Effects of iron oxide nanoparticles (Fe3O4) and salinity on growth, photosynthesis, antioxidant activity and distribution of mineral elements in wheat (Triticum aestivum)
Vladimir D. Kreslavski


A Institute of Basic Biological Problems, Russian Academy of Sciences, Pushchino 142290, Russia.
B International Research Centre for Environmental Membrane Biology, Foshan University, Foshan 528000, China.
C K.A. Timiryazev Institute of Plant Physiology, Russian Academy of Sciences, Moscow 127276, Russia.
D Faculty of Engineering and Natural Sciences, Bahcesehir University, Istanbul, Turkey.
E School of Biological Science, University of Western Australia, Crawley, WA 6009, Australia.
Functional Plant Biology 50(11) 932-940 https://doi.org/10.1071/FP23085
Submitted: 7 May 2023 Accepted: 27 July 2023 Published: 14 August 2023
Abstract
Soil salinisation is one of the main abiotic stresses decreasing crop productivity. Here, we show that the plant treatment with iron oxide (Fe3O4) nanoparticles (NPs) may be a promising solution for reducing the negative impact of soil salinity on plant performance. For this purpose, effects of the NPs on growth, photosynthesis, pro-/antioxidant, redox balance and the content of mineral elements in 19-day-old wheat (Triticum aestivum) plants under soil salinity were studied. Seed treatment with NPs (200 and 500 mg L−1) enhanced growth and photosynthetic rate in leaves. Moderate salinity stress (150 mM NaCl) led to a decrease in plant biomass as well as the rate of photosynthesis and PSII activity; leaf photosynthetic characteristics were also suppressed by lower (75 mM NaCl) salinity treatment. However, seed pre-treatment with the NPs partially eliminated the negative effect of the salt on growth, PSII activity and photosynthesis. Also, we observed a decrease in the content of malondialdehyde (MDA) and an increase in ascorbate and total peroxidase activity in the plant leaves upon combined treatment with NaCl and the NPs compared with treatment with NaCl alone. The combined treatment with the NPs and salinity also led to a noticeable increase in the content of Fe and Mn in the shoot. It was concluded that Fe3O4 NPs can enhance plant growth by improving photosynthetic characteristics, antioxidant balance and the availability of iron and manganese ions, under conditions of soil salinisation.
Keywords: antioxidants, growth, iron oxide, nanoparticles, photosynthesis, soil salinity, stress, Triticum aestivum L.
Introduction
In recent decades, abiotic stressors such as extreme temperatures, salinity, drought, flooding and various toxic compounds have been increasingly affecting plant growth and development as well as product quality (Qamer et al. 2021). To mitigate these detrimental effects, it is necessary to develop new biotechnologies that can increase plant tolerance to various stressors. One of the most promising new strategies is the application of synthetic nanoparticles (NPs) as bioactive stimulants and micro-fertilisers (Abhilash 2010; Ahmad and Akhtar 2019; Usman et al. 2020). Such application was shown to increase productivity of various crops by improving seed germination, enhancing synthesis of various metabolites, particularly proteins, and improving the balance between antioxidants and prooxidants (Bakhtiari et al. 2015; Rui et al. 2016; Amjad et al. 2018; Singh et al. 2021). They also reduce environmental risks of different chemical fertilisers and increase fertiliser use efficiency (Baruah and Dutta 2009; Bakhtiari et al. 2015). In this context, seeds priming is considered as more environmentally friendly due much less contamination of soil while using this technology. Also, in a contrast to micronutrients applied in ionic form, micronutrients delivered by NPs penetrate in the plants more gradually and can be considered as analogues of slow-release fertilisers.
NPs have unique properties that depend on such characteristics as their size, shape, chemical composition and pH of the medium (Wang et al. 2011; Elfeky et al. 2013); all these factors will affect their efficiency as both micro-fertilisers and regulators of plant adaptive responses to hostile environment. The advantages of NPs are their high activity, effective penetration into various plant organs and relatively small amounts when used compared to ionic and chelate forms (Ghafari and Razmjoo 2013). As such, beneficial effects of NPs applications on crop yield (Torney et al. 2007; Singh et al. 2021) and photosynthesis (Liu et al. 2017; Mohamed et al. 2017; Feng et al. 2022) were reported. However, the patterns, pathways of action and targets of NPs under stress conditions on photosynthesis and other metabolic processes are poorly understood.
Iron is an essential micronutrient that plays a key role in the regulation of plant growth and development, being involved in numerous processes of metabolism and biosynthesis of chlorophyll, photosynthesis and dark respiration (Iannone et al. 2016; Siddiqi et al. 2016; Rizwan et al. 2019). As biological iron availability for plants is strongly affected by soil pH, iron deficiency is common phenomenon in plants growing on many soils (Mazaherinia et al. 2010). Iron uptake is also severely affected in plants under stress. Thus, application of nano-iron in various forms (Fe, FeO, Fe2O3, Fe3O4) under stress conditions may provide an efficient way of mitigating symptoms of iron deficiency and enhance plant performance in hostile soils.
One of major constraints on crop production is soil salinity (Pan et al. 2021). Accumulation of salts such as NaCl is the most important reason for the reduction of cultivable land (Ahmad and Akhtar 2019). However, the decrease in photosynthetic rate and crop productivity due to salt stress can be avoided by applying NPs. For example, application of FeO NPs to soil at all tested concentrations markedly increased K+ uptake by wheat (Triticum aestivum) plants, but at the same time decreased the amount of Na+ accumulated in the shoot (Manzoor et al. 2021). Also, application of Ag-based NPs improved germination percentage, increased fresh and dry weight of seedlings and finally provided better survival and growth of Lathyrus sativus under saline conditions (Hojjat 2019), and beneficial effects of ZnO NPs on growth of Lupinus termis were also reported (Abdel Latef et al. 2017).
Earlier, we showed that treatment of seeds with iron oxide (Fe3O4) NPs led to an increase in photosynthetic rate and antioxidant potential of wheat plants (Feng et al. 2022). Importantly, no negative effects of Fe3O4 NPs were reported over a wide range of concentrations tested (Rizwan et al. 2019; Feng et al. 2022). Thus, we have postulated that Fe3O4 NPs may be suitable to ameliorate detrimental effects of salinity stress on plant photosynthesis (and overall growth). Experiments were conducted on wheat, the second most important staple food crop in the world. Wheat is considered to be sensitive to salinity, with its salinity threshold being 6 dS m−1 (Maas and Hoffman 1977) and most varieties dying or failing to set-up grain at salinities exceeding 150 mM NaCl (Cuin et al. 2009). The latter occur due to numerous constraints imposed by salinity stress (van Zelm et al. 2020; Zhao et al. 2020). One of them is osmotic stress (the lowering of the external water potential) that compromises a plant’s ability to take up water. Prolonged exposures to salinity also result in a major nutritional imbalance and elemental (mostly Na+ and Cl−) toxicities. As a result, plant ability for CO2 assimilation is compromised, due to both stomatal and non-stomatal limitations (Pan et al. 2021). Finally, salinity stress results in a rapid increase in amount of reactive oxygen species (ROS) in plant tissues, causing a major damage to key cellular structures (Ozgur et al. 2013) and disturbing plant ion homeostasis by direct activation of numerous ion channels in cellular membranes (Demidchik 2015; Shabala et al. 2016). Accordingly, plant tolerance to salinity is conferred by a large number of complementary mechanisms involved in control of Na+ uptake, long-distant transport, and sequestration; K+ retention; optimising stomata operation; and maintain redox balance (Wu et al. 2018; van Zelm et al. 2020; Zhao et al. 2020).
In the light of above, this work aimed to elucidate impact of salinity and potential ameliorative effects of NP on growth, photosynthesis, redox balance and ion content in wheat plants. Our working hypothesis was that NP treatment may ameliorate detrimental effects of salinity by affecting ROS-mediated ionic homeostasis in plant tissues.
Materials and methods
Plant material and growing conditions
Seeds of wheat (Triticum aestivum L. cv. Moscow 35) were obtained from Rusagro Group of Companies (Moscow, Russia). The seeds were sterilised in 5% hydrogen peroxide solution for 10 min followed by a thorough rinsing with deionised water. Iron oxide nanoparticles (Fe3O4 NPs) sized 80–110 nm (99% purity; Advanced Powder Technology, Tomsk, Russia) were used in the study. The particles were prepared in double-distilled water and subjected to 20 min treatment with an ultrasonic generator UZG13-0.1/22 (Ultrasonic Technologies, St. Petersburg, Russia) at a frequency of 22 kHz. Working concentrations of Fe3O4 varied from 40 to 500 mg L−1.
Wheat seeds were treated with Fe3O4 solution while shaking in tubes for 6 h with a shaker (Rotamix RM-1; ELMI, Riga, Latvia). In some experiments, iron citrate FeC6H5O7 (200 mg L−1) was used instead of Fe3O4 NPs. As a control sample, seeds were not treated with Fe3O4 NPs but were shaken in an aqueous solution for 6 h or in iron citrate solution in the same concentrations as Fe3O4 NPs. Seeds were then washed and placed on a moist filter paper in Petri dishes, which were placed in a thermostat at 23 ± 0.5°C for 2 days under weak illumination (5 μmol quanta m−2 s−1).
For planting, uniform seedlings of about 1 cm in size were selected and transplanted into 1.2 L plastic pots filled with a soil mixture containing a mixture of top and bottom peat, washed sand, and lime flour (Garden Retail Service, Ivanteevka, Russia), The soil contained 250 mg kg−1 nitrogen, 400 mg kg−1 phosphorus, 500 mg kg−1 potassium, and micronutrients (pH 6.5). Plants were then grown for 18 days under white LED lamps at 24 ± 1°C and irradiation levels of 300 μmol (photons) m−2 s−1 at 16-h photoperiod. Six days after soaking the NaCl solution (either 75 or 150 mM) was added to pots. Two vessels with 32 plants were used for each treatment. At the end of experiment, plant photosynthetic and biochemical characteristics were measured, and plants were harvested for an agronomical assessment (fresh and dry weight). The youngest fully developed leaf (leaf 3) was used for analysis.
Photosynthetic activity
To assess PSII activity, fluorescence parameters that characterise the state of the photosynthetic apparatus were determined. They were calculated on the basis of Chl a fluorescence induction curves, by analysing the data of rapid fluorescence induction kinetics in dark adapted leaves (JIP-test). To record the induction curves, we used the fluorometer described previously (Kreslavski et al. 2014), which allows recording of chl a fluorescence induction curves at 1-ms intervals and at 10-μs intervals within the first ms.
The curves were recorded in the 1-s interval at a saturation light intensity of 5000 μmol quanta m−2 s−1 at λm = 455 nm. Based on the obtained induction curves, fluorescence parameters were calculated: DI0/RC and FV/F0, which denote quantum efficiency of energy dissipation in PSII and PSII maximum quantum yield, respectively. Also, the PSII performance index PIABS was calculated.
where M0 is the average value of the initial slope of the relative variable fluorescence of chl a. M0 reflects the rate of closure of PSII reaction centres, and VJ is the relative level of fluorescence in phase J after 2 min.
The rates of photosynthesis, respiration, and transpiration as well as the value of stomatal conductance were determined using a LCPro+ infra-red gas analyser from ADC Bio-Scientific Ltd. (Hoddesdon, UK) connected to a 6.25 cm2 leaf chamber. Photosynthetic rate was measured at a saturating light intensity of 1200 μmol quanta m−2 s−1, and respiration rate was measured in the dark. Leaf samples were placed in the chamber so that the portion between the central and upper leaf regions was in the study area, and then light with an intensity of 1200 μmol quanta m−2 s−1 was turned on.
Photosynthetic pigments
Concentrations of chl a and b and carotenoids (Car) in pigment extracts were determined spectrophotometrically in 80% acetone as described by Lichtenthaler (1987), using a spectrophotometer (Genesis 10UV, Thermo Spectronic, Waltham, MA, USA).
Lipid peroxidation and enzyme activity
The intensity of lipid peroxidation was assessed by measuring malonic dialdehyde (MDA) content using the method (Uchiyama and Mihara 1978). A total of 0.25 g of leaves were grinded in a mortar with 2 mL of chilled 100 mM K-phosphate buffer (pH 7.5) containing 0.1 mM ethylenediaminetetraacetic acid (EDTA). The homogenate was then centrifuged for 15 min at 13 000g at 4°C. The reaction mixture consisted of equal proportions (v/v) of the supernatant and a solution that contained 0.8% thiobarbituric acid and 20% trichloroacetic acid. The mixture was heated in boiling water for 15 min, then quickly cooled with ice and centrifuged at 15 000g for 5 min.
Ascorbate peroxidase (APX) activity was determined according to the method described by Veljovic-Jovanovic et al. (2006) with some modifications by measuring the absorbance decrease at 290 nm (ε = 2.8 mM−1 cm−1) in the reaction mixture (2 mL) containing 50 mM K-phosphate (pH 7.2), 0.3 mM ascorbate, 0.1 mM H2O2 and 100 μL extract. A mixture without ascorbate was used for a comparison. The activity of guaiacol-dependent peroxidase (POD) was determined as described elsewhere (Chance and Maehly 1955; Maehly 2006). Enzyme activity was measured by an increase in absorbance at 470 nm (ε = 26.6 mM−1 cm−1) compared with controls without the enzyme. Estimation of specific enzyme activity was performed on the basis of a crude biomass of plant material.
Proline content and protein determination
Determination of the amount of free proline (Pro) was carried out according to the method of Bates et al. (1973). Dry plant material (20 mg) was homogenised in 4 mL of 3% sulfosalicylic acid. After centrifugation at 15 000g for 15 min, 2 mL of the supernatant was mixed with 2 mL of glacial acetic acid and 2 mL of ninhydrin solution (2.5% ninhydrin was dissolved in a mixture of glacial acetic acid and 6 M phosphoric acid, 3/2, v/v). The reaction mixture was incubated at 100°С for 1 h. The reaction was stopped by placing the samples in an ice bath, then 4 mL of toluene was added, shaken, and the absorbance of the toluene fraction was measured at 520 nm. Pro content (μg g−1 DW) was determined from a calibration curve.
Total protein content was determined using the method of Bradford (1976).
Leaf elemental content
To determine the elements, the plant material was dried for 2 days at 85°C and then manually grinded in a mortar. The content of Ca, K, Na, Cl, Mg, P, S, Mn and Fe in various wheat tissues was determined by X-ray fluorescence method (S6 Jaguar Spectrometer, Bruker, FRG, Billerica, MA, USA) according to the protocol described by Towett et al. (2013) and presented as % of DW.
Statistics
At least 12 plants for each treatment were used to calculate growth rates. Between 6 and 12 leaves selected from two growth containers were used to measure chlorophyll fluorescence and to estimate enzymatic activity, malonic dialdehyde and pigment content. The data presented in the tables and figures are mean values ± s.d., where n represents a pooled sample of three to four biological replicates. Significance of differences between several groups was performed using a one-factor analysis of variance (ANOVA) and Tukey’s multiple comparison test. For data processing and statistical analysis, we used R 3.5.0 (R Foundation for Statistical Computing, Vienna, Austria, 2017).
Results
Growth, leaf gas exchange, and pigment composition
Salinity stress has reduced plant biomass in a dose-dependent manner; seed pre-treatment with NPs has reversed this effect (Fig. 1a). Salt treatment also significantly decreased activity of leaf photosynthetic machinery as evident in a decline in chlorophyll fluorescence characteristics such as PSII maximal quantum yield (Fv/Fm ratio; Fig. 2a) and PSII performance index (PIABS; Fig. 2b) but increased the value of heat dissipation (DI0/RC; Fig. 2c). Treatment with NPs did not affect any of these parameters under control conditions. However, leaf photosynthetic characteristics were improved in NP-treated plants. The effect was specific to NPs, as mock controls using pre-treatment with equivalent concentration of citrate did not ameliorate the inhibitory effect of the salt treatment on PSII operation.
Effect of salt treatments on the fresh weight of the third leaf (a) and total shoot fresh weight (b) in plants treated with two concentrations of Fe3O4 NPs (200 mg L−1, abbreviated as +NPs(200); and 500 mg L−1, abbreviated as +NPs(500)). Data is means ± s.d. (n = 12). Data labelled with different letters is significantly different at P < 0.05.
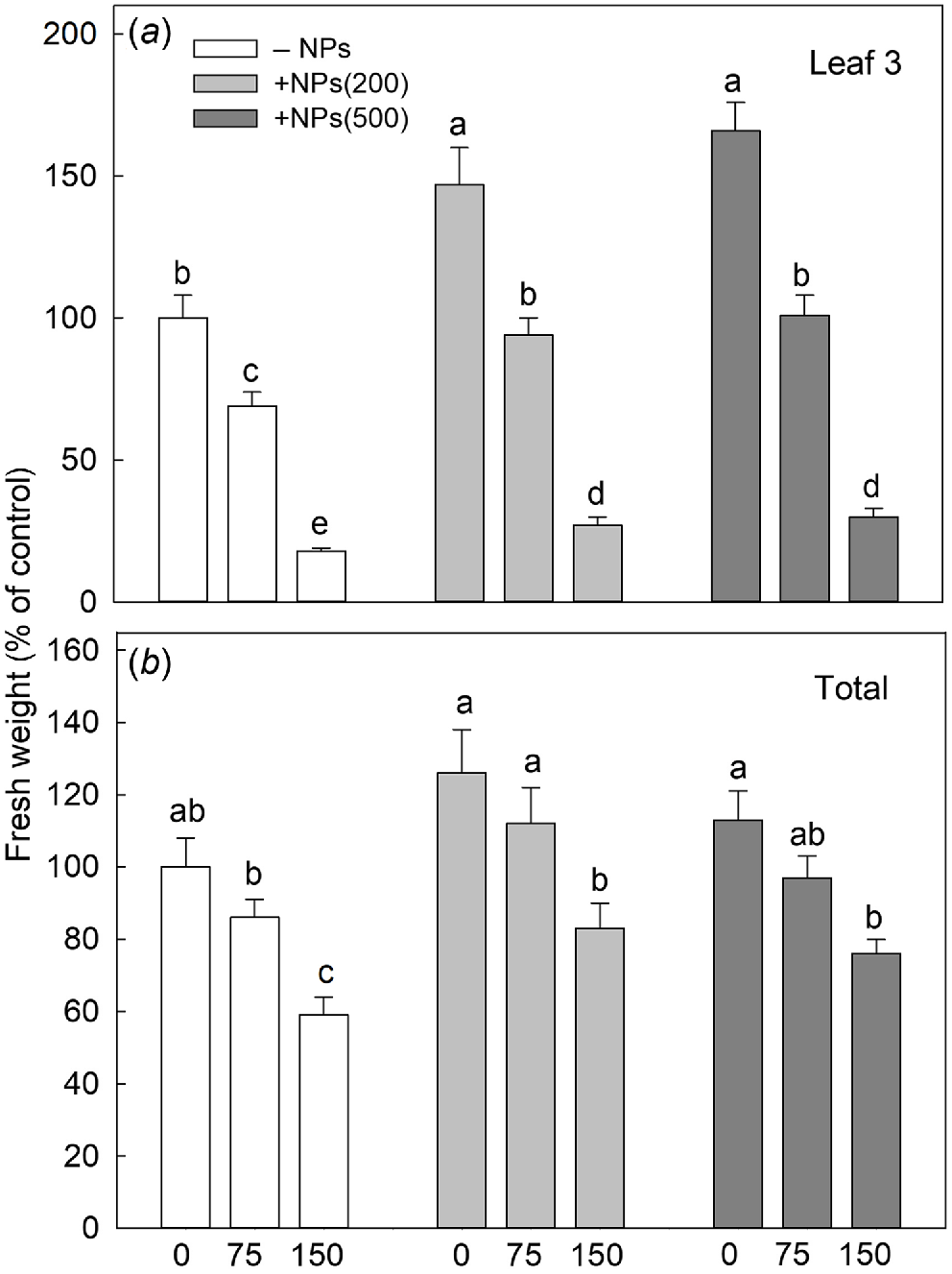
Effect of salt treatment on chlorophyll fluorescence characteristics in plants treated with 500 mg L−1 Fe3O4 NPs. (a) maximal quantum yield of PSII (Fv/Fm ratio); (b) performance index (PIABS); (c) PSII energy dissipation (DI0/RC). Data is means ± s.d. (n = 12). Data labelled with different letters is significantly different at P < 0.05.
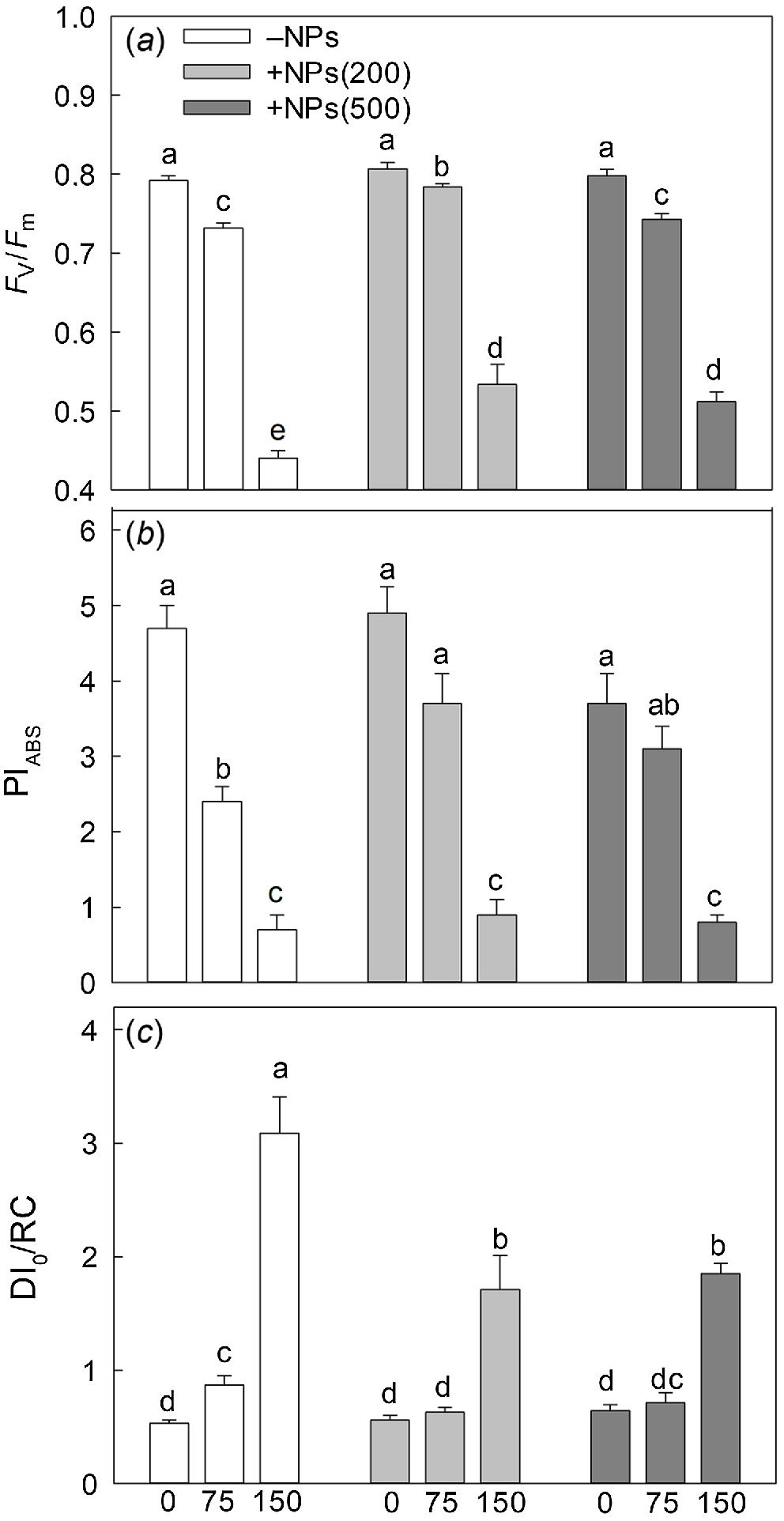
Photosynthetic rates were higher in plants treated with NP (Table 1, Fig. 3). Salinity stress reduced the rate of net CO2 assimilation in a dose-dependent manner (Fig. 3) but this reduction was ameliorated in NP-treated plants. However, the positive effect of iron citrate pre-treatment was absent suggesting the effect was specific to NPs. The similar conclusion was made for effect of NPs on leaf respiration (Table 1).
NaCl concentration (mM) | NP content (mg L−1) | Pn (% of control) | R (% of control) | |
---|---|---|---|---|
0 | 0 | 100 ± 4b | 100 ± 7ab | |
75 | 0 | 91 ± 5b | 92 ± 6b | |
0 | 200 | 132 ± 6a | 126 ± 8a | |
75 | 200 | 161 ± 10a | 85 ± 5b | |
0 | 200 IC | 105 ± 5b | 109 ± 8ab | |
75 | 200 IC | 68 ± 4c | 58 ± 5c |
Data is means ± s.d. (n = 6). Data labelled with different letters is significantly different at P < 0.05. Plants treated with iron citrate (IC) were used as mock controls for NP treatments.
Effect of salt treatment on net CO2 assimilation in plants treated with 500 mg L−1 Fe3O4 NPs. Data is means ± s.d. (n = 6). Data labelled with different letters is significantly different at P < 0.05.
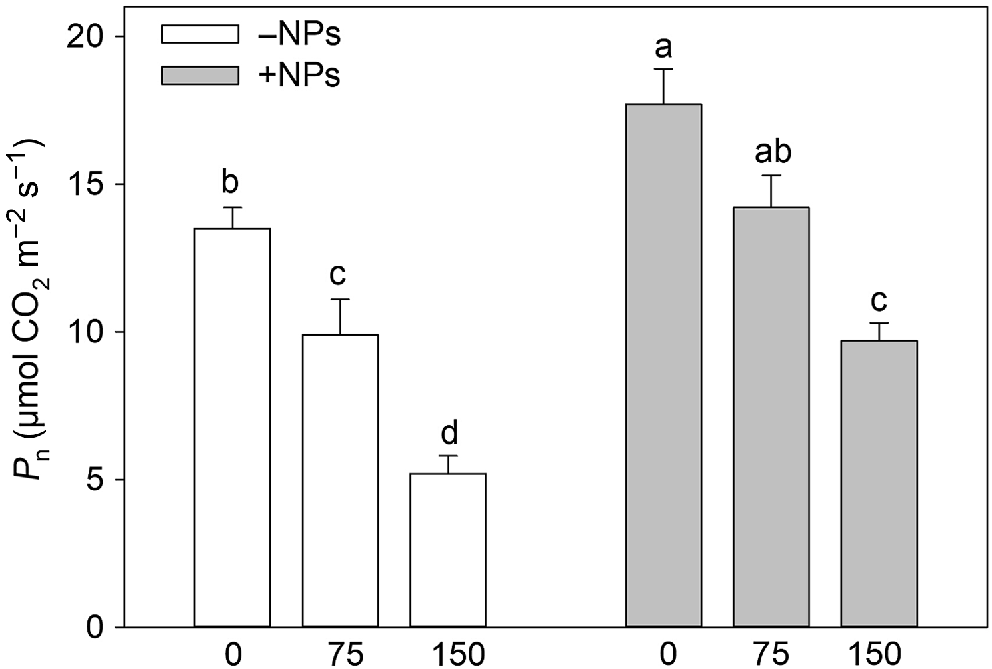
No noticeable increase in pigment content was observed in NPs-treated plants under control conditions (Fig. 4a, b). However, Fe3O4 NP treatment relieved the negative effect of salinity on photosynthetic pigments content leading to increased chl (a + b) content reduced by salinity.
Redox characteristics, proline (Pro) content and elemental composition
Activity of APX was not affected by NP treatment under control conditions but increased in salt (150 mM) grown plants (Fig. 5a). Activity of POD was increased in NP-treated and salt-grown plants (Fig. 5b). The MDA content was increased upon salinity exposure (Fig. 5c). NPs treatment has markedly decreased MDA content in leave in salt-grown plants. Pro content data showed no obvious trends (Fig. 6), with most changes being physiologically not significant.
Effect of salt treatment on antioxidant activity and redox relations in plants treated with 500 mg L−1 Fe3O4 NPs. (a) APX activity; (b) POD activity; (c) MDA content. Data is means ± s.d. (n = 6). Data labelled with different letters is significantly different at P < 0.05.
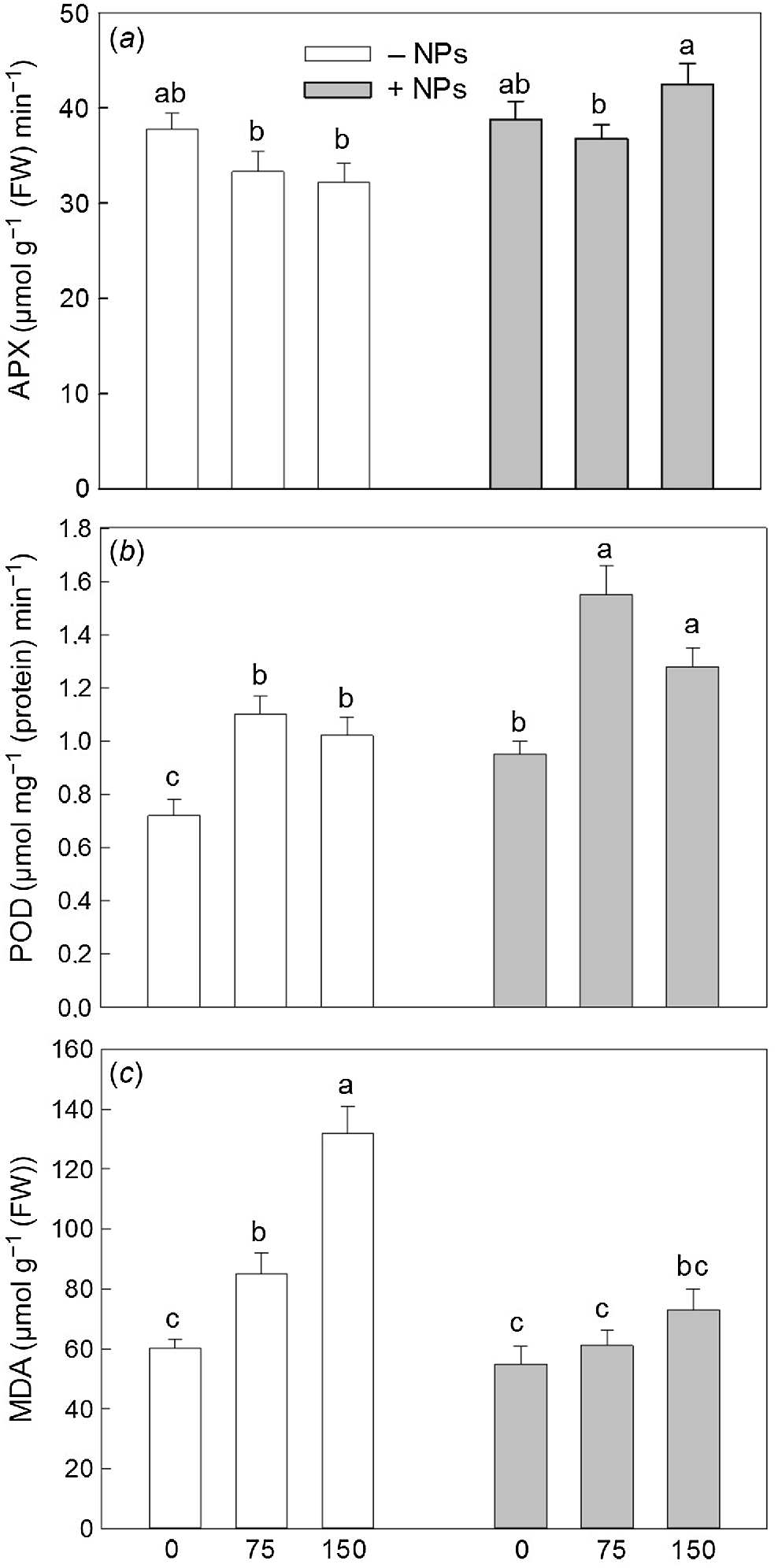
Effect of salt treatment on proline content in plants treated with 500 mg L−1 Fe3O4 NPs. Data is means ± s.d. (n = 6). Data labelled with different letters is significantly different at P < 0.05.
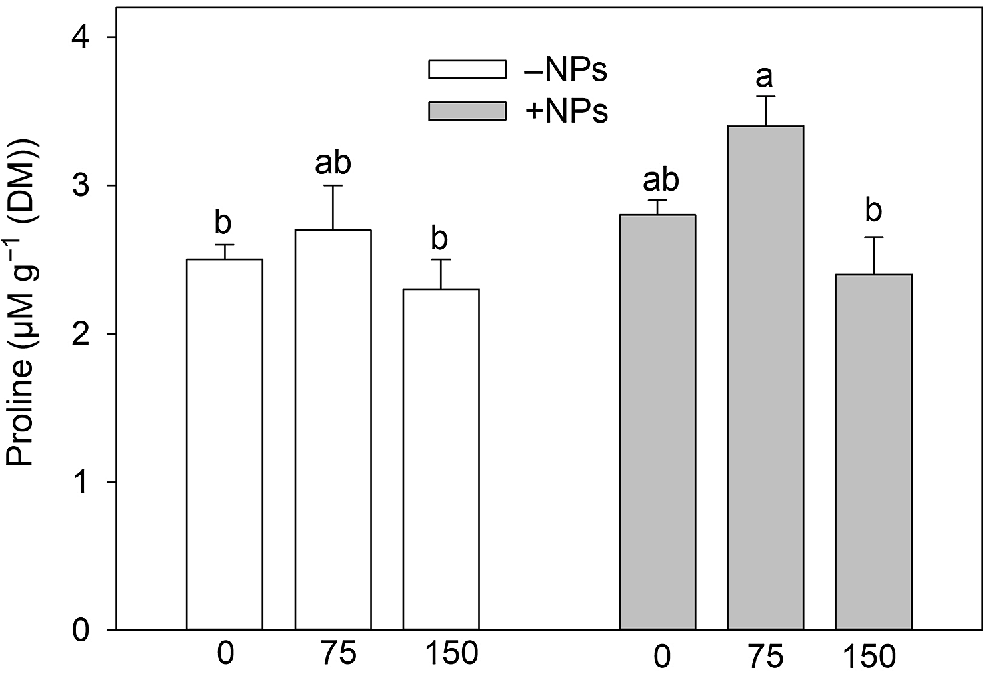
Sodium and chloride content has significantly increased in plant leaves under saline conditions (Fig. 7a, b), and treatment with NPs had only very minor effect (a small reduction in Cl content; no impact on Na content). However, both Fe and Mn content was drastically increased in NP-treated plants exposed to salinity while no difference was reported for control plants.
Effect of salt treatment on leaf elemental composition in plants treated with 500 mg L−1 Fe3O4 NPs. (a) Sodium, (b) chloride, (c) iron and (d) manganese. Data is means ± s.d. (n = 6). Data labelled with different letters is significantly different at P < 0.05.
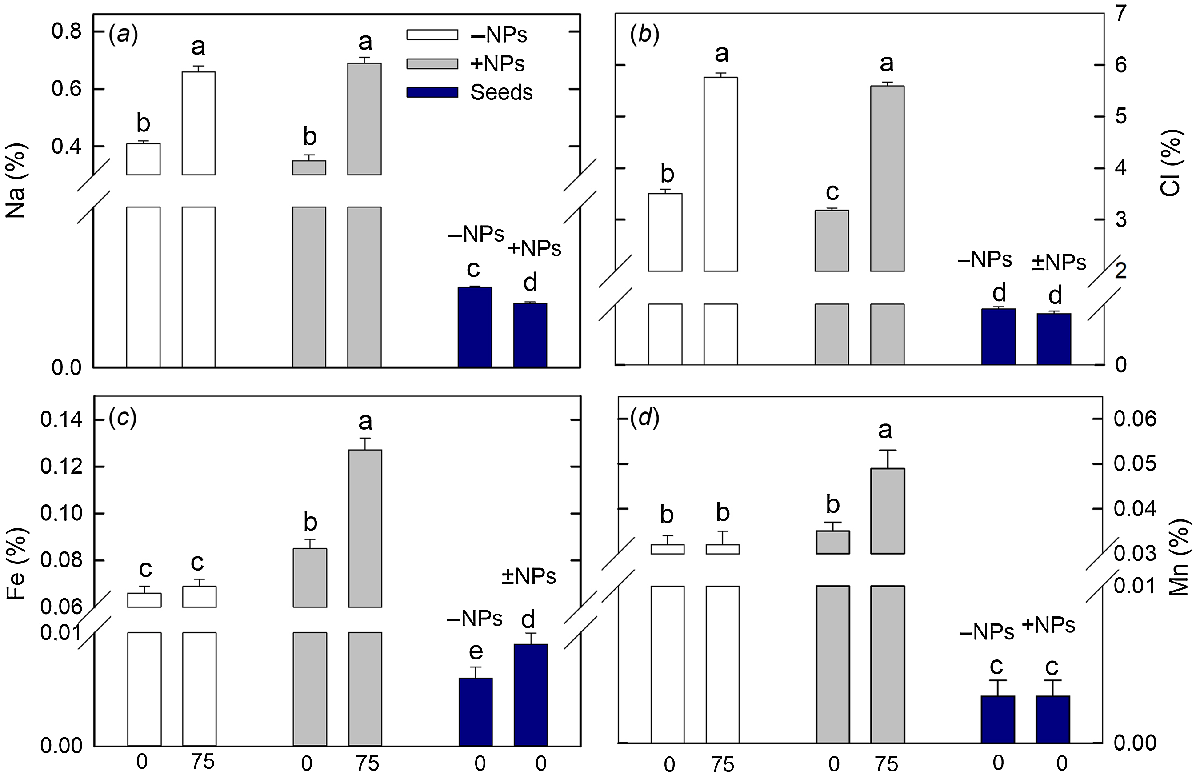
The content of mineral nutrients in the seeds was lower than in shoots. Plant treatment with NPs has significantly reduced seed Na and increased seed Fe content (Fig. 7a, c).
Discussion
Treatment of wheat seeds with Fe3O4 NPs is known to positively affect the growth and photosynthesis of wheat plants (Rizwan et al. 2019; Feng et al. 2022). In particular, treating plants with 200 mg L−1 and 500 mg L−1 Fe3O4 NPs was beneficial for photosynthetic pigments content, photosynthetic rate, and leaf carbon consumption (Feng et al. 2022). We then hypothesised that these treatments may ameliorate the inhibitory effect of NaCl salinity on plant growth and photosynthesis. Indeed, this was the case (Figs 1 and 3, Table 1). Similar data were reported earlier for ameliorative effects of Fe3O4 NPs on plant responses to cadmium stress (Rizwan et al. 2019; Manzoor et al. 2021).
Salinity stress disturbs plant ionic homeostasis and results in reduced K+/Na+ ratio in the cytosol as well as increased production of ROS in various cellular compartments (Shabala 2009). In our case, NPs treatment had no physiologically relevant effects on plant Na content, however reducing the extent of oxidative stress (as judged by MDA data; Fig. 5). Apparently, NPs treatment does not reduce Na influx but affects the pro-/antioxidant balance in leaves. This is consistent with our data on an increase in guajacol-dependent peroxidase and ascorbate peroxidase activity and a decrease in MDA content at joint treatment with NPs and NaCl compared with the treatment with NaCl alone. Apparently, treatment of seeds with NPs leads to accumulation of the Fe3O4 in the seeds, which is consistent with the greater accumulation of iron in the treated seeds (Fig. 7). NPs penetrate in the wheat upper organs and likely induce weak oxidative stress and as a result increase in antioxidant potential in leaves. This possibility is considered in the literature (Wang et al. 2016; Gupta et al. 2018).
The lack of impact of NP on shoot Na+ content in salt-grown plants was surprising, given previous reports in the literature (see review by Li et al. 2022). The possible explanation is that salinity tolerance in plants is determined not by an absolute value of amount of Na+ accumulated in the tissue but its content in the cytosol. For obvious reasons, whole-leave ion analysis does not allow to differentiate between Na+ distribution in vacuolar and cytosolic compartments. Additional experiments using fluorescence Na+ dyes (such as CoroNa Green; Wu et al. 2018) are required. Also, plant tissue tolerance is conferred by the cytosolic K/Na ratio, not just Na+ content. It can be envisaged that NP treatment might affected K+ uptake or retention in leaf mesophyll, thus improving plant tissue tolerance without affecting Na+ transport.
There are a number of studies that analyse the possible pathways of action of various NPs under stress factors associated with induction of antioxidant system and their link with intake of micro and macro elements from soil (Liu et al. 2017; Mohamed et al. 2017; Rizwan et al. 2019; Venzhik et al. 2021; Feng et al. 2022). Specifically, it has been suggested that the mechanisms of salt tolerance induction during NP treatment depend on Pro metabolism, ion accumulation, and defence mechanisms associated with increased antioxidant activity (Wahid et al. 2020). One of the ways in which low molecular weight antioxidants such as Pro act under stress is their ability to bind metal ions with variable valence and thus inhibit non-enzymatic free radical processes (Liang et al. 2013). However, in our case, Pro changes were physiologically insignificant to explain the observed phenotype (Fig. 6). On another hand, under salt stress activity of antioxidant enzymes such as POD and APX in NP-treated plants increased (Fig. 5).
In our case, the reduction in the inhibitory effect of NaCl treatment on photosynthesis due to NPs application seems to be associated with the enhancement of antioxidant activity in plant leaves. This is manifested by an increase in both total peroxidase and APX activities (Fig. 4), which are critical antioxidant enzymes (Bela et al. 2015). Fe is known to be a key determinant of the biological functions of a large number of cellular enzymes in organelles and its presence is important for maintaining photosynthesis, respiration, and growth processes (Briat et al. 2015). Manganese is also important for photosynthetic processes. An enhancement of primary photochemical processes was also observed in plants grown from NP-treated seeds and then exposed to salt treatment compared to plants exposed to the salt treatment alone (Fig. 2). It follows that the maintenance of photosynthesis under the combined action of salt stress and seed pre-treatment seems to be partly due to the enhancement of photochemical processes in plants due to the positive effect of Fe3O4 NPs pre-treatment. However, iron in ionic form (iron citrate) did not activate photosynthetic processes (Table 1).
Taken together, the negative effect of salinity on growth and photosynthesis is likely not a consequence of a marked decrease in sodium ions in leaves or an increase in potassium ions (data not shown), but rather a consequence of a decrease in oxidative stress characterised by MDA level (Fig. 5). The reason for the decrease appears to be the increased activity of antioxidant enzymes such as different peroxidases due to increased availability of Fe and Mn. The explicit proof for this hypothesis may come from experiments using mutants with altered antioxidant activity or by using gene editing tools such as CRISP/R.
The dual role of NPs is known; on the one hand, they act as inducers of oxidative stress, and on the other hand, they can counteract the development of stress and increase the potential of the plant antioxidant system (Rui et al. 2016; Askary et al. 2017; Amjad et al. 2018; Singh et al. 2021). Seed treatment with nanomaterials appears to provide a sustainable and inexpensive tool for enhancing plant stress tolerance at the initial stress vulnerable stage (Wahid et al. 2020). Thus, treatment of wheat seeds with Fe3O4 NPs resulted in decrease in the negative effects of salinity on plant growth and photosynthesis. Less inhibition of the growth in plants grown from seeds treated with the NP appear to be due to a higher rate of photosynthesis and increased respiration as well as a higher antioxidant potential in the leaves of these plants. Thus, the use of NPs can contribute to the sustainability goal of increasing crop yields by using a sufficiently safe and environmentally friendly nanobiotechnology.
Conflicts of interest
Sergey Shabala and Suleyman Allakhverdiev are both Editors of Functional Plant Biology. To mitigate this potential conflict of interest they were blinded from the review process. The authors declare no other conflicts of interest.
Declaration of funding
The reported study was funded by joint grant RFBR (No. 21-54-53015) and NSFC (No. 3201101730, No. 21-54-53015), the Science and Technology Department of Guangdong Province (Grant No. 2018A050506085, 163-2018-XMZC-0001-05-0049), the Higher Education Department of Guangdong Province (Grant No. 2020KCXTD025). The results (Fig. 3) were obtained within the state assignment of Ministry of Science and Higher Education of the Russian Federation (theme No. 122050400128-1).
References
Abdel Latef AAH, Alhmad MFA, Abdelfattah KE (2017) The possible roles of priming with ZnO nanoparticles in mitigation of salinity stress in lupine (Lupinus termis) plants. Journal of Plant Growth Regulation 36, 60-70.
| Crossref | Google Scholar |
Abhilash M (2010) Potential applications of nanoparticles. International Journal of Pharmacy and Biological Sciences 1, 1-12.
| Google Scholar |
Ahmad I, Akhtar MS (2019) Use of nanoparticles in alleviating salt stress. In ‘Salt stress, microbes, and plant interactions: causes and solution’. (Ed. MS Akhtar) pp. 199–215. (Springer: Singapore) doi:10.1007/978-981-13-8801-9
Amjad S, Sharma AK, Serajuddin M (2018) Toxicity assessment of cypermethrin nanoparticles in Channa punctatus: behavioural response, micronuclei induction and enzyme alteration. Regulatory Toxicology and Pharmacology 100, 127-133.
| Crossref | Google Scholar |
Askary M, Talebi SM, Amini F, Bangan ADB (2017) Effects of iron nanoparticles on Mentha piperita L. under salinity stress. Biologija 63, 65-75.
| Crossref | Google Scholar |
Bakhtiari M, Moaveni P, Sani B (2015) The effect of magnetite nanoparticles spraying time and concentration on wheat. Biological Forum – An International Journal 7, 679-683.
| Google Scholar |
Baruah S, Dutta J (2009) Nanotechnology applications in pollution sensing and degradation in agriculture: a review. Environmental Chemistry Letters 7, 191-204.
| Crossref | Google Scholar |
Bates LS, Waldren RP, Teare ID (1973) Rapid determination of free proline for water-stress studies. Plant and Soil 39, 205-207.
| Crossref | Google Scholar |
Bela K, Horváth E, Gallé Á, Szabados L, Tari I, Csiszár J (2015) Plant glutathione peroxidases: emerging role of the antioxidant enzymes in plant development and stress responses. Journal of Plant Physiology 176, 192-201.
| Crossref | Google Scholar |
Bradford MM (1976) A rapid and sensitive method for the quantitation of microgram quantities of protein utilizing the principle of protein-dye binding. Analytical Biochemistry 72, 248-254.
| Crossref | Google Scholar |
Briat J-F, Dubos C, Gaymard F (2015) Iron nutrition, biomass production, and plant product quality. Trends in Plant Science 20, 33-40.
| Crossref | Google Scholar |
Chance B, Maehly AC (1955) [136] Assay of catalases and peroxidases. Methods in Enzymology 2, 764-775.
| Crossref | Google Scholar |
Cuin TA, Tian Y, Betts SA, Chalmandrier R, Shabala S (2009) Ionic relations and osmotic adjustment in durum and bread wheat under saline conditions. Functional Plant Biology 36, 1110-1119.
| Crossref | Google Scholar |
Demidchik V (2015) Mechanisms of oxidative stress in plants: from classical chemistry to cell biology. Environmental and Experimental Botany 109, 212-228.
| Crossref | Google Scholar |
Elfeky SA, Mohammed MA, Khater MS, Osman YAH, Elsherbini AAM (2013) Effect of magnetite nano-fertilizer on growth and yield of Ocimum basilicum L. International Journal of Indigenous Medicinal Plants 46, 1286-1293.
| Google Scholar |
Feng Y, Kreslavski VD, Shmarev AN, Ivanov AA, Zharmukhamedov SK, Kosobryukhov A, Yu M, Allakhverdiev SI, Shabala S (2022) Effects of iron oxide nanoparticles (Fe3O4) on growth, photosynthesis, antioxidant activity and distribution of mineral elements in wheat (Triticum aestivum). Plants 11, 1894.
| Crossref | Google Scholar |
Ghafari H, Razmjoo J (2013) Effect of foliar application of nano-iron oxidase, iron chelate and iron sulphate rates on yield and quality of wheat. International Journal of Agronomy and Plant Production 4, 2997-3003.
| Google Scholar |
Gupta SD, Agarwal A, Pradhan S (2018) Phytostimulatory effect of silver nanoparticles (AgNPs) on rice seedling growth: an insight from antioxidative enzyme activities and gene expression patterns. Ecotoxicology and Environmental Safety 161, 624-633.
| Crossref | Google Scholar |
Hojjat SS (2019) Effect of interaction between Ag nanoparticles and salinity on germination stages of Lathyrus Sativus L. Open Access Journal of Environmental and Soil Sciences 2, 193-198.
| Google Scholar |
Iannone MF, Groppa MD, de Sousa ME, Fernández van Raap MB, Benavides MP (2016) Impact of magnetite iron oxide nanoparticles on wheat (Triticum aestivum L.) development: evaluation of oxidative damage. Environmental and Experimental Botany 131, 77-88.
| Crossref | Google Scholar |
Kreslavski VD, Lankin AV, Vasilyeva GK, Luybimov VY, Semenova GN, Schmitt F-J, Friedrich T, Allakhverdiev SI (2014) Effects of polyaromatic hydrocarbons on photosystem II activity in pea leaves. Plant Physiology and Biochemistry 81, 135-142.
| Crossref | Google Scholar |
Li Z, Zhu L, Zhao F, Li J, Zhang X, Kong X, Wu H, Zhang Z (2022) Plant salinity stress response and nano-enabled plant salt tolerance. Frontiers in Plant Science 13, 843994.
| Crossref | Google Scholar |
Liang X, Zhang L, Natarajan SK, Becker DF (2013) Proline mechanisms of stress survival. Antioxidants & Redox Signaling 19, 998-1011.
| Crossref | Google Scholar |
Lichtenthaler HK (1987) [34] Chlorophylls and carotenoids: pigments of photosynthetic biomembranes. Methods in Enzymology 148, 350-382.
| Crossref | Google Scholar |
Liu H, Zhang C, Wang J, Zhou C, Feng H, Mahajan MD, Han X (2017) Influence and interaction of iron and cadmium on photosynthesis and antioxidative enzymes in two rice cultivars. Chemosphere 171, 240-247.
| Crossref | Google Scholar |
Maas EV, Hoffman GJ (1977) Crop salt tolerance-current assessment. Journal of the Irrigation and Drainage Division 103, 115-134.
| Crossref | Google Scholar |
Manzoor N, Ahmed T, Noman M, Shahid M, Nazir MM, Ali L, Alnusaire TS, Li B, Schulin R, Wang G (2021) Iron oxide nanoparticles ameliorated the cadmium and salinity stresses in wheat plants, facilitating photosynthetic pigments and restricting cadmium uptake. Science of The Total Environment 769, 145221.
| Crossref | Google Scholar |
Mazaherinia S, Astaraei AR, Fotovat A, Monshi A (2010) Nano iron oxide particles efficiency on Fe, Mn, Zn and Cu concentrations in wheat plant. World Applied Sciences Journal 7, 36-40.
| Google Scholar |
Mohamed AKSH, Qayyum MF, Abdel-Hadi AM, Rehman RA, Ali S, Rizwan M (2017) Interactive effect of salinity and silver nanoparticles on photosynthetic and biochemical parameters of wheat. Archives of Agronomy and Soil Science 63, 1736-1747.
| Crossref | Google Scholar |
Ozgur R, Uzilday B, Sekmen AH, Turkan I (2013) Reactive oxygen species regulation and antioxidant defence in halophytes. Functional Plant Biology 40, 832-847.
| Crossref | Google Scholar |
Pan T, Liu M, Kreslavski VD, Zharmukhamedov SK, Nie C, Yu M, Kuznetsov VV, Allakhverdiev SI, Shabala S (2021) Non-stomatal limitation of photosynthesis by soil salinity. Critical Reviews in Environmental Science and Technology 51, 791-825.
| Crossref | Google Scholar |
Qamer Z, Chaudhary MT, Du X, Hinze L, Azhar MT (2021) Review of oxidative stress and antioxidative defense mechanisms in Gossypium hirsutum L. in response to extreme abiotic conditions. Journal of Cotton Research 4, 9.
| Crossref | Google Scholar |
Rizwan M, Ali S, Ali B, Adrees M, Arshad M, Hussain A, Zia ur Rehman M, Waris AA (2019) Zinc and iron oxide nanoparticles improved the plant growth and reduced the oxidative stress and cadmium concentration in wheat. Chemosphere 214, 269-277.
| Crossref | Google Scholar |
Rui M, Ma C, Hao Y, Guo J, Rui Y, Tang X, Zhao Q, Fan X, Zhang Z, Hou T, Zhu S (2016) Iron oxide nanoparticles as a potential iron fertilizer for peanut (Arachis hypogaea). Frontiers in Plant Science 7, 815.
| Crossref | Google Scholar |
Shabala S (2009) Salinity and programmed cell death: unravelling mechanisms for ion specific signalling. Journal of Experimental Botany 60, 709-712.
| Crossref | Google Scholar |
Shabala S, Bose J, Fuglsang AT, Pottosin I (2016) On a quest for stress tolerance genes: membrane transporters in sensing and adapting to hostile soils. Journal of Experimental Botany 67, 1015-1031.
| Crossref | Google Scholar |
Siddiqi KS, ur Rahman A, Tajuddin S, Husen A (2016) Biogenic fabrication of iron/iron oxide nanoparticles and their application. Nanoscale Research Letters 11, 498.
| Crossref | Google Scholar |
Singh A, Tiwari S, Pandey J, Lata C, Singh IK (2021) Role of nanoparticles in crop improvement and abiotic stress management. Journal of Biotechnology 337, 57-70.
| Crossref | Google Scholar |
Torney F, Trewyn BG, Lin VS-Y, Wang K (2007) Mesoporous silica nanoparticles deliver DNA and chemicals into plants. Nature Nanotechnology 2, 295-300.
| Crossref | Google Scholar |
Towett EK, Shepherd KD, Cadisch G (2013) Quantification of total element concentrations in soils using total X-ray fluorescence spectroscopy (TXRF). Science of The Total Environment 463–464, 374-388.
| Crossref | Google Scholar |
Uchiyama M, Mihara M (1978) Determination of malonaldehyde precursor in tissues by thiobarbituric acid test. Analytical Biochemistry 86, 271-278.
| Crossref | Google Scholar |
Usman M, Farooq M, Wakeel A, Nawaz A, Cheema SA, Rehman Hu, Ashraf I, Sanaullah M (2020) Nanotechnology in agriculture: current status, challenges and future opportunities. Science of The Total Environment 721, 137778.
| Crossref | Google Scholar |
van Zelm E, Zhang Y, Testerink C (2020) Salt tolerance mechanisms of plants. Annual Review of Plant Biology 71, 403-433.
| Crossref | Google Scholar |
Veljovic-Jovanovic S, Kukavica B, Stevanovic B, Navari-Izzo F (2006) Senescence- and drought-related changes in peroxidase and superoxide dismutase isoforms in leaves of Ramonda serbica. Journal of Experimental Botany 57, 1759-1768.
| Crossref | Google Scholar |
Venzhik YV, Moshkov IE, Dykman LA (2021) Influence of nanoparticles of metals and their oxides on the photosynthetic apparatus of plants. Biology Bulletin 48, 140-155.
| Crossref | Google Scholar |
Wahid I, Kumari S, Ahmad R, Hussain SJ, Alamri S, Siddiqui MH, Khan MIR (2020) Silver nanoparticle regulates salt tolerance in wheat through changes in ABA concentration, ion homeostasis, and defense systems. Biomolecules 10, 1506.
| Crossref | Google Scholar |
Wang H, Kou X, Pei Z, Xiao JQ, Shan X, Xing B (2011) Physiological effects of magnetite (Fe3O4) nanoparticles on perennial ryegrass (Lolium perenne L.) and pumpkin (Cucurbita mixta) plants. Nanotoxicology 5, 30-42.
| Crossref | Google Scholar |
Wang X, Yang X, Chen S, Li Q, Wang W, Hou C, Gao X, Wang L, Wang S (2016) Zinc oxide nanoparticles affect biomass accumulation and photosynthesis in Arabidopsis. Frontiers in Plant Science 6, 1243.
| Crossref | Google Scholar |
Wu H, Shabala L, Azzarello E, Huang Y, Pandolfi C, Su N, Wu Q, Cai S, Bazihizina N, Wang L, Zhou M, Mancuso S, Chen Z, Shabala S (2018) Na+ extrusion from the cytosol and tissue-specific Na+ sequestration in roots confer differential salt stress tolerance between durum and bread wheat. Journal of Experimental Botany 69, 3987-4001.
| Crossref | Google Scholar |
Zhao C, Zhang H, Song C, Zhu J-K, Shabala S (2020) Mechanisms of plant responses and adaptation to soil salinity. The Innovation 1(1), 100017.
| Crossref | Google Scholar |