Genome editing for improvement of biotic and abiotic stress tolerance in cereals
Safeena Inam A # , Amna Muhammad A # , Samra Irum A , Nazia Rehman A , Aamir Riaz

A
Handling Editor: Sajid Fiaz
Abstract
Global agricultural production must quadruple by 2050 to fulfil the needs of a growing global population, but climate change exacerbates the difficulty. Cereals are a very important source of food for the world population. Improved cultivars are needed, with better resistance to abiotic stresses like drought, salt, and increasing temperatures, and resilience to biotic stressors like bacterial and fungal infections, and pest infestation. A popular, versatile, and helpful method for functional genomics and crop improvement is genome editing. Rapidly developing genome editing techniques including clustered regularly interspaced short palindromic repeats (CRISPR) and CRISPR-associated protein (Cas) are very important. This review focuses on how CRISPR/Cas9 genome editing might enhance cereals’ agronomic qualities in the face of climate change, providing important insights for future applications. Genome editing efforts should focus on improving characteristics that confer tolerance to conditions exacerbated by climate change (e.g. drought, salt, rising temperatures). Improved water usage efficiency, salt tolerance, and heat stress resilience are all desirable characteristics. Cultivars that are more resilient to insect infestations and a wide range of biotic stressors, such as bacterial and fungal diseases, should be created. Genome editing can precisely target genes linked to disease resistance pathways to strengthen cereals’ natural defensive systems.
Keywords: agricultural innovation, biotic and abiotic stress tolerance, cereals, climate change, CRISPR variants, disease resistance, genome editing, plant biotechnology.
Introduction
Through Agrobacterium-mediated transfer DNA (T-DNA) insertion, genetically modified plants have benefited farmers and consumers in terms of the environment, agriculture, society, and economics; although concerns have been raised about their safety for the environment and human consumption (Craig et al. 2008). Finding mutations, recognising T-DNA, and identifying insertions takes time. As a result, Voytas (2013) introduced programmable sequence-specific nucleases (SSNs) enabling precise genome editing, and revolutionising genome engineering (Voytas 2013). Over the last several decades, scientists have discovered many important SSNs that can be readily changed and reprogrammed to generate double-stranded breaks (DSBs) at the correct chromosomal location. ZFNs (zinc-finger nucleases), TALENs (transcription activator-like effector nucleases), and the CRISPR/Cas system (clustered regularly interspaced short palindromic repeats and CRISPR-associated proteins) are three major genome engineering tools that have been exploited for a wide range of applications (Bhardwaj and Nain 2021). Though emerging agricultural products face societal and regulatory obstacles (Schaeffer and Nakata 2015; Gao et al. 2018), contemporary plant breeding methods such as genome editing provide crop health improvement without the introduction of a transgene (Ahmad 2023). Recently, several innovative breeding techniques have made it easier to generate high-yielding, disease-free agricultural varieties. These approaches include precise genome editing, high throughput genotyping, and similarly speedy breeding platforms when paired with genetic engineering (Wei et al. 2020; Nerkar et al. 2022). Site-specific nucleases, ZFNs, TALENs, and CRISPR/Cas9 are now all commonly accessible genome editing methods (Li et al. 2020a; Wei et al. 2020). ZFNs were the first protein reagent to target a particular protein. Single guide RNA (sgRNA)-controlled genomic targets are cut by the CRISPR/Cas9 nuclease. The error-prone nonhomologous end-joining repair mechanism repairs the resulting DSBs, potentially leading to insertion–deletion (indel) mutations (Zhu et al. 2017). Therefore, the CRISPR/Cas9 system may promote agricultural progress by potentially enabling escape from GM control, since mutants produced by the method do not essentially include any alien DNA insertions (Zhu et al. 2017; Gao et al. 2018; Zhang et al. 2020; Yao et al. 2023). Furthermore, the use of CRISPR/Cas9 has resulted in substantial advances in cereals and other crops. These findings indicate that this technique might be extensively used in rice (Fig. 1) breeding and innovation, encouraging directional diversity and the development of breeding methods. This review focuses on abiotic and abiotic stress tolerance development in wheat. Furthermore, we addressed potential molecular techniques for improving cereals.
Enhancing crop resilience via molecular tools
Improving plants’ resistance to abiotic stresses like heat, cold, salinity, and drought, and biotic stresses like insects, pests, and diseases, is essential to meeting the global food security challenge in the context of climate change. Global agricultural systems are seriously threatened by the adverse effects of climate change, such as increasing temperatures, different rainfall patterns, and a surge in the occurrence of extreme weather events. Advanced strategies for genetic manipulation present a possible method for producing crops that are more resilient to climate change (KhokharVoytas et al. 2023).
Crop improvement is a multifaceted process that combines several agronomic techniques for crop production in addition to selection or breeding for a desired characteristic. Most crop enhancement procedures involve changing, deleting, or adding one or more genes that are responsible for the desired feature in crop plants. Researchers now have a major advantage in manipulating the expression of a plant gene or genes due to advancements in genetic engineering techniques.
Genome modifications
A class of technologies known as genome editing, or gene editing, enables researchers to modify an organism’s DNA. This involves introducing, eliminating, or changing genetic material at certain genomic regions. CRISPR/Cas9 is a popular genome editing method that guides the Cas9 enzyme to cut DNA at a specific location using a guide RNA. The genetic material is added, removed, or altered by the cell using its DNA repair machinery. Recently, the development of customised endonucleases has started to open the door for the insertion of mutations or predetermined sequence alterations at any genomic location, followed by transgene elimination through progeny segregation (Koeppel et al. 2019). This novel technique called ‘genome editing’ has already been used and proven effective in the majority of crop species. Endonucleases have the potential for mutational breeding in crops. This can be achieved by precisely introducing known allelic states that confer required characteristics into progressive breeding backgrounds or through creating new genetic diversity in the target genes (Hisano et al. 2021).
Several methods (Fig. 2, Table 1), including oligonucleotide-directed mutagenesis, homologous recombination, site-specific gene integration using recombinase, and site-specific genome modification using nucleases, are used to change the plant genome in a target-specific manner (Cardi and Neal Stewart 2016). Mutation in the plant genome can result in knockout, knockdown, gene upregulation or downregulation, or it might not affect the expression at all. The current focus of genome engineering is on precisely altering the plant genome through the nuclease activity (Voytas 2013).
Molecular tools such as meganucleases (a), zinc finger nuclease (b), TALENs (c), CRISPR/Cas9 (d), base editing (e), and prime editing (f) are used for the improvement of crop resilience. Restriction endonuclease Fok1, naturally found in Flavobacterium okeanokoites TALENs, or transcription activator-like (TAL). The red circle labelled ‘C’ in (e) indicates the cytosine base editing. gRNA, guide RNA; pegRNA, prime-editing guide RNA.
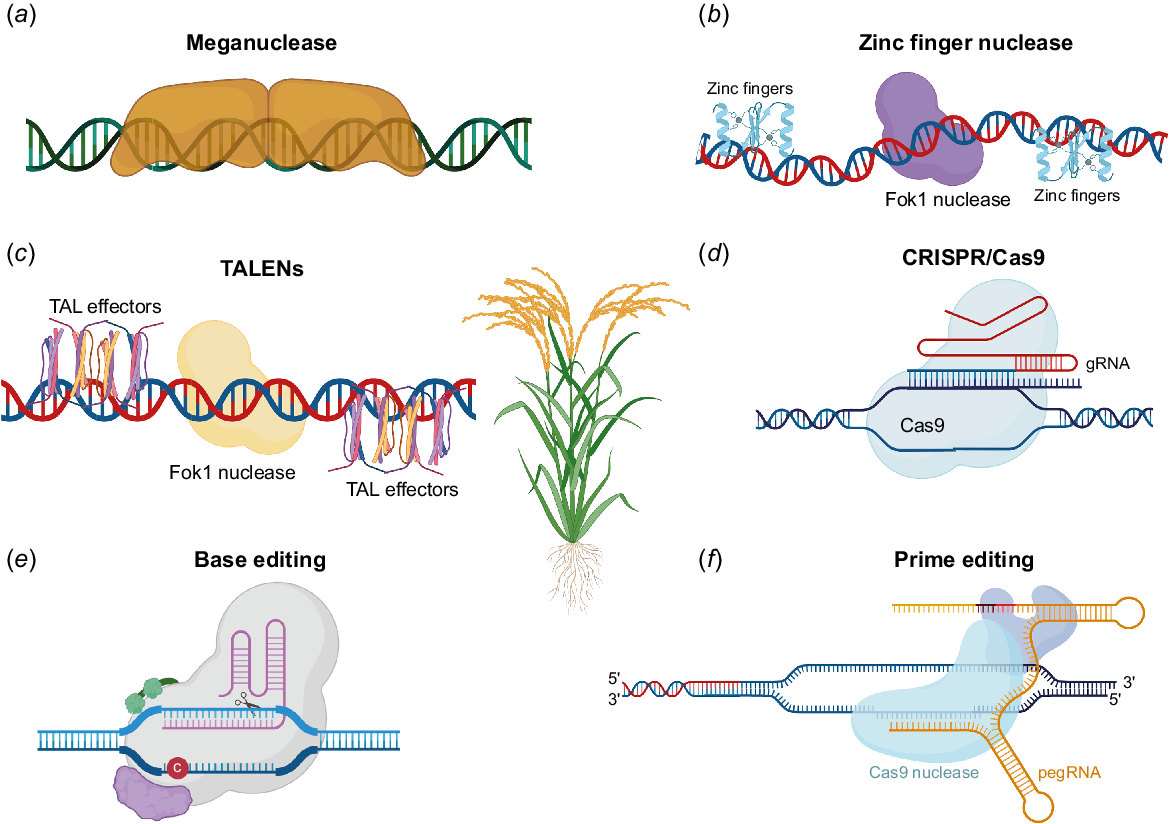
Parameters | Meganucleases | ZFNs | TALENs | CRISPR/Cas | Base editing | Prime editing | References | |
---|---|---|---|---|---|---|---|---|
Source | Bacteria/plants/phages/animals | Eukaryotes | Bacteria | Bacteria/Archaea | Bacteria | Bacteria | Chandrasegaran and Carroll (2016) | |
Enzymes | Endonuclease | Fok1 | Fok1 | Cas9 and its variants | dcas9 | pegRNA | Costa et al. (2017) | |
Target recognition efficiency | Low | Low | Normal | High | Very high | Very high | Bhuyan et al. (2023) | |
Recognition site | 18–44 bp | 18–36 bp | 24–40 bp | 17–23 bp | 4–8 bp | 8–16 bp | Li et al. (2020a) | |
Mode of action | DSB | DSB | DSB | DSB | Single stand nick | Single strand nick | Janik et al. (2020) | |
Repair | HDR | NHEJ | HDR | NHEJ | BER MMR | MMR | Gaj et al. (2013), Kantor et al. (2020) | |
Efficiency | High | Medium | Medium | High | Higher | Highest | Zheng et al. (2020), Xie et al. (2022) | |
Cost | High | High | Higher | Low | Low | Low | Guha and Edgell (2017) | |
Applications | Gene editing | Gene knockout, transcriptional regulation | Gene knockout, transcriptional regulation | Gene knockout, transcriptional regulation | SNVs | All type base conversions |
BER, base excision repair pathway; HDR, homology-directed repair; MMR, specific DNA mismatch repair; NHEJ, nonhomologous end-joining; SNVs, single nucleotide variant.
Meganucleases, ZFNs, and TALENs
Meganucleases, zinc-finger nucleases (ZFNs), transcription activator-like effector nucleases (TALENs), and clustered regularly interspaced short palindromic repeats (CRISPR)/Cas endonucleases are among the several efficient endonucleases used for genome editing (Mohanta et al. 2017) (Table 1). Meganucleases, like I-CreI, I-DmoI, I-SceI and I-DmoI, have three distinct features: a site-specific double stranded (ds) DNA binding domain of 12–40 nucleotides, an endonuclease domain that breaks the DNA and a transit peptide for nuclear localisation. Meganucleases are very specific for target sequences, unlike TALENs, ZFNs, or Cas endonucleases (Gaj et al. 2013).
A transgene with a promoter, such as the UBIQUITIN or cauliflower mosaic virus 35S, which stimulates the production of these nucleases and is recognised by RNA polymerase II, is typically inserted to use these nucleases in plants. A nuclease domain generated from the restriction enzyme Fok1 is joined to a DNA-binding domain to form the chimeric proteins TALENs and ZFNs. The zinc-finger nuclease (ZFN) DNA-binding domain consists of three to six zinc-finger modules that are generated from transcription factors that bind DNA. Each zinc-finger module recognises a different nucleotide triplet. Approximately 20 modules of four basic types, each specific to one of the four nucleo-bases (A, C, G, or T), make up the DNA-binding domain of TALENs (González Castro et al. 2021). The 35S promoter was effectively inserted upstream of a promoter-less herbicide-resistant transgenic in maize through targeted genome editing with meganuclease. (D’Halluin et al. 2008). ZFNs were first used for the IPK1 gene in maize. They were also utilised to insert a PAT gene into a similar locus using a site-directed DNA insertion (Shukla et al. 2009). The bacterial leaf blight susceptibility gene OsSWEET14, a susceptible gene in rice that causes bacterial leaf blight disease, was first successfully modified by using TALENs (Li et al. 2012).
CRISPR/Cas
TALEN and ZFN have not been used extensively because of their high failure rate and difficult procedures. CRISPR/Cas is the most widely used of these methods due to its simplicity, low cost, high efficacy, low mutation rate, and faster rate of progress. There are numerous uses for CRISPR/Cas9 in humans, animals, plants, and microorganisms. Moreover, it finds use in horticulture, targeted human genome therapy, and food biotechnology, among other areas (Akram et al. 2023). The simple and adaptable method by which CRISPR/Cas identifies targets is base complementation between the target sequence and guide RNA; target site selection just needs to conform to the specifications of the protospacer adjacent motif (PAM) sequences of various systems. In contrast to previous genome editing techniques, the CRISPR/Cas-based genome editing method is easy to modify, stable, adaptable, and uncomplicated. Owing to these characteristics, CRISPR/Cas-mediated genome editing is now the most widely used genome editing technique, surpassing both ZFN and TALEN (Hille et al. 2018).
The most effective, accurate, and productive genome editing instruments available for all live cells are CRISPR and its associated protein, Cas9, which are used in a wide range of applications. The two fundamental parts of the CRISPR/Cas9 system are gRNA and Cas9 proteins. The steps involved in editing the genome with CRISPR/Cas9 are recognition, cleavage, and DNA repair. A complementary base pair in the produced sgRNA recognises the target sequence in the gene of interest. Triple base pair breaks are produced by the Cas9 nuclease three base pairs upstream from a protospacer adjacent motif. The breaks are repaired by nonhomologous end-joining or homology-directed repair pathways in cells. The CRISPR/Cas9-based genome editing method has numerous applications in agriculture (Asmamaw and Zawdie 2021). In addition to SpCas9, additional endonucleases can also be used for genome editing (Yin et al. 2017), for instance, expressing the Cas12a endonuclease in rice to cause mutations. Unlike Cas9, Cas12a utilises a native single CRISPR RNA (crRNA) for its function. Like several other Cas endonuclease types, Cas12a has a wide range of possible target sequences as it needs a T-rich PAM (5′-(T) TTV-3′) which is located upstream of the nucleotides that are bound by the gRNA. In contrast to Cas12, Cas9 cleaves the target sequence in stages (Hisano et al. 2021).
Cereal crops are considered a major food and energy source as they provide vital nutrients for the human diet. Cereal crops account for more than 90% of the world’s food production. CRISPR/Cas9 technology is the best possible solution to meet the increasing need for cereal crops. In cereal crops, the CRISPR/Cas approach can enhance tolerance to biotic and abiotic challenges (Basu et al. 2023). Extreme environmental situations like high temperatures, salinity, drought, and heavy metals have a significant effect on the growth and development of plants, perhaps leading to a 50% decrease in agricultural yield (Liu et al. 2022). Crop varieties that are more tolerant to a variety of environmental conditions are needed in these conditions (Wolter et al. 2019). Through CRISPR research, commercially important crops like wheat (Triticum aestivum), maize (Zea mays), rice (Oryza sativa), cotton (Gossypium hirsutum), potato (Solanum tuberosum), soybean (Glycine max), brassica (Brassica napus), and tomato (Solanum lycopersicum) have been genetically modified to be resistant to heat, cold, herbicides, viruses, bacteria, and fungi (Chen et al. 2019).
Base editing
The field of molecular biology has transformed agricultural enhancement research with the beginning of genome editing technologies that allow precise alterations to plant genomes. This method has drawn interest from all around the world and has been used in functional analysis studies of crop plants. Single-nucleotide polymorphisms have been shown to determine many important agronomic features; as a result, superior crop varieties could be generated by precisely converting specific single bases in plant genomes under controlled conditions. For this, a novel sort of genome editing called base editing can be applied. Without resulting in double-strand breakage, base editing directly and irreversibly modifies single bases (Bharat et al. 2020) . The accuracy, ease of usage, and multiplex possibilities of this approach have led to its recent increasing adoption.
Without the requirement for donor templates or dsDNA breaks, point mutations can be successfully inserted at the target genomic locus using base editing technology. This method offers a great deal of potential for improving gene repair and genetic variety in human, plant, animal, and yeast cells (Lu and Zhu 2017). An adenosine or cytosine deaminase domain that changes one base to another is connected to a catalytically inactive CRISPR/Cas9 domain (also known as Cas9 variations, deadCas9, or Cas9 nickase) (Yuan et al. 2023). The two primary kinds of base editors are adenosine base editors (ABEs) and cytosine base editors (CBEs). All four kinds of transition mutations can be inserted into the genome by these two base editors without causing breaks in the double strand of DNA (Gu et al. 2021). Because of their effectiveness and convenience of use, ABE and CBE base editors have been used extensively in humans and plants, for gene correction and gene functional annotation. Both have been demonstrated to be effective in plant cells through research on a variety of crops, including sugar cane (Saccharum officinarum), wheat, rice, and barley (Hordeum vulgare).
Prime editing
Prime editing (PE) is a very precise genome alteration method that does not need DNA double-strand breaks or exogenous donor DNA. Instead, it is based on the ‘search and replace’ methodology. The editing capabilities of prime editing have substantially expanded as compared to basic editing technology. Prime editing is considered an efficient approach for genetic modifications and is used in different types of plant cells, animal cells, and the model microorganism Escherichia coli, and it has demonstrated a high possibility for use in functional genomics and breeding and studies of animals and plants, disease treatment, and microbial strain modification (Huang and Liu 2023). PEs are made up of a prime-editing guide RNA (pegRNA) that is linked with an engineered reverse transcriptase (RT) conjugated with nCas9 (H840A) that both specifies and edits the genome (Zhao et al. 2023).
DNA-free editing allows for targeted genetic alteration without disrupting the genome. Innovative methods have been used in different crops for non-DNA-based delivery. These novel strategies include the use of virus-like particles, the use of bacterial secretory systems for Cas/gRNA administration, and formulations of in vitro ribonucleoprotein (Cas9/gRNA) complexes (delivered by microinjection, particle bombardment, electroporation, liposomes, etc.) (Woo et al. 2015).
The above-mentioned methods are commonly used for Cas9/gRNA delivery to accomplish DNA-free editing. Delivering ribonucleoprotein synthesised in vitro is the most often used method (complexes of Cas9-gRNA ribonucleoproteins). It depends on the innate capacity of single-molecule Cas protein members (such as Cpf1 or Cas9) to interact with gRNA on their own without the use of other components. The transformation process is then followed to create a ribonucleoprotein complex (Park and Choe 2019). This technique can be modified by generating more complicated nanostructures rather than simple ribonucleoproteins. These complexes resemble but are not the same as virus-like particles. In addition to delivering readymade protein-RNA complexes, nanoparticles facilitate the integration of mRNA, after which the plant cell assembles Cas9/gRNA and performs DNA-free editing (Miller et al. 2017).
The second strategy makes use of virus-mediated RNA template delivery for encoding. Virus-like particles (VLPs) are a novel class of delivery vehicles that have been designed for gene editing (Yip 2020). The bulk of the elements of a typical viral vector, including the envelope and capsids, are present in these particles even when the virus’s DNA is absent. The major role of capsids is to enclose the viral DNA in virions inside one host, transfer it, and then release it into an alternative host cell. Today, scientists combine the high infection efficiency of viral vectors with the ephemeral nature of mRNA, protein, and CRISPR ribonucleoproteins (RNPs) delivery by using VLPs as delivery vehicles. These delivery methods provide safe and efficient DNA-free genome editing by encasing mRNAs, proteins, or RNPs into viral capsids (Lyu et al. 2020).
The third approach for free DNA editing is the introduction of CRISPR/Cas by an agrobacterium, a well-known agent that is frequently utilised to alter plants (He and Zhao 2020). Cas9 is delivered as a protein into plant cells through the Agrobacterium tumefaciens Type IV secretory system. In plant genome editing, the most fascinating development is the transport of Cas9 through the bacterial secretory system. Transient Cas9 and gRNA production can be achieved via Agrobacterium infection, preventing T-DNA integration into the plant genome, and producing DNA-free plants. By targeting the phytoene desaturase (PDS) gene in tobacco plants, transgene-free plants have been effectively produced (Chen et al. 2019). A DNA-free editing technique has been used on numerous species. Scientists have effectively manipulated Brassicaceae, rice, lettuce (Lactuca sativa), bananas (Musa paradisiaca), peppers (Capsicum annuum), potatoes, wheat, and maize without the use of DNA (Tsanova et al. 2021).
Genome editing for mitigating the impact of biotic stress
Genome editing has emerged as a solution to address the challenges posed by plant diseases and their negative impact on crop yield (Table 2). Biotic stresses such as fungal, bacterial, and viral diseases significantly impact global agriculture, affecting 11–30% of crops (Van Esse et al. 2020). The primary objective of cereal crops’ gene editing is to ensure sustainability and enhance food productivity for the growing population (Savary et al. 2019; Wang et al. 2022). Genome editing has successfully been utilised to develop disease resistance in cereal crops against pathogens fungi, bacteria, viruses, and insects (Arora and Narula 2017; Bisht et al. 2019; Matres et al. 2021).
Crops | Stress | Target genes | Functions | References | |
---|---|---|---|---|---|
Oryza sativa | Fungal stress | OSERF922 | Negative regulator of blast disease | Wang et al. (2016a) | |
SEC3A, OsERF | Magnaporthe oryzae disease resistance | Ma et al. (2018) | |||
ALB1, RSY1 | Fungal disease resistance | Foster et al. (2018) | |||
Pi21, Host S-gene | Blast disease resistance | Nawaz et al. (2020) | |||
Oryza sativa | Viral stress | STA, UvSLT2 | Smut resistance | Liang et al. (2018) | |
eIF4G | Resistance to rice tungrospherical virus (RTSV) | Macovei et al. (2018) | |||
Oryza sativa | Bacterial stress | OsMPK5 | Bacterial blight resistance | Xie and Yang (2013) | |
Bsr-d1, Pi21 and ERF922 | Bacterial blight | Zhou et al. (2022) | |||
XA13promoter | Xanthomonas oryzae pv. oryzae | Li et al. (2020b) | |||
OsSWEET11, OsSWEET14, OsSWEET13 | Sucrose efflux transporter genes provide resistance to blight | Jiang et al. (2013), Oliva et al. (2019) | |||
Xa13/Host S-gene | Bacterial resistance | Li et al. (2020b) | |||
Oryza sativa | Herbicide stress | ALS | Herbicide resistance | Sun et al. (2017) | |
ALS1, FT1p1E | Imazamox herbicide resistance | Shimatani et al. (2017) | |||
ACC | Herbicide resistance | Liu et al. (2020a) | |||
EPSPS | Herbicide tolerance | Li et al. (2016) | |||
Oryza sativa | Insect stress | Cytochrome (P450 71A1) | Plant hopper | Lu et al. (2018) | |
OsCYP71A1 | Stem borer | Lu et al. (2018) | |||
Triticum aestivum | Fungal stress | TaMLO | Mildew resistant locus | Shan et al. (2014) | |
TaMLO-A1 | Powdery mildew resistance | Wang et al. (2014) | |||
TaEDR1 | Resistance to powdery mildew disease | Zhang et al. (2017) | |||
NFXL1 | Fusarium head blight resistance | Brauer et al. (2020) | |||
Hordeum vulgare | Fungal stress | MORC | Fungal resistance | Kumar et al. (2018) | |
Hordeum vulgare | Viral stress | Rep/Rep, MP, IR, CP, | Resistance to a viral pathogen | Kis et al. (2019) | |
Zea mays | Fungal stress | LOX3 | Resistance to fungal infection | Pathi et al. (2020) | |
Zea mays | Herbicide stress | PAT | Resistance to herbicide | Shukla et al. (2009) | |
ALS2 | Chlorsulfuron resistance | Svitashev et al. (2016) | |||
AAD1 | Herbicide resistance | Ainley et al. (2013) |
Improving crop resilience against fungal pathogens
Fungal pathogens can cause disease in crops, such as rust, mildew, rot, and smut. CRISPR/Cas technology has been used to make cereal crops more resistant to pathogens by leveraging the pathways involved in crop–pathogen interactions (Gosavi et al. 2020). Ethylene-responsive factors (ERF) play a role in defending against Magnaporthe oryzae fungus (Cao et al. 2006) and OsERF922 has been identified as a blast disease negative regulator (Liu et al. 2012). CRISPR/Cas9 gene editing was implemented to enhance the resistance of japonica rice against blast disease by targeting the translation initiation codons of OsERF922 leading to indels (Langner et al. 2018). The study shows that mutations may be passed down to the T1 and T2 generations without causing any appreciable changes in agronomic traits, showing rice lines resistant to blast disease (Wang et al. 2016a). To enhance fungal disease resistance in rice, the OsSEC3A gene putative subunit was knocked out. This increased the salicylic acid level, and was involved in exocytosis, resulting in a pleiotropic phenotype, and activation of pathogenesis-associated genes (Ma et al. 2018). Another example is genetically modifying rice using CRISPR/SpCas9 to eliminate the BSR-K1 gene, conferring resistance against M. oryzae (Zhou et al. 2018). In addition to the knockout approach, another effective and expedient method of enhancing crop disease resistance is resistance (R) gene rectification via CRISPR/Cas9-based editing. For instance, it has been shown that resistance to M. oryzae is linked to SNP at 441 positions in the Pid2 recessive allele. Rice that carries the recessive gene Pid2 (M441) becomes susceptible to ZB15 blast isolate. Conversely, the rice variety Digu conferred resistance to ZB15 upon insertion of the R gene (Chen et al. 2006). A cytidine base editor was used to introduce a G to A substitution in the rice variety kitaake, thereby correcting the recessive Pid2 gene (Ren et al. 2018). The resistance to rice blast disease was improved by modifying the Pyricularia oryzae 21 resistance gene (Pi21) (Nawaz et al. 2020). To enhance resistance against smut disease and rice blast, specific fungal genes such as RSY1, ALB1 (Foster et al. 2018), USTA, and UvSLT2 have been knocked out using CRISPR/Cas genome editing technique (Liang et al. 2018).
Wheat mildew is manifested by the fungus Blumeria graminis f. sp. tritici. The MLO resistance locus is an identified S gene region that was first discovered in Hordeum vulgare (Jørgensen 1992). CRISPR/Cas9 has been utilised successfully to edit homoeoalleles of the MLO in wheat, resulting in the development of varieties that are resistant to powdery mildew disease (Wang et al. 2014). Furthermore, Zhang et al. (2017) have also used CRISPR/Cas9 editing to modify the TaEDR1 gene (Enhanced Disease Resistance 1), leading to the generation of wheat resistance to powdery mildew (Zhang et al. 2017). Likewise, Fusarium head blight resistance was developed by targeting three wheat genes. These genes include a lipid transfer protein (TansLTP9.4), an ABC transporter (TaABCC6), and a nuclear transcription factor X box binding protein 1 (TaNFXL1). CRISPR-based knockout of the TaNFXL1 gene resulted in increased resistance to Fusarium head blight (Brauer et al. 2020). The gene editing technology offers an advancement in disease-resistance breeding programs surpassing the effectiveness of marker-assisted selection and traditional breeding methods.
Enhancing crop resistance against bacterial pathogens
Bacterial pathogens are responsible for causing different diseases and also produce numerous metabolites, such as polysaccharides, toxins, hormones, and pectic compounds. Plant cells release Type III effectors during the bacterial infection (Buttner and He 2009). These effectors mainly interfere with the host’s natural guard mechanisms or trigger susceptibility genes (known as S genes) that contribute to the development of diseases (Zaidi et al. 2018). Consequently, CRISPR/Cas9-based editing can effectively target both negative regulators of plant immune response and S genes to enhance bacterial disease resistance in cereal crops. OsSWEET13 encodes a sucrose transporter that plays a key role in the interaction between plants and pathogens. The effector protein PthXo2X increases the expression of OsSWEET13 in the host (Li et al. 2012). In an earlier report, a successful mutagenesis technique based on TALEN disrupted the OsSWEET14 gene, preventing binding between Xanthomonas oryzae effectors and OsSWEET14 resulting in a disease-resistant rice (Blanvillain-Baufumé et al. 2017). CRISPR-based mutagenesis was used to improve the rice plant’s resistance against blight caused by Xanthomonas oryzae pv. oryzae (Xoo) by targeting the OsSWEET13 gene (Oliva et al. 2019). By targeting transcription activator-like effectors (TALE) binding elements in OsSWEET11, it was possible to develop rice lines with mutations that conferred resistance against most of the Xoo strains (Xu et al. 2019). Furthermore, multiplex gene editing of OsSWEET11, OsSWEET13, and OsSWEET14 proved successful in developing resistance to blight in rice (Zhou et al. 2015). The mutant cereal crops developed using targeted genome editing can effectively combat stress and were explored by Li et al. (2017) and Kim et al. (2019), particularly for bacterial disease resistance. Advanced genome editing techniques have been used to attain resistance to bacterial blight involving combining the effectors and resistance genes (R genes) in a multiplex manner (Kim et al. 2019).
Strategies for mitigating resistance to insect and viral pathogens
The extensive applications of insecticides in agriculture present a threat to both well-being and the environment. To address this issue agriculturalists are now embracing biological methods and growing crops that are resistant to insects. Developing genetically engineered crops with built-in resistance to insect pests is an effective approach for safeguarding crops and promoting sustainable agriculture (Moon et al. 2022; Yang et al. 2024). Numerous research groups have concentrated their efforts on editing the genomes of disease-causing insects by targeting sex-associated genes. Genome editing has revolutionised insect genomics. For instance, advancements such as homology-assisted genome knock-in (HACK), DNA base editors, chromosomal translocation, and gene drives have the potential to eradicate disease-carrying insects. According to Gunasekara et al. (2017), the insecticidal protein Cry2A was introduced into rice plants, and it resulted in a death rate of 80% for the rice leaf folder. Genome editing has shown successful knockdown of insecticidal genes in maize and rice.
Cereal crops are susceptible to viral infections, resulting in substantial agricultural losses on a global scale. Controlling viruses through chemical means is extremely challenging because of their highly regulated intracellular process of causing disease (Ansari et al. 2020). CRISPR-based based gene-editing has undergone rapid development for engineering viral pathogen resistance. Virus resistance could be achieved by targeting components that are essential for viral replication and eliminating the genome of the virus, hence halting the replication process (Cao et al. 2020). Therefore, it is imperative to employ advanced methods to expedite the growth of crop plants that are resistant to viruses. Macovei et al. (2018) developed a novel method to enhance resistance to rice tungro virus (RTSV) by inducing mutations in the eIF4G alleles in rice crops (Macovei et al. 2018). The T2 plants obtained were transgene-free and exhibited resistance against RTSV. Moreover, no noticeable changes or mutations were observed in off-targets. To effectively control the transmission of infections it is possible to modify genes within the host that encode the necessary response factors for these viruses. Other research studies have also demonstrated that the SpCas9 CRISPR system can effectively target conserved regions within viral genomes, successfully conferring resistance to wheat dwarf virus (WDV) in barley (Kis et al. 2019). Similar strategies have been successfully employed to develop resistance to streaked draft virus and stripe mosaic virus in rice (Zhang et al. 2019b).
Improving cereal crops’ resilience to abiotic stresses via genome editing
Abiotic stress involves the unfavourable environmental conditions that plants frequently experience. These conditions can involve variables such as intense sunlight, elevated carbon dioxide levels, restricted availability of soil minerals, excessive or insufficient water supplies, harsh temperatures, and the existence of toxins in the soil. These elements exert a substantial influence on the many properties of plants, resulting in substantial negative impacts on their growth and productivity. Abiotic stress has significantly reduced agricultural productivity by up to 50% (Schneider et al. 2018). The environment has a substantial role in causing abiotic stress in plants, which can be brought on by changes in the weather (Mittler and Blumwald 2010). A considerable scientific emphasis has been placed on undertaking thorough research into the mechanisms by which plants endure, adapt to new habitats, and cope with different stress conditions (Kopecká et al. 2023) (Table 3).
Crop | Stress | Target gene | Role | References | |
---|---|---|---|---|---|
Oryza sativa | Salt stress | OsbHLH024 | Disrupting the OsbHLH024 gene leads to increased expression of ion transporter genes | Alam et al. (2022) | |
OsRR22 | Gene mutation enhances salt tolerance in rice. | Zhang et al. (2019a) | |||
OsRAV2 | Mutants demonstrated enhanced survival viability under salt stress | Liu et al. (2020b) | |||
OsNAC041 | Improves salt stress in crops | Wang et al. (2016b) | |||
OsBBS1 | Provides tolerance against salt stress | Zeng et al. (2018) | |||
OsPQT3 | Knockout mutants exhibited enhanced salinity and oxidative stress | Alfatih et al. (2020) | |||
Drought stress | OsERA1 | Mutations in OsERA1 resulted in drought stress tolerance in plants | Ogata et al. (2020) | ||
OsDST | Mutations in gene lead to broad leaves, reduced stomatal density and water retention in plants during drought stress | Santosh Kumar et al. (2020) | |||
OsPYL9 | Mutations in gene improved tolerance in drought and rice yield | Usman et al. (2020) | |||
OsSAPK2 | The mutants showed less drought sensitivity than wild type plants | Lou et al. (2018) | |||
Cold stress | OsPIN5b | Plants with ospin5b, gs3, and osmyb30 mutations displayed enhanced spike length, grain size, and demonstrated improved cold tolerance | Zeng et al. (2020) | ||
GS3 | |||||
OsMYB30 | |||||
OsAnn3 | Knocking out OsAnn3 and OsAnn5 produced plants sensitive to cold treatments | Shen et al. (2017) | |||
OsPRP1 | Knocking out the gene produced plants susceptible towards cold | Nawaz et al. (2019) | |||
Heat stress | OsPYL1/4/6 | Mutations in gene exhibited heat tolerance | Miao et al. (2018) | ||
OsPDS | Mutations in OsPDS developed heat tolerance in plants | Nandy et al. (2019) | |||
OsHSA1 | OsHSA1 mutants exhibited heat tolerance in plants | Qiu et al. (2018) | |||
Metal stress (Cadmium) | OsNRAMP5 | Knocking down OsNramp5/1 and OsLCT1 exhibited reduced cadmium (Cd) accumulation in rice | Chang et al. (2020), Chu et al. (2022) | ||
OsLCT1 | |||||
OsNRAMP1 | |||||
OsATX1 | OsATX1 knockout plants had reduced accumulation of caesium in plant roots | Zhang et al. (2021) | |||
Metal stress (Arsenic) | OsARM1 | Repressed the absorption and accumulation of arsenic in mutants | Wang et al. (2017) | ||
OSHAK1 | K+ transporter inactivation led to insensitivity towards arsenic | Nieves-Cordones et al. (2017) | |||
Hordeum vulgare | Salt stress | HvITPK1 | Gene mutations have the potential to enhance the resistance of crops against salinity | Vlčko and Ohnoutková (2020) | |
Triticum aestivum | Drought stress | TaDREB2, TaDREB3 TaERF3 | Mutations in genes lead to improved drought tolerance in wheat | Kim et al. (2018) | |
Zea mays | Drought stress | ZmARGOS8 | GOS2 promoter sequence was replaced with the intention of augmenting yield in maize during drought stress | Shi et al. (2017) |
The primary challenge is determining how these plants respond to various types of stress, which activate certain pathways and control the key genes involved in these pathways (Lamers et al. 2020; Zhang et al. 2023). Photosynthesis and other genetic or physiological mechanisms are the only ways for plants to sustain or develop their ability to deal with these stress conditions. Resistance from various stress factors is achieved by modulating the expression of a variety of genes involved in biochemical pathways. Molecular, biochemical, cellular, and physiological processes are triggered by sudden changes in nonliving environmental factors like light, temperature, or air humidity. The potential of genome editing in cereal crops against multiple abiotic stresses is substantial as shown in Fig. 3. CRISPR/Cas9 technology has been used to make specific genetic modifications in many grass plant species such as rice, sorghum, wheat, and switchgrass (Panicum virgatum) (Hussain et al. 2018; Zia et al. 2021; Han et al. 2022; Hasanuzzaman and Fujita 2022; Li et al. 2022; Rehman et al. 2022). We have reviewed various studies in this paper that have addressed abiotic stress in cereal crops.
The potential of genome editing in cereal crops against multiple stress factors. Genome editing in cereal crops against various biotic and abiotic factors has been done extensively in rice; followed by wheat, maize, and barley, as shown in numeric order.
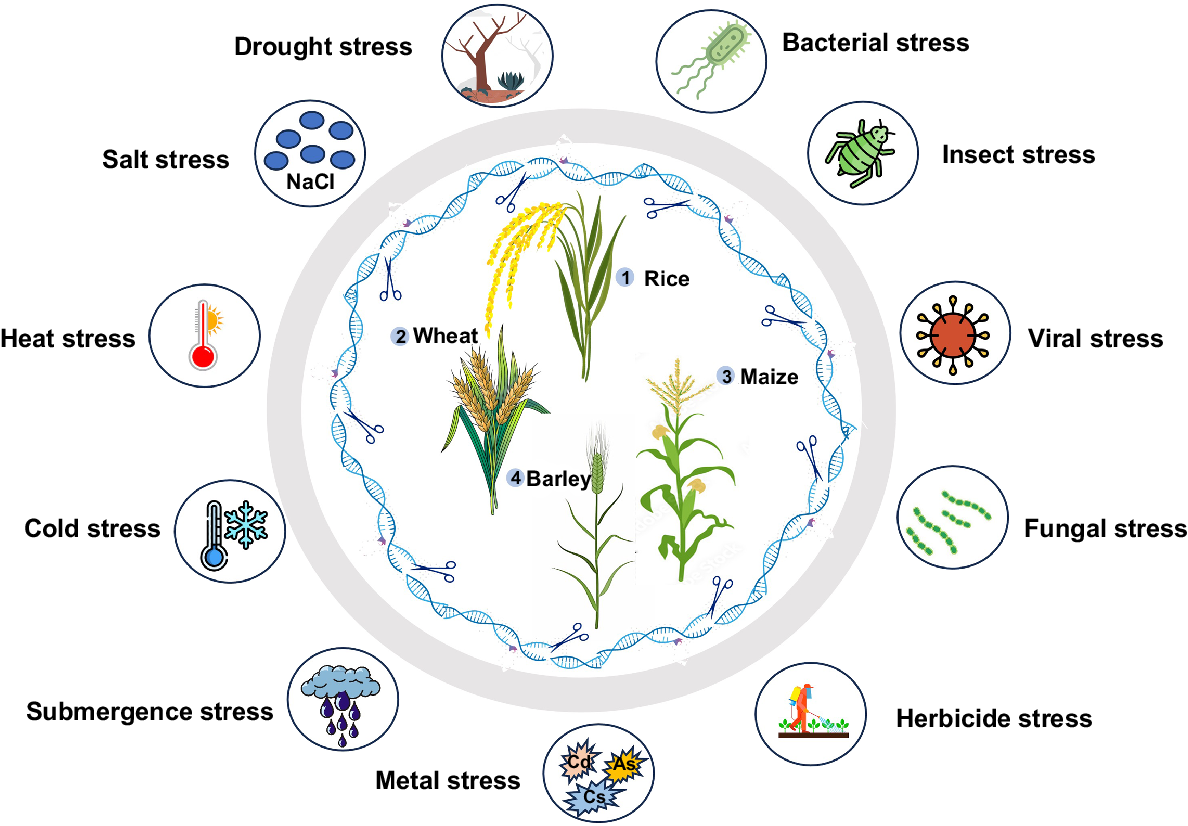
Enhancing drought tolerance in cereal crops
Drought stress has an undesirable impact on crop growth and yield by interrupting biochemical and physiological processes (Fahad et al. 2017; Sallam et al. 2019). Insufficient water supply can result in a fall in shoot biomass, foremost to a subsequent reduction in grain yield. Water stress is the main factor leading to decreased agricultural yields in comparison to other forms of stress. The intensity of drought stress is worsened by the evaporation of water in the root zone, resulting in an elevation of the vapour pressure deficit (Seleiman et al. 2021; Zia et al. 2021). Like other environmental stresses, this stressor causes major reductions in agricultural productivity. The length and severity of drought-induced stress are two of the several ecological factors that substantially impact agricultural production.
The extent to which agricultural productivity is reduced is governed by various factors, such as plant species, growth stage, severity, and duration of drought. Various genome editing studies have been conducted to address drought stress in cereal crops. The CRISPR/Cas9 genome editing technique was used to induce mutations into the SAPK genes of rice and wheat. This process was regulated by the SAPK promoter. The mutant plants exhibited the capacity to alleviate the effects of reactive oxygen species (ROS) formed due to stress induced by drought (Lou et al. 2017, 2018). Improved drought tolerance was achieved in wheat plants with the application of CRISPR/Cas-mediated alterations to TaDREB2 and TaERF3 genes (Kim et al. 2018; Akbar et al. 2023). A mutant of the indica mega rice cultivar was produced when Santosh Kumar et al. (2020) modified the OsDST gene using CRISPR/Cas9. Under drought conditions, this mutant exhibited wider leaves, reduced stomatal concentration, and improved water retention capacity in the leaves (Santosh Kumar et al. 2020).
Augmenting cereal crop resilience to cold and heat stress
Every crop has a distinct optimal temperature for its growth. Cold stress arises from a considerable reduction in temperatures, whereas heat stress arises from an extreme increase in temperatures. Temperate and subtropical species, such as grains, sometimes encounter chilling stress when exposed to temperatures between 0 and 10°C. Genome editing is a method employed to manipulate genetic material, which has the potential to trigger cold shocks. Cereal yield is decreased because of the 64 early reproductive phases that occur, which also cause pollen and ovule function to be compromised and flower growth to cease. This also has a negative effect on metabolic rates, which decreases grain yields (Fatima et al. 2020). Substantial changes in membrane permeability, free proline concentration, and malonic dialdehyde (MDA) levels were identified due to exposure to low temperatures (Guan et al. 2023). The occurrence of low temperatures caused by frost impedes the process of photosynthesis and raises the probability of internal harm, consequential in the generation of ROS (Bhattacharya 2022).
Elevated temperatures have an important impact on various physiological processes. Respiration, photosynthesis, and the production of ROS are among these processes (Mittler et al. 2022). Flowers have a low threshold value, which makes them more susceptible to heat stress during the blooming stage. This stress has a negative impact on seed production and grain yield (Scaven and Rafferty 2013; Zahra et al. 2023). The CRISPR/Cas9 system was utilised to generate the osmyb30, gs3, and ospin5b mutants, which demonstrated enhancements in cold tolerance, particle size, and spike length (Zeng et al. 2020). The entire growth cycle of crops is significantly impacted by extreme heat, particularly during heat-sensitive stages such as initial development and flowering (Jagadish et al. 2021). Not only heat, but cold also has a detrimental impact on crops. An investigation was conducted wherein the Ann3 gene was genetically modified utilising CRISPR/Cas9 technology in an effort to target and ameliorate cold sensitivity. Cold-tolerant plants with reduced electrical conductivity and enhanced resistance to cold stress were effectively generated through gene deletion facilitated by Agrobacterium-mediated transformation (Shen et al. 2017).
Improving crop resilience to salinity and submergence stress in cereal crops
Salt stress refers to the condition where the concentration of salt exceeds a specific threshold. Soil salinity is a highly damaging abiotic stressor. The detrimental impact of this stress on plant and cereal crops has been established by multiple studies (Laghmouchi et al. 2017; Sánchez-García et al. 2017; Zhao et al. 2021). Irrigated arable land accounted for 20% of total arable land, meaning that 80% of the region was unsuitable for agricultural crops, whereas 7% of the overall cultivable land area is experiencing adverse effects due to elevated salinity levels. The diminished agricultural yield is a direct outcome of this stress, which has reduced plant productivity due to inadequate nutrients. Approximately 14 billion acres (~5.6 billion hectares) of Earth’s land is classified as arid or semiarid, encompassing regions typically characterised by low precipitation, high evapotranspiration, and various forms of land degradation, including salinity issues. The area is characterised by a semiarid climate and salt degradation affects a land area of one billion hectares (Sha et al. 2022). Salt stress impacts 20% of cultivated land and 50% of irrigated land globally. The presence of high salinity levels has caused the deterioration of 14% of the cultivated regions in Pakistan, resulting in a substantial decline of 64% in agricultural productivity (Syed et al. 2020). Mutations in OsRAV2 led to the development of a mutant that showed an improved ability to survive under salt-stress conditions (Liu et al. 2020a). Salt tolerance in rice was increased by knocking down the OsbHLH024 gene and increasing the expression of ion transporter genes OsHKT1;3, OsHAK7, and OsSOS1 (Alam et al. 2022).
Submersion stress arises from the presence of still water. Waterlogging is caused by an excessive buildup of water, whereas drought is caused by a lack of sufficient water supply. Both factors, which disrupt plant biology, result in reduced agricultural productivity (Pan et al. 2021). During the early stage of the flowering cycle, the crop’s metabolic activities are highly sensitive and prone to being affected by external factors (El-Maarouf-Bouteau 2022). Waterlogging causes an increased need for metabolic energy, which leads to the emergence of various fungal infections. The halt in crop growth is due to decreased metabolic energy consumption through anaerobic respiration. The CRISPR/Cas9 technology was utilised to genetically edit genes involved in salt and submergence tolerance, the skilled application of CRISPR by specialists resulted in increased resistance to salt and enhanced agricultural traits (Li et al. 2022).
Improving the tolerance of cereal crops to heavy metal stress
The damaging effects of heavy metal contamination on plant growth and yield make it a substantial obstacle to the achievement of sustainable agricultural development. The presence of these elements has a negative impact on plant physiological and biochemical processes. This refers to the occurrence of oxidative and osmotic stress, disruption of tissue, ion imbalance, cytotoxicity, and disturbance of metabolic equilibrium (Hoque et al. 2021). Plants contaminated due to the accumulation of heavy metals pose serious health hazards to individuals. Various studies have been performed in plants to deal with heavy metal stress. The disruption of OsLCT1 and OsNRAMP5 genes by utilising CRISPR/Cas9 technology resulted in less cadmium (Cd) build-up in rice (Songmei et al. 2019). In another study, lower levels of lead (Pb) and Cd were found in rice grains because of the OsNRAMP1 gene disruption (Chang et al. 2020; Chu et al. 2022). OsARM1, a specific R2R3 MYB transcription factor, was effectively eliminated by Wang et al. (2017) using CRISPR/Cas9, which limited the transport and assimilation of arsenic in rice (Wang et al. 2017). Likewise, in another study, caesium (Cs) levels in rice crops were lowered by deactivating the transporter gene (OsHAK1) (Nieves-Cordones et al. 2017). The activation or deactivation of particular genes are certainly critical stages in such adaptive responses.
Strategies for herbicide resistance in cereal crops
Herbicide tolerance is currently considered one of the most important traits in cereal crops. To ensure weed control, it is crucial to prioritise genetically modifying crops with multiple genes that provide herbicide tolerance (Hulme 2022). Genome editing using endonucleases was demonstrated by D’Halluin et al. (2008), who successfully achieved the insertion of a 35S promoter of a promoter-less herbicide-resistant transgene in maize through the use of a meganuclease. Maize ALS genes were modified using base editing, resulting in the development of a variety that can tolerate herbicides like sulfonylurea, imidazolinone, and aryloxyphenoxy propionate (Li et al. 2017). The introduction of herbicide mutations at the SuR locus was achieved successfully using ZFN (Zhang et al. 2019c). For instance, the Cas9-based CRISPR method was utilised to insert the OsEPSPS gene into rice plants, conferring glyphosate resistance (Li et al. 2016). Furthermore, CRISPR/Cas9 was used to induce mutations in acetolactate synthase genes (ALS) in rice, resulting in two amino acid substitutions that ultimately granted herbicide tolerance. Additionally, knocking down ALS2 in maize using sgRNA/Cas9 was found to confer herbicide tolerance (Svitashev et al. 2016). CRISPR-based point mutation was found in the ALS gene causing the amino acid A96V substitution. Moreover, point mutations (W483 and G590) were inserted into the FTIP1e gene granting resistance to imazamox (Shimatani et al. 2017).
Conclusion
Creative solutions are needed to meet the task of quadrupling global agricultural production by 2050 in the face of climate change. One such possibility is to improve the agronomic properties of cereals, which are essential for ensuring global food security. A potential approach to creating robust crop types that can resist biotic stressors like pests and diseases and abiotic stressors like heat, salt, and drought is genome editing, particularly with the use of CRISPR/Cas9 technology. Through the targeted manipulation of particular genes linked to stress tolerance and disease resistance pathways, genome editing has the potential to expedite the development of more sustainable and productive grain varieties. It will need further investigation and implementation of these methods to fulfil future food demands sustainably and lessen the effects of climate change on agriculture.
Limitations and future recommendations
Genome editing technologies like CRISPR/Cas9 enable precise alterations to DNA, they offer great potential for advancements in fundamental research, agriculture, and medicine. They do, however, confront considerable obstacles and limits. These include the intricacy of transporting editing machinery into target cells, off-target consequences, undesired modifications in the genome, and challenges in generating uniform edits across all cells (mosaicism). The use of genome editing in humans raises ethical issues regarding safety, equality, and long-term effects on future generations, especially regarding germline cells. Moreover, the intricacy of genetic characteristics and the possibility of unforeseen outcomes highlight the need for cautious deliberation and strong regulatory structures to steer the proper utilisation and advancement of these potent technologies.
CRISPR/Cas-based genetic modification has emerged as a game changer in recent years because of its incredible capacity to induce predicted gene changes as well as for a range of diagnostic applications. Breakthroughs in technology are being utilised not just to generate knockouts but also knock-ins, as well as to activate and decrease gene expression. Because of its usefulness in real-world contexts, many innovations have occurred in a very short time after its discovery. These include DNA-free genome editing systems (RNPs), various Cas9 variations, a wide range of multigene targeting techniques, and efforts to increase the frequency of HDR. This very efficient approach to genome editing is becoming more popular because of recent advances in the computational tools linked with the CRISPR/Cas system. However, the difficulties of providing enough information to the public regarding the CRISPR/Cas-mediated approach to genetically editing plants should not be underestimated. This is because bringing laboratory research findings to the broader population is critical to ensuring agriculture’s long-term viability. It is critical to have rules that clearly distinguish between gene-edited plants that include foreign genes and those that do not contain any foreign DNA. This will free the latter from any limitations, making the application of such plants straightforward. Such laws are very necessary in this situation. In contrast to the commercial approval of transgene-free genome-edited crops with a unique mutation, the official launch of genome-edited plants with a genetic modification equivalent to those seen in natural variations will be more lenient.
Declaration of funding
This work is supported by the Ministry of National Food Security and Research of Pakistan released for the PSDP-project ‘Sino-Pak Agricultural Breeding Innovations Project for Rapid Yield Enhancement’ (857).
Author contributions
All authors equally contributed to conceptualisation, literature collection, resources, data curation, writing – original draft preparation, writing – review and editing, and visualisation. Supervision and project administration, MRK; funding acquisition, MRK and MU. All authors have read and agreed to the published version of the manuscript.
Acknowledgements
The authors acknowledge the research facilities provided by the Functional Genomics and Bioinformatics Group, National Institute for Genomics and Advanced Biotechnology (NIGAB), Pakistan.
References
Ahmad M (2023) Plant breeding advancements with “CRISPR-Cas” genome editing technologies will assist future food security. Frontiers in Plant Science 14, 1133036.
| Crossref | Google Scholar | PubMed |
Ainley WM, Sastry-Dent L, Welter ME, Murray MG, Zeitler B, Amora R, Corbin DR, Miles RR, Arnold NL, Strange TL, Simpson MA, Cao Z, Carroll C, Pawelczak KS, Blue R, West K, Rowland LM, Perkins D, Samuel P, Dewes CM, Shen L, Sriram S, Evans SL, Rebar EJ, Zhang L, Gregory PD, Urnov FD, Webb SR, Petolino JF (2013) Trait stacking via targeted genome editing. Plant Biotechnology Journal 11, 1126-1134.
| Crossref | Google Scholar | PubMed |
Akbar M, Aleem K, Sandhu K, Shamoon F, Fatima T, Ehsan M, Shaukat F (2023) A mini review on insect pests of wheat and their management strategies. International Journal of Agriculture and Biosciences 12, 110-115.
| Crossref | Google Scholar |
Akram F, Sahreen S, Aamir F, Haq Iu, Malik K, Imtiaz M, Naseem W, Nasir N, Waheed HM (2023) An insight into modern targeted genome-editing technologies with a special focus on CRISPR/Cas9 and its applications. Molecular Biotechnology 65, 227-242.
| Crossref | Google Scholar | PubMed |
Alam MS, Kong J, Tao R, Ahmed T, Alamin M, Alotaibi SS, Abdelsalam NR, Xu J-H (2022) CRISPR/Cas9 mediated knockout of the OsbHLH024 transcription factor improves salt stress resistance in rice (Oryza sativa L.). Plants 11, 1184.
| Crossref | Google Scholar | PubMed |
Alfatih A, Wu J, Jan SU, Zhang Z-S, Xia J-Q, Xiang C-B (2020) Loss of rice PARAQUAT TOLERANCE 3 confers enhanced resistance to abiotic stresses and increases grain yield in field. Plant, Cell & Environment 43, 2743-2754.
| Crossref | Google Scholar | PubMed |
Ansari WA, Chandanshive SU, Bhatt V, Nadaf AB, Vats S, Katara JL, Sonah H, Deshmukh R (2020) Genome editing in cereals: approaches, applications and challenges. International Journal of Molecular Sciences 21, 4040.
| Crossref | Google Scholar | PubMed |
Arora L, Narula A (2017) Gene editing and crop improvement using CRISPR-Cas9 system. Frontiers in Plant Science 8, 1932.
| Crossref | Google Scholar | PubMed |
Asmamaw M, Zawdie B (2021) Mechanism and applications of CRISPR/Cas-9-mediated genome editing. Biologics: Targets and Therapy 15, 353-361.
| Google Scholar |
Basu U, Riaz Ahmed S, Bhat BA, Anwar Z, Ali A, Ijaz A, Gulzar A, Bibi A, Tyagi A, Nebapure SM, Goud CA, Ahanger SA, Ali S, Mushtaq M (2023) A CRISPR way for accelerating cereal crop improvement: progress and challenges. Frontiers in Genetics 13, 866976.
| Crossref | Google Scholar | PubMed |
Bharat SS, Li S, Li J, Yan L, Xia L (2020) Base editing in plants: current status and challenges. The Crop Journal 8, 384-395.
| Crossref | Google Scholar |
Bhardwaj A, Nain V (2021) TALENs—an indispensable tool in the era of CRISPR: a mini review. Journal of Genetic Engineering and Biotechnology 19, 125.
| Crossref | Google Scholar | PubMed |
Bhuyan SJ, Kumar M, Devde PR, Rai AC, Mishra AK, Singh PK, Siddique KHM (2023) Progress in gene editing tools, implications and success in plants: a review. Frontiers in Genome Editing 5, 1272678.
| Crossref | Google Scholar |
Bisht DS, Bhatia V, Bhattacharya R (2019) Improving plant-resistance to insect-pests and pathogens: the new opportunities through targeted genome editing. Seminars in Cell & Developmental Biology 96, 65-76.
| Crossref | Google Scholar |
Blanvillain-Baufumé S, Reschke M, Solé M, Auguy F, Doucoure H, Szurek B, Meynard D, Portefaix M, Cunnac S, Guiderdoni E, Boch J, Koebnik R (2017) Targeted promoter editing for rice resistance to Xanthomonas oryzae pv. oryzae reveals differential activities for SWEET 14-inducing TAL effectors. Plant Biotechnology Journal 15, 306-317.
| Crossref | Google Scholar | PubMed |
Brauer EK, Balcerzak M, Rocheleau H, Leung W, Schernthaner J, Subramaniam R, Ouellet T (2020) Genome editing of a deoxynivalenol-induced transcription factor confers resistance to Fusarium graminearum in wheat. Molecular Plant-Microbe Interactions 33, 553-560.
| Crossref | Google Scholar | PubMed |
Buttner D, He SY (2009) Type III protein secretion in plant pathogenic bacteria. Plant Physiology 150, 1656-1664.
| Crossref | Google Scholar | PubMed |
Cao Y, Song F, Goodman RM, Zheng Z (2006) Molecular characterization of four rice genes encoding ethylene-responsive transcriptional factors and their expressions in response to biotic and abiotic stress. Journal of Plant Physiology 163, 1167-1178.
| Crossref | Google Scholar | PubMed |
Cao Y, Zhou H, Zhou X, Li F (2020) Control of plant viruses by CRISPR/Cas system-mediated adaptive immunity. Frontiers in Microbiology 11, 593700.
| Crossref | Google Scholar | PubMed |
Cardi T, Neal Stewart C, Jr (2016) Progress of targeted genome modification approaches in higher plants. Plant Cell Reports 35, 1401-1416.
| Crossref | Google Scholar | PubMed |
Chandrasegaran S, Carroll D (2016) Origins of programmable nucleases for genome engineering. Journal of Molecular Biology 428, 963-989.
| Crossref | Google Scholar | PubMed |
Chang J-D, Huang S, Yamaji N, Zhang W, Ma JF, Zhao F-J (2020) OsNRAMP1 transporter contributes to cadmium and manganese uptake in rice. Plant, Cell and Environment 43, 2476-2491.
| Crossref | Google Scholar |
Chen X, Shang J, Chen D, Lei C, Zou Y, Zhai W, Liu G, Xu J, Ling Z, Cao G, Ma B, Wang Y, Zhao X, Li S, Zhu L (2006) A B-lectin receptor kinase gene conferring rice blast resistance. The Plant Journal 46, 794-804.
| Crossref | Google Scholar | PubMed |
Chen K, Wang Y, Zhang R, Zhang H, Gao C (2019) CRISPR/Cas genome editing and precision plant breeding in agriculture. Annual Review of Plant Biology 70, 667-697.
| Crossref | Google Scholar | PubMed |
Chu C, Huang R, Liu L, Tang G, Xiao J, Yoo H, Yuan M (2022) The rice heavy-metal transporter OsNRAMP1 regulates disease resistance by modulating ROS homoeostasis. Plant, Cell and Environment 45, 1109-1126.
| Crossref | Google Scholar |
Costa JR, Bejcek BE, McGee JE, Fogel AI, Brimacombe KR, Ketteler R (2017) Genome editing using engineered nucleases and their use in genomic screening. In ‘Assay Guidance Manual’. (Eds S Markossian, A Grossman, M Arkin, et al.) pp. 931–954. (Eli Lilly & Company and the National Center for Advancing Translational Sciences: Bethesda, MD, USA)
Craig W, Tepfer M, Degrassi G, Ripandelli D (2008) An overview of general features of risk assessments of genetically modified crops. Euphytica 164, 853-880.
| Crossref | Google Scholar |
D’Halluin K, Vanderstraeten C, Stals E, Cornelissen M, Ruiter R (2008) Homologous recombination: a basis for targeted genome optimization in crop species such as maize. Plant Biotechnology Journal 6, 93-102.
| Crossref | Google Scholar | PubMed |
El-Maarouf-Bouteau H (2022) The seed and the metabolism regulation. Biology 11, 168.
| Crossref | Google Scholar | PubMed |
Fahad S, Bajwa AA, Nazir U, Anjum SA, Farooq A, Zohaib A, Sadia S, Nasim W, Adkins S, Saud S, Ihsan MZ, Alharby H, Wu C, Wang D, Huang J (2017) Crop production under drought and heat stress: plant responses and management options. Frontiers in Plant Science 8, 1147.
| Crossref | Google Scholar |
Fatima Z, Ahmed M, Hussain M, Abbas G, Ul-Allah S, Ahmad S, Ahmed N, Ali MA, Sarwar G, Haque Eu, Iqbal P, Hussain S (2020) The fingerprints of climate warming on cereal crops phenology and adaptation options. Scientific Reports 10, 18013.
| Crossref | Google Scholar |
Foster AJ, Martin-Urdiroz M, Yan X, Wright HS, Soanes DM, Talbot NJ (2018) CRISPR-Cas9 ribonucleoprotein-mediated co-editing and counterselection in the rice blast fungus. Scientific Reports 8, 14355.
| Crossref | Google Scholar | PubMed |
Gaj T, Gersbach CA, Barbas CF, III (2013) ZFN, TALEN, and CRISPR/Cas-based methods for genome engineering. Trends in Biotechnology 31, 397-405.
| Crossref | Google Scholar | PubMed |
Gao W, Xu W-T, Huang K-L, Guo M-z, Luo Y-B (2018) Risk analysis for genome editing-derived food safety in China. Food Control 84, 128-137.
| Crossref | Google Scholar |
González Castro N, Bjelic J, Malhotra G, Huang C, Alsaffar SH (2021) Comparison of the feasibility, efficiency, and safety of genome editing technologies. International Journal of Molecular Sciences 22, 10355.
| Crossref | Google Scholar | PubMed |
Gosavi G, Yan F, Ren B, Kuang Y, Yan D, Zhou X, Zhou H (2020) Applications of CRISPR technology in studying plant-pathogen interactions: overview and perspective. Phytopathology Research 2, 21.
| Crossref | Google Scholar |
Gu S, Bodai Z, Cowan QT, Komor AC (2021) Base editors: expanding the types of DNA damage products harnessed for genome editing. Gene and Genome Editing 1, 100005.
| Crossref | Google Scholar | PubMed |
Guan Y, Hwarari D, Korboe HM, Ahmad B, Cao Y, Movahedi A, Yang L (2023) Low temperature stress-induced perception and molecular signaling pathways in plants. Environmental and Experimental Botany 207, 105190.
| Crossref | Google Scholar |
Guha TK, Edgell DR (2017) Applications of alternative nucleases in the age of CRISPR/Cas9. International Journal of Molecular Sciences 18, 2565.
| Crossref | Google Scholar | PubMed |
Gunasekara JMA, Jayasekera GAU, Perera KLNS, Wickramasuriya AM (2017) Development of a Sri Lankan rice variety Bg 94-1 harbouring cry2A gene of Bacillus thuringiensis resistant to rice leaffolder [Cnaphalocrocis medinalis (Guenée)]. Journal of the National Science Foundation of Sri Lanka 45, 143-157.
| Crossref | Google Scholar |
Han X, Chen Z, Li P, Xu H, Liu K, Zha W, Li S, Chen J, Yang G, Huang J, You A, Zhou L (2022) Development of novel rice germplasm for salt-tolerance at seedling stage using CRISPR-Cas9. Sustainability 14, 2621.
| Crossref | Google Scholar |
Hasanuzzaman M, Fujita M (2022) Plant responses and tolerance to salt stress: physiological and molecular interventions. International Journal of Molecular Sciences 23, 4810.
| Crossref | Google Scholar | PubMed |
He Y, Zhao Y (2020) Technological breakthroughs in generating transgene-free and genetically stable CRISPR-edited plants. aBIOTECH 1, 88-96.
| Crossref | Google Scholar | PubMed |
Hille F, Richter H, Wong SP, Bratovič M, Ressel S, Charpentier E (2018) The biology of CRISPR-Cas: backward and forward. Cell 172, 1239-1259.
| Crossref | Google Scholar | PubMed |
Hisano H, Abe F, Hoffie RE, Kumlehn J (2021) Targeted genome modifications in cereal crops. Breeding Science 71, 405-416.
| Crossref | Google Scholar | PubMed |
Hoque MN, Tahjib-Ul-Arif M, Hannan A, Sultana N, Akhter S, Hasanuzzaman M, Akter F, Hossain MS, Sayed MA, Hasan MT, Skalicky M, Li X, Brestič M (2021) Melatonin modulates plant tolerance to heavy metal stress: morphological responses to molecular mechanisms. International Journal of Molecular Sciences 22, 11445.
| Crossref | Google Scholar | PubMed |
Huang Z, Liu G (2023) Current advancement in the application of prime editing. Frontiers in Bioengineering and Biotechnology 11, 1039315.
| Crossref | Google Scholar | PubMed |
Hulme PE (2022) Global drivers of herbicide-resistant weed richness in major cereal crops worldwide. Pest Management Science 78, 1824-1832.
| Crossref | Google Scholar | PubMed |
Hussain B, Lucas SJ, Budak H (2018) CRISPR/Cas9 in plants: at play in the genome and at work for crop improvement. Briefings in Functional Genomics 17, 319-328.
| Crossref | Google Scholar |
Jagadish SVK, Way DA, Sharkey TD (2021) Plant heat stress: concepts directing future research. Plant, Cell & Environment 44, 1992-2005.
| Crossref | Google Scholar | PubMed |
Janik E, Niemcewicz M, Ceremuga M, Krzowski L, Saluk-Bijak J, Bijak M (2020) Various aspects of a gene editing system—CRISPR–cas9. International Journal of Molecular Sciences 21, 9604.
| Crossref | Google Scholar | PubMed |
Jiang W, Zhou H, Bi H, Fromm M, Yang B, Weeks DP (2013) Demonstration of CRISPR/Cas9/sgRNA-mediated targeted gene modification in Arabidopsis, tobacco, sorghum and rice. Nucleic Acids Research 41, e188.
| Crossref | Google Scholar | PubMed |
Jørgensen IH (1992) Discovery, characterization and exploitation of Mlo powdery mildew resistance in barley. Euphytica 63, 141-152.
| Crossref | Google Scholar |
Kantor A, McClements ME, MacLaren RE (2020) CRISPR-Cas9 DNA base-editing and prime-editing. International Journal of Molecular Sciences 21, 6240.
| Crossref | Google Scholar | PubMed |
KhokharVoytas A, Shahbaz M, Maqsood MF, Zulfiqar U, Naz N, Iqbal UZ, Sara M, Aqeel M, Khalid N, Noman A, Zulfiqar F, Al Syaad KM, AlShaqhaa MA (2023) Genetic modification strategies for enhancing plant resilience to abiotic stresses in the context of climate change. Functional & Integrative Genomics 23, 283.
| Crossref | Google Scholar |
Kim D, Alptekin B, Budak H (2018) CRISPR/Cas9 genome editing in wheat. Functional & Integrative Genomics 18, 31-41.
| Crossref | Google Scholar | PubMed |
Kim Y-A, Moon H, Park C-J (2019) CRISPR/Cas9-targeted mutagenesis of Os8N3 in rice to confer resistance to Xanthomonas oryzae pv. oryzae. Rice 12, 71.
| Crossref | Google Scholar |
Kis A, Hamar É, Tholt G, Bán R, Havelda Z (2019) Creating highly efficient resistance against wheat dwarf virus in barley by employing CRISPR/Cas9 system. Plant Biotechnology Journal 17, 1004-1006.
| Crossref | Google Scholar | PubMed |
Koeppel I, Hertig C, Hoffie R, Kumlehn J (2019) Cas endonuclease technology—a quantum leap in the advancement of barley and wheat genetic engineering. International Journal of Molecular Sciences 20, 2647.
| Crossref | Google Scholar | PubMed |
Kopecká R, Kameniarová M, Černý M, Brzobohatý B, Novák J (2023) Abiotic Stress in Crop Production. International Journal of Molecular Sciences 24, 6603.
| Crossref | Google Scholar | PubMed |
Kumar N, Galli M, Ordon J, Stuttmann J, Kogel K-H, Imani J (2018) Further analysis of barley MORC1 using a highly efficient RNA-guided Cas9 gene-editing system. Plant Biotechnology Journal 16, 1892-1903.
| Crossref | Google Scholar | PubMed |
Laghmouchi Y, Belmehdi O, Bouyahya A, Skali Senhaji N, Abrini J (2017) Effect of temperature, salt stress and pH on seed germination of medicinal plant Origanum compactum. Biocatalysis and Agricultural Biotechnology 10, 156-160.
| Crossref | Google Scholar |
Lamers J, van der Meer T, Testerink C (2020) How Plants Sense and Respond to Stressful Environments. Plant Physiology 182, 1624-1635.
| Crossref | Google Scholar | PubMed |
Langner T, Kamoun S, Belhaj K (2018) CRISPR crops: plant genome editing toward disease resistance. Annual Review of Phytopathology 56, 479-512.
| Crossref | Google Scholar | PubMed |
Li T, Liu B, Spalding MH, Weeks DP, Yang B (2012) High-efficiency TALEN-based gene editing produces disease-resistant rice. Nature Biotechnology 30, 390-392.
| Crossref | Google Scholar | PubMed |
Li J, Meng X, Zong Y, Chen K, Zhang H, Liu J, Li J, Gao C (2016) Gene replacements and insertions in rice by intron targeting using CRISPR–Cas9. Nature Plants 2, 16139.
| Crossref | Google Scholar |
Li C, Liu C, Qi X, Wu Y, Fei X, Mao L, Cheng B, Li X, Xie C (2017) RNA-guided Cas9 as an in vivo desired-target mutator in maize. Plant Biotechnology Journal 15, 1566-1576.
| Crossref | Google Scholar | PubMed |
Li C, Li W, Zhou Z, Chen H, Xie C, Lin Y (2020a) A new rice breeding method: CRISPR/Cas9 system editing of the Xa13 promoter to cultivate transgene-free bacterial blight-resistant rice. Plant Biotechnology Journal 18, 313-315.
| Crossref | Google Scholar | PubMed |
Li H, Yang Y, Hong W, Huang M, Wu M, Zhao X (2020b) Applications of genome editing technology in the targeted therapy of human diseases: mechanisms, advances and prospects. Signal Transduction and Targeted Therapy 5, 1.
| Crossref | Google Scholar | PubMed |
Li Y, Wu X, Zhang Y, Zhang Q (2022) CRISPR/Cas genome editing improves abiotic and biotic stress tolerance of crops. Frontiers in Genome Editing 4, 987817.
| Crossref | Google Scholar |
Liang Y, Han Y, Wang C, Jiang C, Xu J-R (2018) Targeted deletion of the USTA and UvSLT2 genes efficiently in Ustilaginoidea virens with the CRISPR-Cas9 system. Frontiers in Plant Science 9, 699.
| Crossref | Google Scholar | PubMed |
Liu D, Chen X, Liu J, Ye J, Guo Z (2012) The rice ERF transcription factor OsERF922 negatively regulates resistance to Magnaporthe oryzae and salt tolerance. Journal of Experimental Botany 63, 3899-3911.
| Crossref | Google Scholar | PubMed |
Liu X, Qin R, Li J, Liao S, Shan T, Xu R, Wu D, Wei P (2020a) A CRISPR-Cas9-mediated domain-specific base-editing screen enables functional assessment of ACCase variants in rice. Plant Biotechnology Journal 18, 1845.
| Crossref | Google Scholar | PubMed |
Liu X, Wu D, Shan T, Xu S, Qin R, Li H, Negm M, Wu D, Li J (2020b) The trihelix transcription factor OsGTγ-2 is involved adaption to salt stress in rice. Plant Molecular Biology 103, 545-560.
| Crossref | Google Scholar | PubMed |
Liu Z, Ma C, Hou L, Wu X, Wang D, Zhang L, Liu P (2022) Exogenous SA affects rice seed germination under salt stress by regulating Na+/K+ balance and endogenous GAs and ABA homeostasis. International Journal of Molecular Sciences 23, 3293.
| Crossref | Google Scholar | PubMed |
Lou D, Wang H, Liang G, Yu D (2017) OsSAPK2 confers abscisic acid sensitivity and tolerance to drought stress in rice. Frontiers in Plant Science 8, 993.
| Crossref | Google Scholar | PubMed |
Lou D, Wang H, Yu D (2018) The sucrose non-fermenting-1-related protein kinases SAPK1 and SAPK2 function collaboratively as positive regulators of salt stress tolerance in rice. BMC Plant Biology 18, 203.
| Crossref | Google Scholar |
Lu Y, Zhu J-K (2017) Precise editing of a target base in the rice genome using a modified CRISPR/Cas9 system. Molecular Plant 10, 523-525.
| Crossref | Google Scholar | PubMed |
Lu H-P, Luo T, Fu H-W, Wang L, Tan Y-Y, Huang J-Z, Wang Q, Ye G-Y, Gatehouse AMR, Lou Y-G, Shu Q-Y (2018) Resistance of rice to insect pests mediated by suppression of serotonin biosynthesis. Nature Plants 4, 338-344.
| Crossref | Google Scholar |
Lyu P, Wang L, Lu B (2020) Virus-like particle mediated CRISPR/Cas9 delivery for efficient and safe genome editing. Life 10, 366.
| Crossref | Google Scholar | PubMed |
Ma J, Chen J, Wang M, Ren Y, Wang S, Lei C, Cheng Z, Sodmergen (2018) Disruption of OsSEC3A increases the content of salicylic acid and induces plant defense responses in rice. Journal of Experimental Botany 69, 1051-1064.
| Crossref | Google Scholar | PubMed |
Macovei A, Sevilla NR, Cantos C, Jonson GB, Slamet-Loedin I, Čermák T, Voytas DF, Choi I-R, Chadha-Mohanty P (2018) Novel alleles of rice eIF4G generated by CRISPR/Cas9-targeted mutagenesis confer resistance to Rice tungro spherical virus. Plant Biotechnology Journal 16, 1918-1927.
| Crossref | Google Scholar | PubMed |
Matres JM, Hilscher J, Datta A, Armario-Nájera V, Baysal C, He W, Huang X, Zhu C, Valizadeh-Kamran R, Trijatmiko KR, Capell T, Christou P, Stoger E, Slamet-Loedin IH (2021) Genome editing in cereal crops: an overview. Transgenic Research 30, 461-498.
| Crossref | Google Scholar | PubMed |
Miao C, Xiao L, Hua K, Zou C, Zhao Y, Bressan RA, Zhu J-K (2018) Mutations in a subfamily of abscisic acid receptor genes promote rice growth and productivity. Proceedings of the National Academy of Sciences 115, 6058-6063.
| Crossref | Google Scholar |
Miller JB, Zhang S, Kos P, Xiong H, Zhou K, Perelman SS, Zhu H, Siegwart DJ (2017) Non-viral CRISPR/Cas gene editing in vitro and in vivo enabled by synthetic nanoparticle co-delivery of Cas9 mRNA and sgRNA. Angewandte Chemie 129, 1079-1083.
| Crossref | Google Scholar |
Mittler R, Blumwald E (2010) Genetic engineering for modern agriculture: challenges and perspectives. Annual Review of Plant Biology 61, 443-462.
| Crossref | Google Scholar | PubMed |
Mittler R, Zandalinas SI, Fichman Y, Van Breusegem F (2022) Reactive oxygen species signalling in plant stress responses. Nature Reviews Molecular Cell Biology 23, 663-679.
| Crossref | Google Scholar | PubMed |
Mohanta T, Bashir T, Hashem A, Abd_Allah EF, Bae H (2017) Genome editing tools in plants. Genes 8, 399,.
| Crossref | Google Scholar | PubMed |
Moon TT, Maliha IJ, Khan AAM, Chakraborty M, Uddin MS, Amin MR, Islam T (2022) CRISPR-Cas Genome Editing for Insect Pest Stress Management in Crop Plants. Stresses 2, 493-514.
| Crossref | Google Scholar |
Nandy S, Pathak B, Zhao S, Srivastava V (2019) Heat-shock-inducible CRISPR/Cas9 system generates heritable mutations in rice. Plant Direct 3, e00145.
| Crossref | Google Scholar | PubMed |
Nawaz G, Han Y, Usman B, Liu F, Qin B, Li R (2019) Knockout of OsPRP1, a gene encoding proline-rich protein, confers enhanced cold sensitivity in rice (Oryza sativa L.) at the seedling stage. 3 Biotech 9, 254.
| Crossref | Google Scholar |
Nawaz G, Usman B, Peng H, Zhao N, Yuan R, Liu Y, Li R (2020) Knockout of Pi21 by CRISPR/Cas9 and iTRAQ-based proteomic analysis of mutants revealed new insights into M. oryzae resistance in elite rice line. Genes 11, 735.
| Crossref | Google Scholar | PubMed |
Nerkar G, Devarumath S, Purankar M, Kumar A, Valarmathi R, Devarumath R, Appunu C (2022) Advances in crop breeding through precision genome editing. Frontiers in Genetics 13, 880195.
| Crossref | Google Scholar | PubMed |
Nieves-Cordones M, Mohamed S, Tanoi K, Kobayashi NI, Takagi K, Vernet A, Guiderdoni E, Périn C, Sentenac H, Véry A-A (2017) Production of low-Cs+ rice plants by inactivation of the K+ transporter OsHAK1 with the CRISPR-Cas system. The Plant Journal 92, 43-56.
| Crossref | Google Scholar | PubMed |
Ogata T, Ishizaki T, Fujita M, Fujita Y (2020) CRISPR/Cas9-targeted mutagenesis of OsERA1 confers enhanced responses to abscisic acid and drought stress and increased primary root growth under nonstressed conditions in rice. PLoS ONE 15, e0243376.
| Crossref | Google Scholar | PubMed |
Oliva R, Ji C, Atienza-Grande G, Huguet-Tapia JC, Perez-Quintero A, Li T, Eom J-S, Li C, Nguyen H, Liu B, Auguy F, Sciallano C, Luu VT, Dossa GS, Cunnac S, Schmidt SM, Slamet-Loedin IH, Vera Cruz C, Szurek B, Frommer WB, White FF, Yang B (2019) Broad-spectrum resistance to bacterial blight in rice using genome editing. Nature Biotechnology 37, 1344-1350.
| Crossref | Google Scholar | PubMed |
Pan J, Sharif R, Xu X, Chen X (2021) Mechanisms of waterlogging tolerance in plants: research progress and prospects. Frontiers in Plant Science 11, 627331.
| Crossref | Google Scholar |
Park J, Choe S (2019) DNA-free genome editing with preassembled CRISPR/Cas9 ribonucleoproteins in plants. Transgenic Research 28, 61-64.
| Crossref | Google Scholar | PubMed |
Pathi KM, Rink P, Budhagatapalli N, Betz R, Saado I, Hiekel S, Becker M, Djamei A, Kumlehn J (2020) Engineering smut resistance in maize by site-directed mutagenesis of LIPOXYGENASE 3. Frontiers in Plant Science 11, 543895.
| Crossref | Google Scholar | PubMed |
Qiu Z, Kang S, He L, Zhao J, Zhang S, Hu J, Zeng D, Zhang G, Dong G, Gao Z, Ren D, Chen G, Guo L, Qian Q, Zhu L (2018) The newly identified heat-stress sensitive albino 1 gene affects chloroplast development in rice. Plant Science 267, 168-179.
| Crossref | Google Scholar | PubMed |
Rehman I, Shaukat F, Anwar Z, Ijaz A, Nadeem R (2022) Applications of Crispr/Cas system in plants. International Journal of Agriculture and Biosciences 11(4), 231-237.
| Google Scholar |
Ren B, Yan F, Kuang Y, Li N, Zhang D, Zhou X, Lin H, Zhou H (2018) Improved base editor for efficiently inducing genetic variations in rice with CRISPR/Cas9-guided hyperactive hAID mutant. Molecular Plant 11, 623-626.
| Crossref | Google Scholar |
Sallam A, Alqudah AM, Dawood MFA, Baenziger PS, Börner A (2019) Drought stress tolerance in wheat and barley: advances in physiology, breeding and genetics research. International Journal of Molecular Sciences 20, 3137.
| Crossref | Google Scholar | PubMed |
Sánchez-García EA, Rodríguez-Medina K, Moreno-Casasola P (2017) Effects of soil saturation and salinity on seed germination in seven freshwater marsh species from the tropical coast of the Gulf of Mexico. Aquatic Botany 140, 4-12.
| Crossref | Google Scholar |
Santosh Kumar VV, Verma RK, Yadav SK, Yadav P, Watts A, Rao MV, Chinnusamy V (2020) CRISPR-Cas9 mediated genome editing of drought and salt tolerance (OsDST) gene in indica mega rice cultivar MTU1010. Physiology and Molecular Biology of Plants 26, 1099-1110.
| Crossref | Google Scholar | PubMed |
Savary S, Willocquet L, Pethybridge SJ, Esker P, McRoberts N, Nelson A (2019) The global burden of pathogens and pests on major food crops. Nature Ecology & Evolution 3, 430-439.
| Crossref | Google Scholar | PubMed |
Scaven VL, Rafferty NE (2013) Physiological effects of climate warming on flowering plants and insect pollinators and potential consequences for their interactions. Current Zoology 59, 418-426.
| Crossref | Google Scholar | PubMed |
Schaeffer SM, Nakata PA (2015) CRISPR/Cas9-mediated genome editing and gene replacement in plants: transitioning from lab to field. Plant Science 240, 130-142.
| Crossref | Google Scholar | PubMed |
Schneider JR, Caverzan A, Chavarria G (2018) Water deficit stress, ROS involvement, and plant performance. Archives of Agronomy and Soil Science 65, 1160-1181.
| Crossref | Google Scholar |
Seleiman MF, Al-Suhaibani N, Ali N, Akmal M, Alotaibi M, Refay Y, Dindaroglu T, Abdul-Wajid HH, Battaglia ML (2021) Drought stress impacts on plants and different approaches to alleviate its adverse effects. Plants 10, 259.
| Crossref | Google Scholar | PubMed |
Sha Z, Bai Y, Li R, Lan H, Zhang X, Li J, Liu X, Chang S, Xie Y (2022) The global carbon sink potential of terrestrial vegetation can be increased substantially by optimal land management. Communications Earth & Environment 3, 8.
| Crossref | Google Scholar |
Shan Q, Wang Y, Li J, Gao C (2014) Genome editing in rice and wheat using the CRISPR/Cas system. Nature Protocols 9, 2395-2410.
| Crossref | Google Scholar | PubMed |
Shen C, Que Z, Xia Y, Tang N, Li D, He R, Cao M (2017) Knock out of the annexin gene OsAnn3 via CRISPR/Cas9-mediated genome editing decreased cold tolerance in rice. Journal of Plant Biology 60, 539-547.
| Crossref | Google Scholar |
Shi J, Gao H, Wang H, Lafitte HR, Archibald RL, Yang M, Hakimi SM, Mo H, Habben JE (2017) ARGOS8 variants generated by CRISPR-Cas9 improve maize grain yield under field drought stress conditions. Plant Biotechnology Journal 15, 207-216.
| Crossref | Google Scholar | PubMed |
Shimatani Z, Kashojiya S, Takayama M, Terada R, Arazoe T, Ishii H, Teramura H, Yamamoto T, Komatsu H, Miura K, Ezura H, Nishida K, Ariizumi T, Kondo A (2017) Targeted base editing in rice and tomato using a CRISPR-Cas9 cytidine deaminase fusion. Nature Biotechnology 35, 441-443.
| Crossref | Google Scholar | PubMed |
Shukla VK, Doyon Y, Miller JC, DeKelver RC, Moehle EA, Worden SE, Mitchell JC, Arnold NL, Gopalan S, Meng X, Choi VM, Rock JM, Wu Y-Y, Katibah GE, Zhifang G, McCaskill D, Simpson MA, Blakeslee B, Greenwalt SA, Butler HJ, Hinkley SJ, Zhang L, Rebar EJ, Gregory PD, Urnov FD (2009) Precise genome modification in the crop species Zea mays using zinc-finger nucleases. Nature 459, 437-441.
| Crossref | Google Scholar | PubMed |
Songmei L, Jie J, Yang L, Jun M, Shouling X, Yuanyuan T, Youfa L, Qingyao S, Jianzhong H (2019) Characterization and evaluation of OsLCT1 and OsNramp5 mutants generated through CRISPR/Cas9-mediated mutagenesis for breeding low Cd rice. Rice Science 26, 88-97.
| Crossref | Google Scholar |
Sun YW, Jiao GA, Liu ZP, Zhang X, Li JY, Guo XP, Du WM, Du JL, Francis F, Zhao YD, Xia LQ (2017) Generation of high-amylose rice through CRISPR/Cas9-mediated targeted mutagenesis of starch branching enzymes. Frontiers in Plant Science 8, 298.
| Crossref | Google Scholar | PubMed |
Svitashev S, Schwartz C, Lenderts B, Young JK, Mark Cigan A (2016) Genome editing in maize directed by CRISPR–Cas9 ribonucleoprotein complexes. Nature Communications 7, 13274.
| Crossref | Google Scholar | PubMed |
Syed A, Sarwar G, Shah SH, Muhammad S (2020) Soil salinity research in 21st century in Pakistan: its impact on availability of plant nutrients, growth and yield of crops. Communications in Soil Science and Plant Analysis 52, 183-200.
| Crossref | Google Scholar |
Tsanova T, Stefanova L, Topalova L, Atanasov A, Pantchev I (2021) DNA-free gene editing in plants: a brief overview. Biotechnology & Biotechnological Equipment 35, 131-138.
| Crossref | Google Scholar |
Usman B, Nawaz G, Zhao N, Liao S, Liu Y, Li R (2020) Precise editing of the OsPYL9 gene by RNA-guided Cas9 nuclease confers enhanced drought tolerance and grain yield in rice (Oryza sativa L.) by regulating circadian rhythm and abiotic stress responsive proteins. International Journal of Molecular Sciences 21, 7854.
| Crossref | Google Scholar | PubMed |
Van Esse HP, Reuber TL, van der Does D (2020) Genetic modification to improve disease resistance in crops. New Phytologist 225, 70-86.
| Crossref | Google Scholar | PubMed |
Vlčko T, Ohnoutková L (2020) Allelic variants of CRISPR/Cas9 Induced mutation in an inositol trisphosphate 5/6 kinase gene manifest different phenotypes in barley. Plants 9, 195.
| Crossref | Google Scholar |
Voytas DF (2013) Plant genome engineering with sequence-specific nucleases. Annual Review of Plant Biology 64, 327-350.
| Crossref | Google Scholar | PubMed |
Wang Y, Cheng X, Shan Q, Zhang Y, Liu J, Gao C, Qiu J-L (2014) Simultaneous editing of three homoeoalleles in hexaploid bread wheat confers heritable resistance to powdery mildew. Nature Biotechnology 32, 947-951.
| Crossref | Google Scholar | PubMed |
Wang F, Wang C, Liu P, Lei C, Hao W, Gao Y, Liu Y-G, Zhao K (2016a) Enhanced rice blast resistance by CRISPR/Cas9-targeted mutagenesis of the ERF transcription factor gene OsERF922. PLoS ONE 11, e0154027.
| Crossref | Google Scholar | PubMed |
Wang H, La Russa M, Qi LS (2016b) CRISPR/Cas9 in genome editing and beyond. Annual Review of Biochemistry 85, 227-264.
| Crossref | Google Scholar | PubMed |
Wang F-Z, Chen M-X, Yu L-J, Xie L-J, Yuan L-B, Qi H, Xiao M, Guo W, Chen Z, Yi K, Zhang J, Qiu R, Shu W, Xiao S, Chen Q-F (2017) OsARM1, an R2R3 MYB transcription factor, is involved in regulation of the response to arsenic stress in rice. Frontiers in Plant Science 8, 1868.
| Crossref | Google Scholar |
Wang Q, Guo Q, Niu W, Wu L, Gong W, Yan S, Nishinari K, Zhao M (2022) The pH-responsive phase separation of type-A gelatin and dextran characterized with static multiple light scattering (S-MLS). Food Hydrocolloids 127, 107503.
| Crossref | Google Scholar |
Wei T, Cheng Q, Min Y-L, Olson EN, Siegwart DJ (2020) Systemic nanoparticle delivery of CRISPR-Cas9 ribonucleoproteins for effective tissue specific genome editing. Nature Communications 11, 3232.
| Crossref | Google Scholar |
Wolter F, Schindele P, Puchta H (2019) Plant breeding at the speed of light: the power of CRISPR/Cas to generate directed genetic diversity at multiple sites. BMC Plant Biology 19, 176.
| Crossref | Google Scholar |
Woo JW, Kim J, Kwon SI, Corvalán C, Cho SW, Kim H, Kim S-G, Kim S-T, Choe S, Kim J-S (2015) DNA-free genome editing in plants with preassembled CRISPR-Cas9 ribonucleoproteins. Nature Biotechnology 33, 1162-1164.
| Crossref | Google Scholar | PubMed |
Xie K, Yang Y (2013) RNA-guided genome editing in plants using a CRISPR–Cas system. Molecular Plant 6, 1975-1983.
| Crossref | Google Scholar | PubMed |
Xie Y, Haq SIU, Jiang X, Zheng D, Feng N, Wang W, He J-S, Qiu Q-S (2022) Plant genome editing: CRISPR, base editing, prime editing, and beyond. Grassland Research 1, 234-243.
| Crossref | Google Scholar |
Xu Z, Xu X, Gong Q, Li Z, Li Y, Wang S, Yang Y, Ma W, Liu L, Zhu B, Zou L, Chen G (2019) Engineering broad-spectrum bacterial blight resistance by simultaneously disrupting variable TALE-binding elements of multiple susceptibility genes in rice. Molecular Plant 12, 1434-1446.
| Crossref | Google Scholar | PubMed |
Yang T, Zhang Y, Guo L, Li D, Liu A, Bilal M, Xie C, Yang R, Gu Z, Jiang D, Wang P (2024) Antifreeze polysaccharides from wheat bran: the structural characterization and antifreeze mechanism. Biomacromolecules 25, 3877-3892.
| Crossref | Google Scholar |
Yao X, Xie R, Zan X, Su Y, Xu P, Liu W (2023) A novel image encryption scheme for DNA storage systems based on DNA hybridization and gene mutation. Interdisciplinary Sciences: Computational Life Sciences 15, 419-432.
| Crossref | Google Scholar | PubMed |
Yin X, Biswal AK, Dionora J, Perdigon KM, Balahadia CP, Mazumdar S, Chater C, Lin H-C, Coe RA, Kretzschmar T, Gray JE, Quick PW, Bandyopadhyay A (2017) CRISPR-Cas9 and CRISPR-Cpf1 mediated targeting of a stomatal developmental gene EPFL9 in rice. Plant Cell Reports 36, 745-757.
| Crossref | Google Scholar | PubMed |
Yip BH (2020) Recent advances in CRISPR/Cas9 delivery strategies. Biomolecules 10, 839.
| Crossref | Google Scholar | PubMed |
Yuan B, Zhang S, Song L, Chen J, Cao J, Qiu J, Qiu Z, Chen J, Zhao X-M, Cheng T-L (2023) Engineering of cytosine base editors with DNA damage minimization and editing scope diversification. Nucleic Acids Research 51, e105.
| Google Scholar |
Zahra N, Hafeez MB, Ghaffar A, Kausar A, Zeidi MA, Siddique KHM, Farooq M (2023) Plant photosynthesis under heat stress: effects and management. Environmental and Experimental Botany 206, 105178.
| Crossref | Google Scholar |
Zaidi SS-e-A, Mukhtar MS, Mansoor S (2018) Genome editing: targeting susceptibility genes for plant disease resistance. Trends in Biotechnology 36, 898-906.
| Crossref | Google Scholar |
Zeng D-D, Yang C-C, Qin R, Alamin M, Yue E-K, Jin X-L, Shi C-H (2018) A guanine insert in OsBBS1 leads to early leaf senescence and salt stress sensitivity in rice (Oryza sativa L.). Plant Cell Reports 37, 933-946.
| Crossref | Google Scholar | PubMed |
Zeng Y, Wen J, Zhao W, Wang Q, Huang W (2020) rational improvement of rice yield and cold tolerance by editing the three genes OsPIN5b, GS3, and OsMYB30 with the CRISPR–Cas9 system. Frontiers in Plant Science 10, 1663.
| Crossref | Google Scholar |
Zhang Y, Bai Y, Wu G, Zou S, Chen Y, Gao C, Tang D (2017) Simultaneous modification of three homoeologs of TaEDR1 by genome editing enhances powdery mildew resistance in wheat. The Plant Journal 91, 714-724.
| Crossref | Google Scholar | PubMed |
Zhang A, Liu Y, Wang F, Li T, Chen Z, Kong D, Bi J, Zhang F, Luo X, Wang J, Tang J, Yu X, Liu G, Luo L (2019a) Enhanced rice salinity tolerance via CRISPR/Cas9-targeted mutagenesis of the OsRR22 gene. Molecular Breeding 39, 47.
| Crossref | Google Scholar |
Zhang T, Zhao Y, Ye J, Cao X, Xu C, Chen B, An H, Jiao Y, Zhang F, Yang X, Zhou G (2019b) Establishing CRISPR/Cas13a immune system conferring RNA virus resistance in both dicot and monocot plants. Plant Biotechnology Journal 17, 1185-1187.
| Crossref | Google Scholar | PubMed |
Zhang R, Liu J, Chai Z, Chen S, Bai Y, Zong Y, Chen K, Li J, Jiang L, Gao C (2019c) Generation of herbicide tolerance traits and a new selectable marker in wheat using base editing. Nature Plants 5, 480-485.
| Crossref | Google Scholar | PubMed |
Zhang D, Hussain A, Manghwar H, Xie K, Xie S, Zhao S, Larkin RM, Qing P, Jin S, Ding F (2020) Genome editing with the CRISPR-Cas system: an art, ethics and global regulatory perspective. Plant Biotechnology Journal 18, 1651-1669.
| Crossref | Google Scholar | PubMed |
Zhang Y, Iaffaldano B, Qi Y (2021) CRISPR ribonucleoprotein-mediated genetic engineering in plants. Plant Communications 2, 100168.
| Crossref | Google Scholar | PubMed |
Zhang Y, Xu J, Li R, Ge Y, Li Y, Li R (2023) Plants’ response to abiotic stress: mechanisms and strategies. International Journal of Molecular Sciences 24, 10915.
| Crossref | Google Scholar | PubMed |
Zhao S, Zhang Q, Liu M, Zhou H, Ma C, Wang P (2021) Regulation of plant responses to salt stress. International Journal of Molecular Sciences 22, 4609.
| Crossref | Google Scholar | PubMed |
Zhao Z, Shang P, Mohanraju P, Geijsen N (2023) Prime editing: advances and therapeutic applications. Trends in Biotechnology 41, 1000-1012.
| Crossref | Google Scholar |
Zheng N, Li L, Wang X (2020) Molecular mechanisms, off-target activities, and clinical potentials of genome editing systems. Clinical and Translational Medicine 10, 412-426.
| Crossref | Google Scholar | PubMed |
Zhou J, Peng Z, Long J, Sosso D, Liu B, Eom J-S, Huang S, Liu S, Vera Cruz C, Frommer WB, White FF, Yang B (2015) Gene targeting by the TAL effector PthXo2 reveals cryptic resistance gene for bacterial blight of rice. The Plant Journal 82, 632-643.
| Crossref | Google Scholar | PubMed |
Zhou X, Liao H, Chern M, Yin J, Chen Y, Wang J, Zhu X, Chen Z, Yuan C, Zhao W, Wang J, Li W, He M, Ma B, Wang J, Qin P, Chen W, Wang Y, Liu J, Qian Y, Wang W, Wu X, Li P, Zhu L, Li S, Ronald PC, Chen X (2018) Loss of function of a rice TPR-domain RNA-binding protein confers broad-spectrum disease resistance. Proceedings of the National Academy of Sciences 115, 3174-3179.
| Crossref | Google Scholar |
Zhou Y, Xu S, Jiang N, Zhao X, Bai Z, Liu J, Yao W, Tang Q, Xiao G, Lv C, Wang K, Hu X, Tan J, Yang Y (2022) Engineering of rice varieties with enhanced resistances to both blast and bacterial blight diseases via CRISPR/Cas9. Plant Biotechnology Journal 20, 876-885.
| Crossref | Google Scholar | PubMed |
Zhu C, Bortesi L, Baysal C, Twyman RM, Fischer R, Capell T, Schillberg S, Christou P (2017) Characteristics of genome editing mutations in cereal crops. Trends in Plant Science 22, 38-52.
| Crossref | Google Scholar | PubMed |
Zia R, Nawaz MS, Siddique MJ, Hakim S, Imran A (2021) Plant survival under drought stress: implications, adaptive responses, and integrated rhizosphere management strategy for stress mitigation. Microbiological Research 242, 126626.
| Crossref | Google Scholar | PubMed |