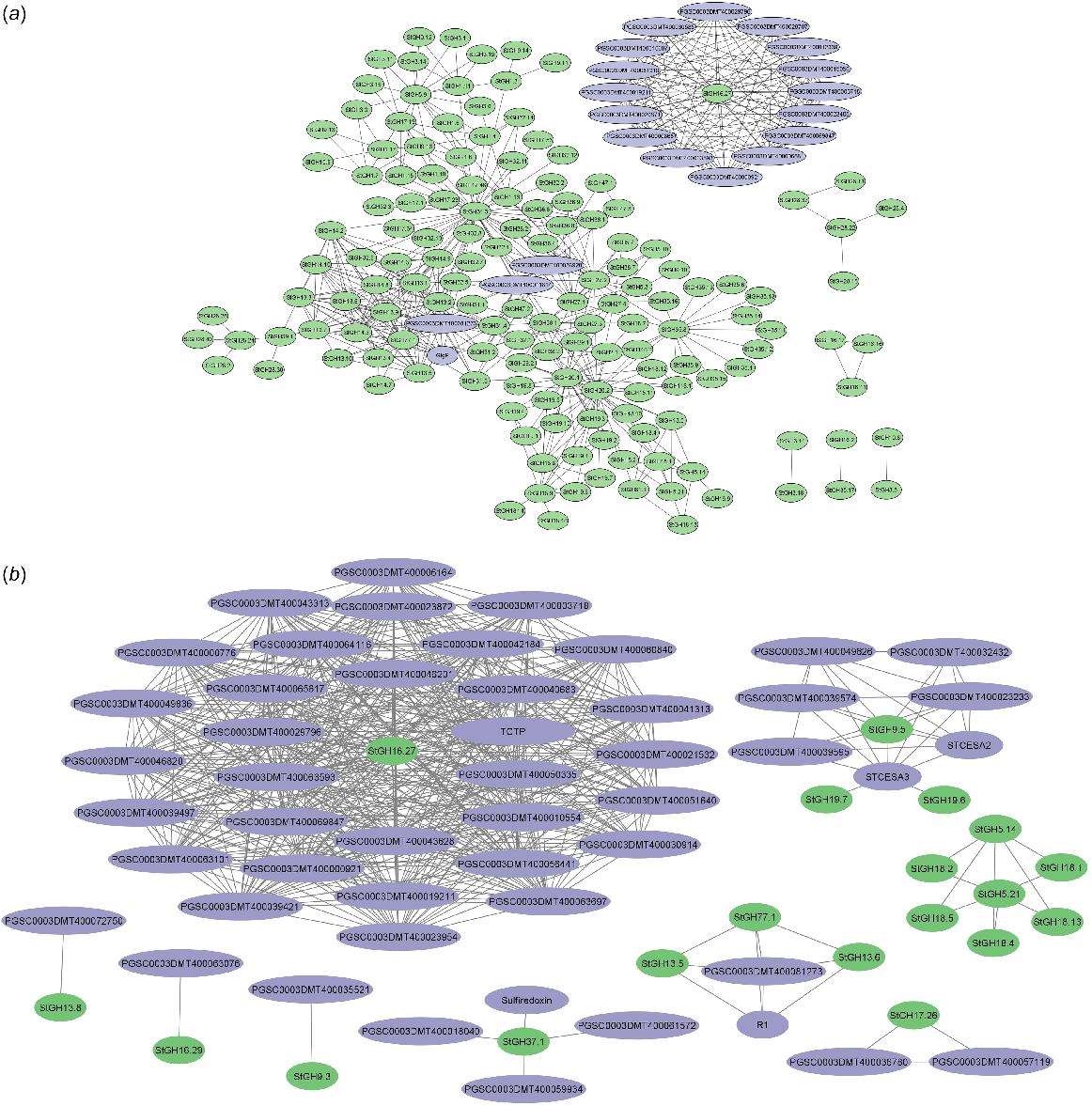
Analysis of microRNAs targeting StGHs
Potential interactions between 343 earlier reported potato miRNAs (Kozomara et al. 2019) and StGHs differentially expressed under salt and drought stress were studied. We found 393 interactions between potato miRNAs and differentially expressed StGHs under drought stress (Fig. 8a), where 80 StGHs interacted with 204 miRNAs, and 627 interactions between potato miRNAs and differentially expressed StGHs under salt stress, where 111 StGHs interacted with 227 miRNAs (Fig. 8b). A network was constructed using Cytoscape software, which revealed that StGH35.11 and StGH3.9 were the most targeted genes by S. tuberosum miRNAs, as determined by analysing connection distribution. miR1886h was found to target 13 different genes, out of which eight genes were differentially expressed under both salt and drought stress.
Discussion
GHs are known to have significant involvement in various biological processes, including starch hydrolysis, cell signalling, plant defence mechanisms, cell wall biosynthesis, cell wall metabolism and polysaccharide metabolism (Henrissat et al. 2001; Cosgrove 2005; Minic and Jouanin 2006). In this study, we identified 366 GH genes in S. tuberosum and divided them into 31 different families based on the domains present. The GHs superfamily exhibits considerable variation in the number of genes across different plant species with poplar (Populus spp.) having the highest count of 600 genes (Geisler-Lee et al. 2006), followed by rice (Oryza sativa) with 465 genes (Yokoyama and Nishitani 2004), sorghum (Sorghum bicolor) with 404 genes (Tyler et al. 2010), Arabidopsis with 390 genes (Henrissat et al. 2001) and Brachypodium with the lowest count of 356 genes (Tyler et al. 2010). A total of 20 GH1s (β-glucosidases) were identified and analysed in S. lycopersicum by Wei et al. (2022). GHs account for 0.93% of the total coding genes in potato. GH17 was found to contain the maximum number of genes; i.e. 69 in potato, which was comparable to rice having 68 genes in GH17. Potato and rice having similar distributions of gene families even when the latter is a monocot depicts the conservation of glucoside hydrolases during evolution. However, GH28 is the largest family with 66 genes in Arabidopsis. Subcellular localisation prediction revealed that a substantial number of StGH proteins were located in extracellular space, which in high likelihood denotes the plant cell wall as it has already been studied and reported that GHs play a vital role in cell wall metabolism and reorganisation and degradation of cell wall polysaccharides (Minic and Jouanin 2006).
The analysis of chromosome mapping unveiled an asymmetrical distribution of the GH superfamily throughout the 12 potato chromosomes, wherein Chr1 exhibited the highest number of genes (51 genes). The majority of StGHs were observed to be located in the telomeric area of the chromosomes, as opposed to the centromeric region. The process of gene duplication is a prominent catalyst for the augmentation of genetic complexity and variety, ultimately resulting in the origin of novel genes and exerting a pivotal influence on the evolution of genomes. The evolutionary fate of duplicated genes is influenced by their level of sequence similarity, with the potential outcomes including the retention of ancestral function, acquisition of a new function, or the loss of an existing function. Many duplicated StGH genes were seen to be present on different chromosomes but still had the same function and belonged to the same family. The majority of duplicated genes had a Ka/Ks ratio below 0, indicating the presence of purifying selection. A total of three gene pairs (StGH17.58 and StGH17.19, StGH18.10 and StGH18.11, StGH5.18 and StGH5.19) had a Ka/Ks ratio greater than 0, suggesting positive selection. Conversely, one duplicated gene pair (StGH28.50 and StGH28.30) demonstrated neutral selection. Syntenic analysis unveiled the conserved genes between S. tuberosum and S. lycopersicum suggesting divergence from same ancestry. A total of 309 StGHs were observed to have syntenic pairs with S. lycopersicum genes; 17 out of these 309 genes were located on a different chromosome when compared to their syntenic pairs in tomato indicating inter-chromosomal rearrangements during the process of evolution. StGH17.67, StGH19.8, StGH19.9, StGH19.11 and StGH28.50 located on unplaced scaffolds in potato were found to have syntenic relationship with tomato genes located on Chr1, Chr10, Chr10, Chr10 and Chr8, respectively. StGH19.11 was found to have six different syntenic pairs in tomato, all of which were located on the same chromosome, suggesting gene duplication events. This alludes to the possibility of assigning these genes to the chromosome number of their respective syntenic pair. These findings suggest that potato and tomato share a close evolutionary relationship based on synteny analysis with respect to GHs.
The number and organisation of introns and exons frequently serve as key factors in driving changes in gene functions during evolutionary processes. It has been previously reported that genes containing a lower number of introns demonstrate expedited activation when faced with environmental challenges (Xu et al. 2012). Gene structure analysis revealed a considerable variation among the intron-exon structures of StGHs. Overall, the least number of introns were found to be present in StGH17 with 13 genes having zero introns. Motif analysis of StGH proteins revealed the presence of conserved motifs within each family.
The cis-regulatory elements situated upstream of the transcription start site were identified and segmented into three different categories: (1) stress responsive; (2) phytohormone responsive; and (3) plant growth-related. Light-responsive elements were found to be present in large numbers in almost all StGHs but we observed that these findings are somewhat limited due to the lack of adequate data. Among the phytohormone-responsive elements, MeJA and ABA-responsive cis-elements, were the most prevalent in potato. GHs have been speculated to be involved in phytohormone activation. It has been reported that β-glucosidases can hydrolyse biologically inactive ABA to produce active ABA as dehydration rapidly induces polymerisation of an Arabidopsis β-glucosidase (Lee et al. 2006). Another β-D-glucoside was observed to cleave biologically inactive cytokinin conjugates, releasing active cytokinin in Zea mays (Brzobohatý et al. 1993). It was observed that in sugar beet (Beta vulgaris), all the BvXTHs were ubiquitously expressed and were upregulated or downregulated in response to multiple phytohormones (Gao et al. 2024).
Differential expression studies using RNA-seq data revealed the possible involvement of numerous StGHs in various abiotic and biotic stresses with GH16, GH17, GH18, GH19 and GH28 members having the highest members that show change in their expression levels under stress. GH18, GH19 (chitinases) and GH17 (β-1,3-glucanase) have been observed to work in a synergistic manner to stimulate anti-fungal activity as these are co-ordinately induced by ethylene or by pathogen attack in plants (Schlumbaum et al. 1986). Co-expression of chitinase and glucanase genes in transgenic tobacco resulted in enhanced protection against the fungal pathogen Cercospora nicotianae (Zhu et al. 1994). Abiotic stresses such as salinity, drought and cold can lead to enhanced expression of chitinases in plants (Yeh et al. 2000; Pinheiro et al. 2001). As GH16 and GH28 members are involved in cell wall remodelling, they play an important role in acclimatising the plant to various stresses as cell wall is the primary defence of plants against environmental stresses (Sasidharan et al. 2011). GH13 members (α-amylases) were found to have an overall higher expression in all potato tissues when compared to other families as amylases are found in almost all reserve tissues when starch mobilisation occurs (Beck and Ziegler 1989; Fincher 1989). We observed six StGHs that were found differentially expressed in response to drought stress. Real time qPCR results revealed that StGH35.17, a β-galactosidase, showed a remarkable increase in expression when exposed to 3 days of drought stress, which validates the presence of ABRE, MYB and MYC cis-regulatory elements. It has been reported that β-galactosidases respond greatly to drought stress, with Ibbgal4 showing a highly significant increase in expression levels (Hou et al. 2021). The expression levels of StGH17.25 (glucan endo-1,3-β-D-glucosidase) and StGH16.24 (xyloglucan endotransglucosylase/hydrolase 12) were detected to be increased after 3 days of drought stress, with the expression levels still remaining upregulated after rewatering the plants. This was in accordance with the study by Fu et al. (2024), in which the ectopic expression of ZmXTH30 in Arabidopsis seedlings improved their growth under drought stress by lowering reactive oxygen species levels and increasing antioxidant enzyme activity, highlighting the significance of ZmXTH30 in enhancing plant drought tolerance. The differentially expressed glucan endo-1,3-β-D-glucosidase also played a role in regulating glyco-metabolism and enhancing energy catabolism during seedling growth under salt stress in alfalfa (Gao et al. 2024). Two StGHs (StGH28.19 and StGH28.17) showed decreased expression levels when exposed to drought stress, which did not increase even after rewatering. Both these genes encode polygalacturonases and are present in the plant cell wall. The role of these genes in drought stress resistance might be due to their ability of cell wall remodelling and regulation of cell wall elasticity and extensibility.
Overexpression of PG-1 β (a subunit of polygalacturonase) also led to reduced tolerance to abiotic stress in rice (Liu et al. 2014). We also performed real time qPCR analysis of six genes that were differentially expressed in response to salt stress. StGH16.30 (xyloglucan endotransglucosylase-hydrolase, XTH6) showed a continuous increase in expression levels when exposed to increasing time points of salt stress after 24 h, with a significant increase 96 h after being subjected to 500 mM NaCl. XTH expression has been observed to improve abiotic stress tolerance in Arabidopsis and tomato (Choi et al. 2011; Han et al. 2016; Han et al. 2017). Qiao et al. (2022) observed that the transcription levels of some VvXTHs in the leaves and roots exhibited the significant changes, indicating that VvXTHs are likely involved in grapevine (Vitis vinifera) responses to drought and salt stress. StGH17.25 (glucan endo-1,3-β-D-glucosidase) and StGH17.3 (1,3-β-glucanase) showed the highest expression levels after 24 h of salt stress while StGH28.17 (polygalacturonase) was seen to have the highest expression levels after 48 h of salt stress exposure. However, StGH18.5 (endochitinase) was observed to be downregulated at all time points of salt stress. In contrast, the upregulation of VvChit-IV, an endochitinase, was linked to a substantial increase in H2O2 and proline levels in grapevine cell cultures, without a significant change in malondialdehyde content. This suggests that the H2O2 signalling network may initiate a priming effect to enhance the defence response against environmental stress (Ben-Amar et al. 2022). The study’s focus on abiotic stress responses is based on expression data, but the physiological relevance of these expression changes and their direct impact on plant stress tolerance require further experimental validation. Phenotypic assays and stress tolerance tests in transgenic plants would provide stronger evidence of the functional roles of GH genes under stress conditions.
We also analysed potential interactions between potato miRNAs and StGH genes differentially expressing under salt and drought stress. Potato miRNAs were observed to have 393 and 627 interactions with StGHs differentially expressed under drought and salt stress, respectively. The overexpression of miR156, which interacts with StGH3.19 (β-D-glucan exohydrolase), StGH9.16 (Endo-1,4-β-D-glucanase), StGH20.2 (β-hexosaminidase) and StGH100.2 (β-fructofuranosidase), was observed to contribute in inducing drought resistance in alfalfa (Arshad et al. 2018). miR393, which interacts with three StGH16.3 (xyloglucan endotransglucosylase/hydrolase 1), StGH17.60 and StGH17.61 (both are elicitor-inducible β-1,3-glucanase) has been suggested to be involved in helping Arabidopsis adapt to salt stress by attenuating auxin signalling pathways (Denver and Ullah 2019). miR167, miR393 and miR397 were reported to regulate the expression of their target gene to combat drought stress in maize (Das and Mondal 2021). In the case of salt stress, miR167 was observed to be upregulated in downregulated in maize but upregulated in Arabidopsis (Khraiwesh et al. 2012). Cumulatively, these results led us to infer that StGHs may significantly contribute in biotic and abiotic stress response, phytohormone signalling and cellular metabolism in S. tuberosum. In conclusion, while our bioinformatics and computational analyses offer a comprehensive overview of the GH gene family in potato, there still remains the need for experimental validation to confirm these findings. Future research should focus on functional assays, gene editing and phenotypic analyses to bridge the gap between computational predictions and biological functions.
Conclusions and future perspectives
A genome-wide analysis of potato revealed 366 GH superfamily genes which were divided into 31 different families with GH17 being the largest one. Members of the same GH family were observed to have similar motif compositions. Cis-regulatory element analysis unveiled that StGH promoter regions are abundant in LTR, MYB, MYC, MeJARE and ABRE, suggesting their potential role in abiotic stress resistance. Using previous RNA-seq, numerous StGHs were observed to be expressed in almost all potato tissues and differentially expressed under various abiotic and biotic stresses such as salinity, drought, cold, black scurf, etc., some of which were confirmed by real time qPCR. Many of the StGHs differentially expressed under drought and salt stress were found to be interacting with various miRNAs. Our findings lay the groundwork for a deeper understanding of the functional characterisation of GHs in potato. In addition, insights gained from our study can build a foundation for the family Solanaceae to explore the diverse functions and the physiological roles GHs play in various plants. Future research on GHs in potato could explore their roles in stress tolerance and crop yield enhancement. Investigating the functional diversity and regulatory mechanisms of these genes under various conditions can lead to the development of stress-resilient potato varieties. Synteny analysis with tomato suggests potential for cross-species genetic manipulation. Additionally, examining the interactions between GHs and miRNAs could provide new insights into their regulatory networks, contributing to novel crop improvement strategies.
Conflicts of interest
The authors declare that they have no known competing financial interests or personal relationships that could have appeared to influence the work reported in this paper.
Declaration of funding
Authors are thankful to Department of Biotechnology, Government of India for the research grant to Kashmir Singh.
Author contributions
KS: conceptualisation, funding acquisition, investigation, methodology, project administration, supervision, resources. Aiana: data curation, formal analysis, software, validation, visualisation, roles/writing – original draft, writing – review and editing. HC: validation, visualisation, roles/writing – original draft.
Acknowledgements
The authors are thankful to the Department of Biotechnology, Panjab University, Chandigarh for providing research facilities. HC is thankful to the Council of Scientific and Industrial Research (CSIR) for awarding junior and senior research fellowship. Aiana is grateful to the University grants commission (U.G.C.), New Delhi for providing the junior and senior research fellowship.
References
Arshad M, Gruber MY, Hannoufa A (2018) Transcriptome analysis of microRNA156 overexpression alfalfa roots under drought stress. Scientific Reports 8(1), 9363.
| Crossref | Google Scholar | PubMed |
Atkinson RG, Schröder R, Hallett IC, Cohen D, MacRae EA (2002) Overexpression of polygalacturonase in transgenic apple trees leads to a range of novel phenotypes involving changes in cell adhesion. Plant Physiology 129(1), 122-133.
| Crossref | Google Scholar | PubMed |
Bailey TL, Johnson J, Grant CE, Noble WS (2015) The MEME Suite. Nucleic Acids Research 43(W1), W39-W49.
| Crossref | Google Scholar | PubMed |
Beck E, Ziegler P (1989) Biosynthesis and degradation of starch in higher plants. Annual Review of Plant Physiology and Plant Molecular Biology 40(1), 95-117.
| Crossref | Google Scholar |
Ben-Amar A, Allel D, Mliki A (2022) Up-regulation of a stress-responsive endochitinase VvChit-IV in grapevine cell cultures improves in vitro stress tolerance. Protoplasma 259(5), 1189-1203.
| Crossref | Google Scholar | PubMed |
Bi H, Liu Z, Liu S, Qiao W, Zhang K, Zhao M, Wang D (2024) Genome-wide analysis of wheat xyloglucan endotransglucosylase/hydrolase (XTH) gene family revealed TaXTH17 involved in abiotic stress responses. BMC Plant Biology 24(1), 640.
| Crossref | Google Scholar | PubMed |
Biobam (2019) OmicsBox Bioinformatics Made Easy. BioBam Bioinformatics. Available at https://www.biobam.com/omicsbox [accessed 3 March 2019]
Brogue K, Chet I, Holliday M, Cressman R, Biddle P, Knowlton S, Mauvais CJ, Broglie R (1991) Transgenic plants with enhanced resistance to the fungal pathogen Rhizoctonia solani. Science 254(5035), 1194-1197.
| Crossref | Google Scholar | PubMed |
Brzobohatý B, Moore I, Kristoffersen P, Bako L, Campos N, Schell J, Palme K (1993) Release of active cytokinin by a β-glucosidase localized to the maize root meristem. Science 262(5136), 1051-1054.
| Crossref | Google Scholar | PubMed |
Buscaill P, Sanguankiattichai N, Lee YJ, Kourelis J, Preston G, van der Hoorn RAL (2021) Agromonas: a rapid disease assay for Pseudomonas syringae growth in agroinfiltrated leaves. The Plant Journal 105(3), 831-840.
| Crossref | Google Scholar | PubMed |
Chandrasekar B, Wanke A, Wawra S, Saake P, Mahdi L, Charura N, Neidert M, Poschmann G, Malisic M, Thiele M, Stühler K, Dama M, Pauly M, Zuccaro A (2022) Fungi hijack a ubiquitous plant apoplastic endoglucanase to release a ROS scavenging β-glucan decasaccharide to subvert immune responses. The Plant Cell 34(7), 2765-2784.
| Crossref | Google Scholar | PubMed |
Chauhan H, Aiana, Singh K (2023) Genome-wide identification of 2-oxoglutarate and Fe(II)-dependent dioxygenase family genes and their expression profiling under drought and salt stress in potato. PeerJ 11, e16449.
| Crossref | Google Scholar | PubMed |
Chen C, Chen H, Zhang Y, Thomas HR, Frank MH, He Y, Xia R (2020) TBtools: an integrative toolkit developed for interactive analyses of big biological data. Molecular Plant 13(8), 1194-1202.
| Crossref | Google Scholar | PubMed |
Choi JY, Seo YS, Kim SJ, Kim WT, Shin JS (2011) Constitutive expression of CaXTH3, a hot pepper xyloglucan endotransglucosylase/hydrolase, enhanced tolerance to salt and drought stresses without phenotypic defects in tomato plants (Solanum lycopersicum cv. Dotaerang). Plant Cell Reports 30(5), 867-877.
| Crossref | Google Scholar |
Cosgrove DJ (2005) Growth of the plant cell wall. Nature Reviews Molecular Cell Biology 6(11), 850-861.
| Crossref | Google Scholar | PubMed |
Cosgrove DJ, Li LC, Cho HT, Hoffmann-Benning S, Moore RC, Blecker D (2002) The growing world of expansins. Plant and Cell Physiology 43(12), 1436-1444.
| Crossref | Google Scholar | PubMed |
Coutinho PM, Henrissat B (1999) Life with No Sugars? Journal of Molecular Microbiology and Biotechnology 1(2), 307-308.
| Google Scholar | PubMed |
Dai X, Zhao PX (2011) psRNATarget: a plant small RNA target analysis server. Nucleic Acids Research 39(Web Server issue), W155-W159.
| Crossref | Google Scholar |
Das R, Mondal SK (2021) Plant miRNAs: biogenesis and its functional validation to combat drought stress with special focus on maize. Plant Gene 27, 100294.
| Crossref | Google Scholar |
Deng Z, Yang Z, Liu X, Dai X, Zhang J, Deng K (2023) Genome-wide identification and expression analysis of C3H zinc finger family in potato (Solanum tuberosum L.). International Journal of Molecular Sciences 24(16), 12888.
| Crossref | Google Scholar | PubMed |
Denver JB, Ullah H (2019) miR393s regulate salt stress response pathway in Arabidopsis thaliana through scaffold protein RACK1A mediated ABA signaling pathways. Plant Signaling & Behavior 14(6), 1600394.
| Crossref | Google Scholar | PubMed |
Edgar RC (2004) MUSCLE: multiple sequence alignment with high accuracy and high throughput. Nucleic Acids Research 32(5), 1792-1797.
| Crossref | Google Scholar | PubMed |
Fincher GB (1989) Molecular and cellular biology associated with endosperm mobilization in germinating cereal grains. Annual Review of Plant Physiology and Plant Molecular Biology 40(1), 305-346.
| Crossref | Google Scholar |
Fu W, Fan D, Zhang Y (2024) Genome-wide identification and characterization of xyloglucan endotransglucosylase/hydrolase gene family in maize (Zea mays L.) and the function of ZmXTH30 in response to drought stress. Environmental and Experimental Botany 222, 105744.
| Crossref | Google Scholar |
Gao Y, Wang L, Li D, Qi D, Fang F, Luo Y, Zhang H, Zhang S (2024) Genome-wide characterization of the xyloglucan endotransglucosylase/hydrolase family genes and their response to plant hormone in sugar beet. Plant Physiology and Biochemistry 206, 108239.
| Crossref | Google Scholar | PubMed |
Geisler-Lee J, Geisler M, Coutinho PM, Segerman B, Nishikubo N, Takahashi J, Aspeborg H, Djerbi S, Master E, Andersson-Gunnerås S, et al. (2006) Poplar carbohydrate-active enzymes. gene identification and expression analyses. Plant Physiology 140(3), 946-962.
| Crossref | Google Scholar | PubMed |
Geourjon C, Deléage G (1995) SOPMA: significant improvements in protein secondary structure prediction by consensus prediction from multiple alignments. Computer Applications in the Biosciences 11(6), 681-684.
| Crossref | Google Scholar | PubMed |
Ghawana S, Paul A, Kumar H, et al. (2011) An RNA isolation system for plant tissues rich in secondary metabolites. BMC Research Notes 4, 85.
| Crossref | Google Scholar |
Grison R, Grezes-Besset B, Schneider M, Lucante N, Olsen L, Leguay JJ, Toppan A (1996) Field tolerance to fungal pathogens of Brassica napus constitutively expressing a chimeric chitinase gene. Nature Biotechnology 14(5), 643-646.
| Crossref | Google Scholar | PubMed |
Haas BJ, Papanicolaou A, Yassour M, Grabherr M, Blood PD, Bowden J, Couger MB, Eccles D, Li B, Lieber M, et al. (2013) De novo transcript sequence reconstruction from RNA-Seq using the trinity platform for reference generation and analysis. Nature Protocols 8(8), 1494-1512.
| Crossref | Google Scholar | PubMed |
Han Y, Ban Q, Hou Y, Meng K, Suo J, Rao J (2016) Isolation and characterization of two persimmon xyloglucan endotransglycosylase/hydrolase (XTH) genes that have divergent functions in cell wall modification and fruit postharvest softening. Frontiers in Plant Science 7, 624.
| Crossref | Google Scholar | PubMed |
Han Y, Han S, Ban Q, He Y, Jin M, Rao J (2017) Overexpression of persimmon DkXTH1 enhanced tolerance to abiotic stress and delayed fruit softening in transgenic plants. Plant Cell Reports 36(4), 583-596.
| Crossref | Google Scholar |
Henrissat B (1991) A classification of glycosyl hydrolases based on amino acid sequence similarities. Biochemical Journal 280(2), 309-316.
| Crossref | Google Scholar |
Henrissat B, Coutinho PM, Davies GJ (2001) A census of carbohydrate-active enzymes in the genome of Arabidopsis thaliana. Plant Molecular Biology 47(1–2), 55-72.
| Crossref | Google Scholar | PubMed |
Hou F, Du T, Qin Z, Xu T, Li A, Dong S, Ma D, Li Z, Wang Q, Zhang L (2021) Genome-wide in silico identification and expression analysis of beta-galactosidase family members in sweetpotato [Ipomoea batatas (L.) Lam.]. BMC Genomics 22(1), 140.
| Crossref | Google Scholar |
Hu B, Jin J, Guo AY, Zhang H, Luo J, Gao G (2015) GSDS 2.0: an upgraded gene feature visualization server. Bioinformatics 31(8), 1296-1297.
| Crossref | Google Scholar | PubMed |
Khraiwesh B, Zhu JK, Zhu J (2012) Role of miRNAs and siRNAs in biotic and abiotic stress responses of plants. Biochimica et Biophysica Acta 1819(2), 137-148.
| Crossref | Google Scholar | PubMed |
Kotake T, Tsuchiya K, Aohara T, Konishi T, Kaneko S, Igarashi K, Samejima M, Tsumuraya Y (2006) An α-L-arabinofuranosidase/β-D-xylosidase from immature seeds of radish (Raphanus sativus L.). Journal of Experimental Botany 57(10), 2353-2362.
| Crossref | Google Scholar | PubMed |
Kong H, Song J, Ma S, Yang J, Shao Z, Li Q, Li Z, Xie Z, Yang P, Cao Y (2024) Genome-wide identification and expression analysis of the glycosyl hydrolase family 1 genes in Medicago sativa revealed their potential roles in response to multiple abiotic stresses. BMC Genomics 25(1), 20.
| Crossref | Google Scholar | PubMed |
Kozomara A, Birgaoanu M, Griffiths-Jones S (2019) miRBase: from microRNA sequences to function. Nucleic Acids Research 47(D1), D155-D162.
| Crossref | Google Scholar | PubMed |
Kumar S, Stecher G, Li M, Knyaz C, Tamura K, Mega X (2018) MEGA X: molecular evolutionary genetics analysis across computing platforms. Molecular Biology and Evolution 35(6), 1547-1549.
| Crossref | Google Scholar | PubMed |
Lashbrook CC (2005) New insights into cell wall disassembly during fruit ripening. Stewart Postharvest Review 1, 1-18.
| Crossref | Google Scholar |
Lashbrook CC, Gonzalez-Bosch C, Bennett AB (1994) Two divergent endo-β-1,4-glucanase genes exhibit overlapping expression in ripening fruit and abscising flowers. The Plant Cell 6(10), 1485-1493.
| Crossref | Google Scholar | PubMed |
Lee KH, Piao HL, Kim HY, Choi SM, Jiang F, Hartung W, Hwang I, Kwak JM, Lee IJ, Hwang I (2006) Activation of glucosidase via stress-induced polymerization rapidly increases active pools of abscisic acid. Cell 126(6), 1109-1120.
| Crossref | Google Scholar | PubMed |
Lescot M, Déhais P, Thijs G, Marchal K, Moreau Y, Van de Peer Y, Rouzé P, Rombauts S (2002) PlantCARE, a database of plant cis-acting regulatory elements and a portal to tools for in silico analysis of promoter sequences. Nucleic Acids Research 30(1), 325-327.
| Crossref | Google Scholar | PubMed |
Letunic I, Khedkar S, Bork P (2021) SMART: recent updates, new developments and status in 2020. Nucleic Acids Research 49(D1), D458-D460.
| Crossref | Google Scholar |
Lin W, Anuratha CS, Datta K, Potrykus I, Muthukrishnan S, Datta SK (1995) Genetic engineering of rice for resistance to sheath blight. Nature Biotechnology 13(7), 686-691.
| Crossref | Google Scholar |
Liu H, Ma Y, Chen N, Guo S, Liu H, Guo X, Chong K, Xu Y (2014) Overexpression of stress-inducible OsBURP16, the β subunit of polygalacturonase 1, decreases pectin content and cell adhesion and increases abiotic stress sensitivity in rice. Plant, Cell and Environment 37(5), 1144-1158.
| Crossref | Google Scholar | PubMed |
Liu S, Liu R, Lv J, Feng Z, Wei F, Zhao L, Zhang Y, Zhu H, Feng H (2023) The glycoside hydrolase 28 member VdEPG1 is a virulence factor of Verticillium dahliae and interacts with the jasmonic acid pathway-related gene GhOPR9. Molecular Plant Pathology 24(10), 1238-1255.
| Crossref | Google Scholar | PubMed |
Livak KJ, Schmittgen TD (2001) Analysis of relative gene expression data using real-time quantitative PCR and the 2-ΔΔCT method. Methods 25(4), 402-408.
| Crossref | Google Scholar | PubMed |
Lu S, Wang J, Chitsaz F, Derbyshire MK, Geer RC, Gonzales NR, Gwadz M, Hurwitz DI, Marchler GH, Song JS, et al. (2020) CDD/SPARCLE: the conserved domain database in 2020. Nucleic Acids Research 48(D1), D265-D268.
| Crossref | Google Scholar |
Minic Z, Jouanin L (2006) Plant glycoside hydrolases involved in cell wall polysaccharide degradation. Plant Physiology and Biochemistry 44(7–9), 435-449.
| Crossref | Google Scholar | PubMed |
Minic Z, Rihouey C, Do CT, Lerouge P, Jouanin L (2004) Purification and characterization of enzymes exhibiting β-D-xylosidase activities in stem tissues of Arabidopsis. Plant Physiology 135(2), 867-878.
| Crossref | Google Scholar | PubMed |
Minic Z, Do CT, Rihouey C, Morin H, Lerouge P, Jouanin L (2006) Purification, functional characterization, cloning, and identification of mutants of a seed-specific arabinan hydrolase in Arabidopsis. Journal of Experimental Botany 57(10), 2339-2351.
| Crossref | Google Scholar | PubMed |
Minic Z, Jamet E, Négroni L, Arsene der Garabedian P, Zivy M, Jouanin L (2007) A sub-proteome of Arabidopsis thaliana mature stems trapped on Concanavalin A is enriched in cell wall glycoside hydrolase. Journal of Experimental Botany 58(10), 2503-2512.
| Crossref | Google Scholar | PubMed |
Mistry J, Chuguransky S, Williams L, Qureshi M, Salazar GA, Sonnhammer ELL, Tosatto SCE, Paladin L, Raj S, Richardson LJ, Finn RD, Bateman A (2021) Pfam: the protein families database in 2021. Nucleic Acids Research 49(D1), D412-D419.
| Crossref | Google Scholar |
Paysan-Lafosse T, Blum M, Chuguransky S, Grego T, Pinto BL, Salazar GA, Bileschi ML, Bork P, Bridge A, Colwell L, et al. (2023) InterPro in 2022. Nucleic Acids Research 51(D1), D418-D427.
| Crossref | Google Scholar |
Pinheiro C, Chaves MM, Ricardo CP (2001) Alterations in carbon and nitrogen metabolism induced by water deficit in the stems and leaves of Lupinus albus L. Journal of Experimental Botany 52(358), 1063-1070.
| Crossref | Google Scholar | PubMed |
Potato Genome Sequencing Consortium (2011) Genome sequence and analysis of the tuber crop potato. Nature 475(7355), 189-195.
| Crossref | Google Scholar |
Qiao T, Zhang L, Yu Y, Pang Y, Tang X, Wang X, Li L, Li B, Sun Q (2022) Identification and expression analysis of xyloglucan endotransglucosylase/hydrolase (XTH) family in grapevine (Vitis vinifera L.). PeerJ 10, e13546.
| Crossref | Google Scholar |
Roitsch T, Balibrea ME, Hofmann M, Proels R, Sinha AK (2003) Extracellular invertase: key metabolic enzyme and PR protein. Journal of Experimental Botany 54(382), 513-524.
| Crossref | Google Scholar | PubMed |
Sasidharan R, Voesenek LACJ, Pierik R (2011) Cell wall modifying proteins mediate plant acclimatization to biotic and abiotic stresses. Critical Reviews in Plant Sciences 30(6), 548-562.
| Crossref | Google Scholar |
Schlumbaum A, Mauch F, Vögeli U, Boller T (1986) Plant chitinases are potent inhibitors of fungal growth. Nature 324(6095), 365-367.
| Crossref | Google Scholar |
Shannon P, Markiel A, Ozier O, Baliga NS, Wang JT, Ramage D, Amin N, Schwikowski B, Ideker T (2003) Cytoscape: a software environment for integrated models of biomolecular interaction networks. Genome Research 13(11), 2498-2504.
| Crossref | Google Scholar | PubMed |
Sherson SM, Alford HL, Forbes SM, Wallace G, Smith SM (2003) Roles of cell-wall invertases and monosaccharide transporters in the growth and development of arabidopsis. Journal of Experimental Botany 54(382), 525-531.
| Crossref | Google Scholar | PubMed |
Shumayla, Sharma S, Kumar R, Mendu V, Singh K, Upadhyay SK (2016) Genomic dissection and expression profiling revealed functional divergence in Triticum aestivum leucine rich repeat receptor like kinases (TaLRRKs). Frontiers in Plant Science 7, 1374.
| Crossref | Google Scholar | PubMed |
Spooner DM, Ghislain M, Simon R, Jansky SH, Gavrilenko T (2014) Systematics, diversity, genetics, and evolution of wild and cultivated potatoes. Botanical Review 80, 283-383.
| Crossref | Google Scholar |
Sueldo DJ, Godson A, Kaschani F, Krahn D, Kessenbrock T, Buscaill P, Schofield CJ, Kaiser M, van Der Hoorn RAL (2024) Activity-based proteomics uncovers suppressed hydrolases and a neo-functionalised antibacterial enzyme at the plant–pathogen interface. New Phytologist 241(1), 394-408.
| Crossref | Google Scholar | PubMed |
Szklarczyk D, Kirsch R, Koutrouli M, Nastou K, Mehryary F, Hachilif R, Gable AL, Fang T, Doncheva NT, Pyysalo S, et al. (2023) The STRING Database in 2023: protein–protein association networks and functional enrichment analyses for any sequenced genome of interest. Nucleic Acids Research 51(D1), D638-D646.
| Crossref | Google Scholar |
Taylor MA, Ross HA, McRae D, Stewart D, Roberts I, Duncan G, Wright F, Millam S, Davies HV (2000) A potato α-glucosidase gene encodes a glycoprotein-processing α-glucosidase II-like activity. Demonstration of enzyme activity and effects of down-regulation in transgenic plants. The Plant Journal 24(3), 305-316.
| Crossref | Google Scholar | PubMed |
Tang X, Zhang N, Si H, Calderón-Urrea A (2017) Selection and validation of reference genes for RT-qPCR analysis in potato under abiotic stress. Plant Methods 13, 85.
| Crossref | Google Scholar | PubMed |
Thumuluri V, Almagro Armenteros JJ, Johansen AR, Nielsen H, Winther O (2022) DeepLoc 2.0: multi-label subcellular localization prediction using protein language models. Nucleic Acids Research 50(W1), W228-W234.
| Crossref | Google Scholar | PubMed |
Tyler L, Bragg JN, Wu J, Yang X, Tuskan GA, Vogel JP (2010) Annotation and comparative analysis of the glycoside hydrolase genes in Brachypodium Distachyon. BMC Genomics 11, 600.
| Crossref | Google Scholar |
Wang S, Sun X, Miao X, Mo F, Liu T, Chen Y (2023) Genome-wide analysis and expression profiling of the glutathione peroxidase-like enzyme gene family in Solanum tuberosum. International Journal of Molecular Sciences 24(13), 11078.
| Crossref | Google Scholar | PubMed |
Wei J, Chen Q, Lin J, Chen F, Chen R, Liu H, Chu P, Lu Z, Li S, Yu G (2022) Genome-wide identification and expression analysis of tomato glycoside hydrolase family 1 β-glucosidase genes in response to abiotic stresses. Biotechnology & Biotechnological Equipment 36(1), 268-280.
| Crossref | Google Scholar |
Xu G, Guo C, Shan H, Kong H (2012) Divergence of duplicate genes in exon–intron structure. Proceedings of the National Academy of Sciences of the United States of America 109(4), 1187-1192.
| Crossref | Google Scholar |
Yeh S, Moffatt BA, Griffith M, Xiong F, Yang DSC, Wiseman SB, Sarhan F, Danyluk J, Xue YQ, Hew CL, Doherty-Kirby A, Lajoie G (2000) Chitinase genes responsive to cold encode antifreeze proteins in winter cereals. Plant Physiology 124(3), 1251-1264.
| Crossref | Google Scholar | PubMed |
Yin Y, Cui D, Sun H, Guan P, Zhang H, Chi Q, Jiao Z (2024) Genome-wide identification, characterization, and expression analysis of four subgroup members of the GH13 family in wheat (Triticum aestivum L.). International Journal of Molecular Sciences 25(6), 3399.
| Crossref | Google Scholar | PubMed |
Yokoyama R, Nishitani K (2004) Genomic basis for cell-wall diversity in plants. A comparative approach to gene families in rice and Arabidopsis. Plant and Cell Physiology 45(9), 1111-1121.
| Crossref | Google Scholar | PubMed |
Zhang C, Zhang L, Wang D, Ma H, Liu B, Shi Z, Ma X, Chen Y, Chen Q (2018) Evolutionary history of the glycoside hydrolase 3 (GH3) family based on the sequenced genomes of 48 plants and identification of jasmonic acid-related GH3 proteins in Solanum tuberosum. International Journal of Molecular Sciences 19(7), 1850.
| Crossref | Google Scholar | PubMed |
Zhu Q, Maher EA, Masoud S, Dixon RA, Lamb CJ (1994) Enhanced protection against fungal attack by constitutive co-expression of chitinase and glucanase genes in transgenic tobacco. Nature Biotechnology 12(8), 807-812.
| Crossref | Google Scholar |