The role of topography on the local circulation and formation of fog at Perth Airport
Belinda Roux

A
B
C
Abstract
Perth Airport is located on a coastal plain in the south-west of Australia, with the Indian Ocean to the west and the Darling Scarp running approximately parallel to the coast to the east. On average, there are approximately nine fog events per year at the airport, typically occurring during the cooler months in the early morning hours. Onshore winds bringing moisture from the Indian Ocean can combine with nocturnal cooling in stable atmospheres to encourage fog formation. A previous climatological study of fog at Perth Airport found that the majority of events had north to north-easterly 10-m winds at fog onset time. Two case studies are presented to gain a better understanding of the physical processes associated with the north to north-easterly near-surface flow and their influence on the development of fog. The hypothesis is that the escarpment is blocking the moist environmental flow, resulting in light northerly near-surface winds. This was tested through numerical experiments including altered terrain. The main finding from the case studies was that the northerly winds stem from a blocking of the airmass in the lower level of the atmosphere by the Darling Scarp in moderate wind situations. During calm or very light wind occasions, the winds below the surface inversion level can tend northerly regardless of topography. The trapped airmass and light winds in the near surface layer in combination with nocturnal surface cooling and moisture from the environmental flow, create conditions favourable for the development of fog at Perth Airport.
Keywords: aviation, blocked flow, Darling Scarp, fog dynamics, local circulation, near-surface winds, nocturnal cooling, orographic forcing, Perth Airport.
1.Introduction
Perth, Western Australia (WA), is one of the most remote major cities in the world for aviation, with the closest viable alternative airport for large aircraft located ~3 h of flying time away. Given that aircraft are required to carry extra fuel if fog is forecast at the destination airport, the occurrence and forecasting of fog at Perth Airport remains an important economic and safety issue, even though Perth Airport only receives an average of nine fog events per year (Roux et al. 2021). Perth is situated on a coastal plain on the west coast of Australia, with the Indian Ocean to the west and the Darling Scarp ~30 km inland, which rises sharply to ~300 m and runs parallel to the coast for several hundred kilometres. Fig. 1 shows the topography and the locations of some automatic weather stations (AWS) around Perth, with Perth Airport denoted by YPPH.
Topography (heights shaded, m) and location of some AWS around Perth. Identified locations are Perth Airport (YPPH), Pearce Airport (YPEA), Jandakot Airport (YPJT), Swanbourne (SWAN) and Rottnest Island (YRTI). ASL, above sea level.
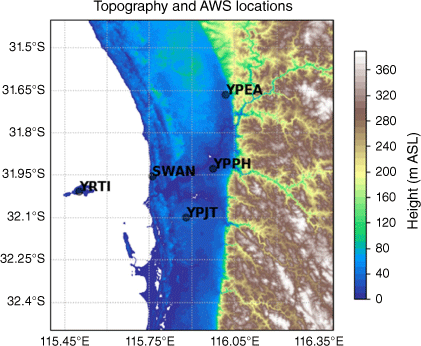
In the warm season months (November–March) the region gets little to no fog, and often experiences hot, dry easterly winds with south-westerly sea breezes in the afternoon (Roux et al. 2021) due to the prevalence of subtropical high-pressure systems to the south of the country, generally moving eastwards. In favourable conditions, when there is a synoptic easterly flow, the presence of the Darling Scarp can induce wind storms with hydraulic jumps and lee waves over the escarpment and the coastal plain, mainly in the early morning (Pitts and Lyons 1989, 1990; Blockley and Lyons 1994). The cooler months, April–October, are characterised by subtropical high-pressure systems moving across the country around Perth latitudes that are separated by cold fronts, and associated cut-off lows, that travel along the south coast of Australia towards the east. These frontal systems are responsible for the majority of precipitation across the Perth region (Wright 1974; Hope et al. 2006; Roux et al. 2021). This is also the main season for the occurrence of fog in Perth. Another common feature of the weather in the Perth area is the West Coast Trough, which is most prevalent and stronger in the summer months but can occur at other times. In the summer months, high temperatures result in a semi-permanent surface heat low over the north-west of Australia with a heat trough extending down the west coast between successive high-pressure systems. The trough normally moves eastward due to synoptic factors but may stay offshore or oscillate across the WA coast due to interactions between the diurnal variation in the surface temperature, the sea–land breeze circulation and the topography (Yimin et al. 2001).
The distinctive geographic features surrounding Perth commonly influence the local meteorology. For example, Wright (1974) found that the showery winter rains in the region are enhanced by the friction on the air as it reaches the land and the elevated topography of the Darling Scarp. In a study on the interaction of the West Coast Trough and the sea breeze in the Perth area, Yimin and Lyons (2000) note that the Darling Scarp acts to enhance the sea breeze.
In situations of weaker synoptic forcing, such as a stable atmosphere conducive to fog formation, topographical features can play an enhanced role. Müller et al. (2010) demonstrated that complex topography can be a dominant factor in the formation and evolution of fog, with cold air flow and pooling overshadowing the more subtle processes like evapotranspiration, thereby simplifying model initialisation for fog forecasting. In a case study on the evolution of valley fog in Heber Valley, Utah, Hang et al. (2016) found that the weak thermal circulations in mountainous terrain can modulate the evolution of fog. Similarly, Cuxart et al. (2021) observed that the gravity flows generated around mountain ranges and along the valleys in the Pannonian Basin in south-east central Europe influence the nocturnal atmospheric boundary layer and the presence of fog.
Coastal fog, however, is said to be one of the most challenging types of fog due to the complex interactions of the lower atmosphere, upper ocean and land surface (O’Brien et al. 2013; Fernando et al. 2021). A major initial finding from a coastal fog field campaign in eastern Canada (Fernando et al. 2021) was that synoptic- scale weather systems alone are not good predictors of fog formation and evolution, and the details of smaller (micrometeorological and microphysical) scale features play a crucial role. Even away from coastal regions, the evaluation of field case studies from the 2007 ParisFog field experiment indicated that fog results from a delicate balance among dynamic, radiative, thermodynamic, microphysical and chemical processes (Haeffelin et al. 2013). This explains why fog remains such a challenging phenomenon to predict, as these processes are rarely all observed comprehensively.
Similar to Perth, fog on the Korean western peninsula is influenced by both the ocean to the west and the topography to the east, with the interaction between moist westerly winds from the Yellow Sea and a combined land breeze and katabatic easterly wind affecting the fog formation. Choi and Speer (2006) found that a combination of moisture-laden westerly winds and nocturnal radiational cooling of the land surface can lead to coastal advection fog in this region. Closer to Perth, Adelaide (in South Australia) also has similar features, being on a coastal plain with the St Vincent Gulf to the west and the Mount Lofty Ranges to the east. Tepper and Watson (1990) studied the wintertime nocturnal north-easterly winds in Adelaide, which have been associated with the rapid onset of low cloud, drizzle and fog. They concluded that the origin of the north-easterly wind was due to topographic blocking by the Mount Lofty ranges, rather than katabatic flow as previously believed. The Mount Lofty ranges in Adelaide are of similar height to the Darling Scarp in Perth, though the topography of the Korean western peninsula reaches much greater heights.
A comprehensive fog climatology for Perth Airport was developed by Roux et al. (2021) in an effort to better understand the occurrence of fog and improve forecasts. They showed that nearly all fog events occurred in the cool season (April–October) during the night and early morning hours. Approximately 60% of the fog events had a northerly 10-m wind component at fog onset time, even though the winds were generally light (<5 m s−1), which would make it common for winds to have a more variable direction. Notably, 41% of fog events had northerly to easterly 10-m winds at 07:00 hours Australian Western Standard Time (AWST) (23:00 hours Coordinated Universal Time, UTC), typically near the time of fog, while the 925-hPa winds were southerly to westerly. For comparison, such winds were recorded on only 15% of all cool season days in the same period (Roux et al. 2021). This observation points to more favourable conditions for fog when the moist onshore flow and the surface flow decouple as nocturnal cooling on the coastal plain leads to increased stability in the near-surface layer. This decoupling of the surface flow from the synoptic flow is consistent with the results presented by Golding (1993), although they concluded that the surface flow results from cold air drainage from the escarpment, largely based on two-dimensional (2-D) model analyses of a single event. Roux et al. (2021) analysed 161 fog events over an 18-year period and showed the surface flow is more northerly than might be expected from a pure drainage flow, which suggests this may be a result of topographic blocking.
Roux et al. (2021) used an objective algorithm to classify the fog events at Perth Airport into three fog types: radiation fog, advection fog and cloud base lowering fog. They found that radiation fog was the most prevalent, and more than half of the radiation fog events had rainfall in the 6 h prior to fog onset time. Roux et al. (2021) examined the rainfall prior to fog onset in their climatology and found that precipitation was not a main mechanism of formation but could be important to precondition the environment. They also identified five weather regimes using a k-means clustering method and found that the majority of the fog events were observed following the passage of a post frontal system or a surface trough aligned along the coast, with an anti-cyclone to the west supplying moisture from the Indian Ocean.
An outstanding question from the work of Roux et al. (2021) is a better understanding of the physical processes associated with the development of the north to north-easterly near-surface winds and their effect on the development of fog. In this study we present two case studies to provide more insight into this question, extending the work of Golding (1993) with high resolution three-dimensional (3-D) modelling studies.
Firstly, a long lasting and spatially coherent fog event was selected, which showed a consistent evolution of northerly near-surface winds during the time of fog formation and exhibited a good fit between the model reanalysis and the surface and upper air observations. The second case is one of the most frequent fog types and class from the Roux et al. (2021) climatology, a radiation fog with preceding rain. A modified terrain experiment was also performed to ascertain the influence of topography on the near surface winds.
The current work focuses on the dynamical aspect of the northerly to north-easterly near-surface winds that are often observed during fog events at Perth Airport, and it is acknowledged that fog is a complex phenomenon and multiple processes are in play during the formation and lifecycle of fog.
2. Data and methods
We employ a variety of in situ and remote sensing observations available for Perth Airport (~20 km from the coast) and surrounding areas to help define both the fog event and the local meteorology. To discuss the synoptic and local meteorology, the Bureau of Meteorology’s mean sea level pressure (MSLP) analyses, upper air soundings from Perth Airport and near-surface observations from AWS in the Perth area were considered. Himawari-8 (Bessho et al. 2016) visible and infrared satellite data together with the derived night-microphysics product were also used. Himawari-8 is a new generation geostationary satellite operated by the Japan Meteorological Agency that became operational in July 2015. The satellite completes full-disk scans every 10 min across 16 spectral bands. The spatial resolution of the Himawari-8 data is 0.5–1 km for the visible bands and 1–2 km for near-infrared and infrared bands. The Himawari-8 satellite data were obtained from the Bureau of Meteorology Satellite Observations Collection, hosted by the National Computational Infrastructure (NCI) in Australia. The night-microphysics product takes the brightness temperature difference between the 10.8- and 3.9-µm channels (Lensky and Rosenfeld 2008) to highlight clouds with small droplets such as fog and low stratus. Since visibility is only recorded at the three airport weather stations in the area (YPEA, YPPH and YPJT) (Fig. 1), the night-microphysics product is particularly useful for monitoring the spatial patterns of fog and low cloud. One limitation of satellite products is that overlying cloud can obscure low level cloud and fog.
For the 3-D analysis of the case studies in this paper we used downscaled reanalysis from the Bureau of Meteorology Atmospheric high-resolution Regional Reanalysis for Australia (BARRA) over the Perth domain, BARRA-PH (Su et al. 2021). This 1.5-km resolution reanalysis was dynamically downscaled from BARRA-R, a 12-km resolution atmospheric reanalysis covering Australia, New Zealand and South-east Asia which has been developed by the Australian Bureau of Meteorology (Su et al. 2019). BARRA-R is produced by running the Unified Model (UM, Davies et al. 2005) regional atmospheric model with ERA-Interim (Dee et al. 2011) boundary conditions and four-dimensional (4-D) data assimilation of observations. BARRA-PH was produced by downscaling the 12-km BARRA-R reanalysis with the UM (Su et al. 2021), using a science configuration based on the first Regional Atmosphere and Land (RAL1) science configuration (Bush et al. 2019). The high-resolution reanalysis showed improved skill for the near-surface temperature and winds compared with the BARRA-R reanalysis, other global reanalyses and with point observations, particularly in coastal and topographically complex regions (Su et al. 2021).
To study the effect of the topography on the wind field, a separate modelling experiment was performed using a regional nesting suite of the UM developed by the United Kingdom Meteorological Office (UKMO). The UM was run in forecast mode, using the RAL3 science configuration, which builds on the RAL2 configuration described by Bush et al. (2023). Coupled to the JULES land surface model (Best et al. 2011; Clark et al. 2011), this configuration uses a bimodal cloud scheme (Van Weverberg et al. 2021a, 2021b), the CASIM double moment microphysics scheme (Cloud AeroSol Interaction Microphysics; Field et al. 2023), SOCRATES (suite of community radiative transfer codes based on Edwards and Slingo) radiative transfer scheme (Edwards and Slingo 1996) and a blended boundary layer parameterisation (Boutle et al. 2014; Bush et al. 2023). The model was run at a 1.5-km horizontal grid length and 90 vertical levels up to a height of 40 km, with 12-levels in the lowest 500 m. The model was initialised at 00:00 hours UTC on the morning prior to the fog event (giving a lead time of more than 12 h before fog formation for both cases), using the 12-km BARRA-R reanalysis as initial and boundary conditions and it was run out to 36 h. The model was run over a 500 × 500 grid (750 × 750 km) centred on Perth Airport, with standard topography for the control simulation and a ‘flat terrain’ experiment where a uniform height of 1 m was set for land areas across the domain. It should be noted that for this experiment there were differences in the ancillary data characterising the land surface from those used for the BARRA-PH analyses and this led to small differences in the forecast near-surface temperatures.
The UM includes visibility and fog fraction diagnostics that use values of the forecast meteorological variables pressure, temperature, specific humidity and cloud liquid water at screen level height. It is also dependent on the aerosol mixing ratio plus several fixed parameters. The visibility scheme operates by hydrating the provided aerosol field so that it is in equilibrium with the atmospheric humidity, and the visibility is then computed based on the fog droplet size and number density (Clark et al. 2008; Wright 1997a, 1997b; B. M. Claxton, unpubl. data). The fog fraction represents the fraction of the grid box having a visibility below 1000 m, with the diagnosis procedure considering the distribution of states over the grid box (Wright 1997a).
The Australian Community Climate and Earth System Simulator (ACCESS) numerical weather prediction (NWP) model operated by the Australian Bureau of Meteorology (Bureau of Meteorology 2010; Puri et al. 2013) is based closely on the UM developed by the UKMO. This provides routine forecasts of visibility and fog fraction across Australia based on the UM diagnostic, and previous studies have demonstrated that predictions of visibility are generally too high (Dare 2017; Roux 2017; Dare and Potts 2019a, 2019b). The inaccuracies are associated with the functioning of the visibility diagnostic across Australia, and this includes a sensitivity to small errors in the forecast meteorological fields. There is ongoing work to improve the model forecast fields and the visibility diagnostic, but this is outside the scope of this study, which is focused on a better understanding of the local circulations and physical processes that contribute to the development of fog at Perth Airport.
3.Case 1: 15 September 2017
The first case occurred during the night of 15 September 2017 and into the early morning hours of 16 September. This was a long-lasting fog event across an extended area, from the north of Geraldton (~28.5°S) to well south of Perth (~34°S), in a region along the coast to ~100 km inland. According to the Roux et al. (2021) climatology, this event was classified as an advection fog, in a weather regime (class 2) typically characterised by a high-pressure ridge extending along the south coast of WA. It is noted that in the classification of ‘advection’ fog by Roux et al. (2021), no distinction is made between fog advected from elsewhere and fog that develops when relatively warm-moist air moves over a colder surface. The evolution of fog was well observed in the Himawari-8 satellite data, with no overlying clouds present in the region of fog formation and development. The development and subsequent clearance of fog is seen in the sequence of night-microphysics and visible images shown in Fig. 2.
Himawari-8 night-microphysics images (a, b, c) followed by visible images (d, e, f), for every second hour from 17:00 hours UTC on 15 September 2017 (a) to 03:00 hours UTC on 16 September (f). The extent of the images goes from ~114–118°E, 28.5–35.5°S and the cyan coloured areas in the night-microphysics images (a, 17:00; b, 19:00; and c, 21:00 hours UTC) show the areas of fog. Approximate position of Perth Airport is denoted by the black cross.
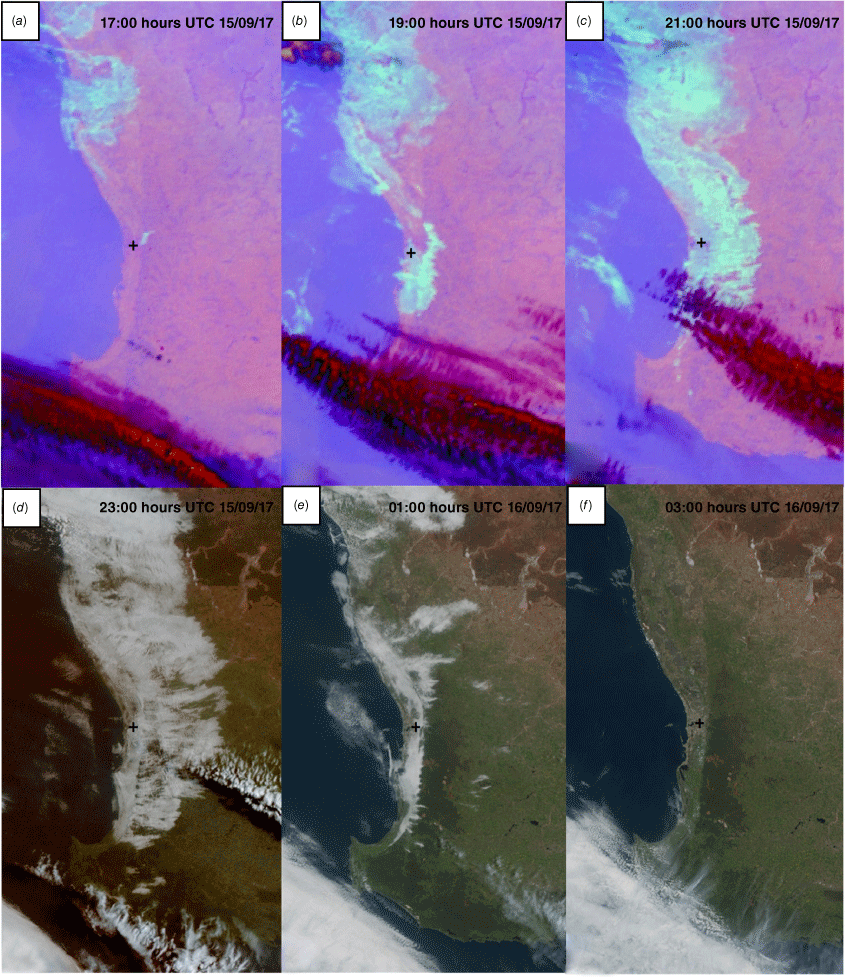
Fog first developed on the coastal plain near Perth c. 00:00 hours AWST on 16 September 2017 (16:00 hours UTC, 15 September 2017), and progressively extended across the coastal plain and adjacent interior before clearing 9–10 h later in the period 09:00–10:00 hours AWST (01:00–02:00 hours UTC). The time series of selected AWS observations around Perth (Fig. 3) for the period 13:00 hours AWST, 15 September (05:00 hours UTC, 15 September) to 14:00 hours AWST, 16 September (06:00 hours UTC, 16 September) show warm and dry east to north-east winds in the early afternoon of 15 September with a cooler and more humid south to south-west sea breeze that moves inland from the coast. That change was most evident at SWAN on the coast c. 14:00 hours AWST (06:00 hours UTC) with a sharp decrease in the temperature and increase in the dew point followed by a more gradual decrease in temperature and increase in dew point until c. 22:00 hours AWST (14:00 hours UTC) when both were ~15°C. At Perth Airport (YPPH), the south-west wind change occurred c. 16:00 hours AWST (08:00 hours UTC), 2 h later than at the coast, with a rapid fall in temperature to ~12°C and a more gradual increase in dew point temperature. By 22:00 hours AWST (14:00 hours UTC), a light northerly was established at all locations on the coastal plain. The satellite data and visibility observations showed the fog starting to develop on 16 September c. 00:00 hours AWST (16:00 hours UTC, 15 September) with the temperature and dewpoint at the airport locations gradually increasing to ~15°C. The fog dissipated in the period 09:00–10:00 hours AWST (01:00–02:00 hours UTC), well after sunrise at 06:15 hours AWST (22:15 hours UTC, 15 September). As the surface temperature rises after sunrise the fog dissipates, the wind direction shifts west to south-west and the dew point decreases.
Time-series of observations for fog event of 15 September 2017. Note that visibility was not available at Rottnest Island (YRTI) and Swanbourne (SWAN). Temperature is denoted by ‘T’; dewpoint temperature by ‘Td’; wind by ‘wnd’; visibility by ‘vis’ and precipitation by ‘prcp’ in the colour bar. Vertical orange lines show sunset (left) and sunrise (right).
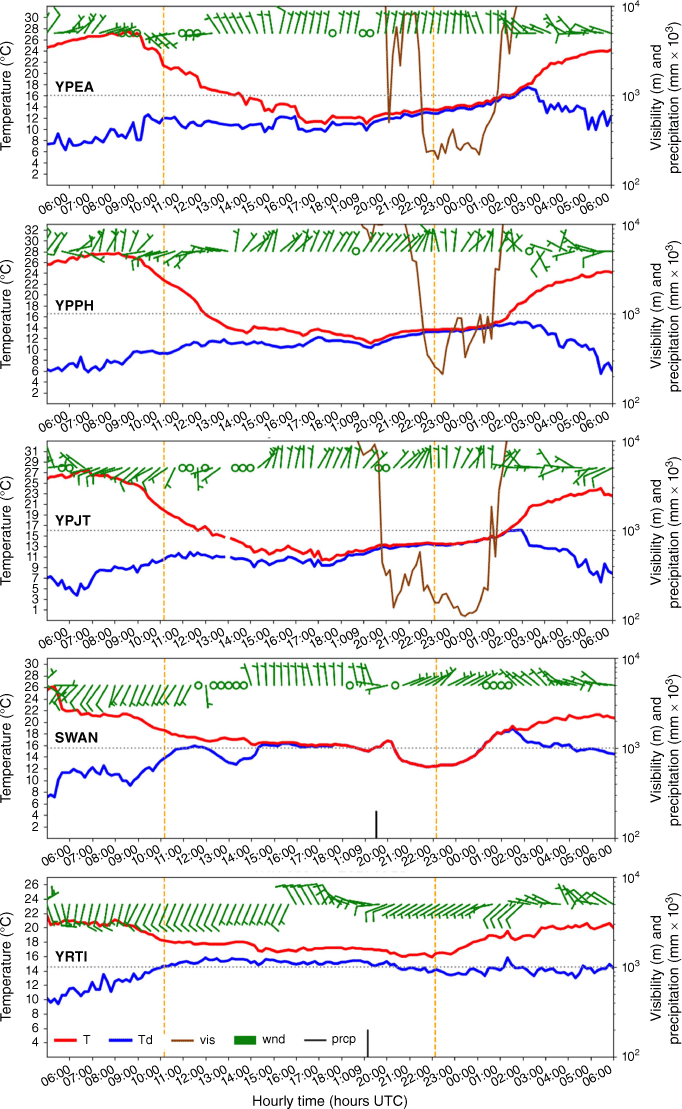
To better understand the physical processes and the origin of the airmass in which the fog at Perth Airport forms we examine the broader synoptic situation, including the upper air soundings and winds, and retrieve the back trajectories for the 24-h period prior to 05:00 hours AWST on 16 September (21:00 hours UTC, 15 September).
There was no precipitation for at least 2 days prior to the event. The MSLP analysis for 08:00 hours AWST on 15 September (00:00 hours UTC) (not presented) showed a shallow West Coast Trough over the west of WA with a weak heat-low on the coast to the north of Perth. This was directing a warm, dry and shallow north-east flow across Perth with north-west winds above 900 hPa. The sea breeze developed on the coast in the afternoon and moved inland reaching Perth Airport c. 16:00 hours AWST (08:00 hours UTC). The mean sea level pressure at Perth and Pearce Airports was a minimum at this time with a significant increase in the following 18 h. This marked the eastward movement of the West Coast Trough. By 08:00 hours AWST on 16 September (00:00 hours UTC) (Fig. 4a), there was a weak ridge lying east–west across the WA coast north of Perth, the trough was ~500 km inland from the west coast and a cold front was approaching the far south-west of WA.
MSLP analysis at 00:00 hours UTC (08:00 hours AWST) on 16 September 2017, during the time of the fog (a) and upper air soundings of the evening before fog at 11:00 hours UTC (19:00 hours AWST) on 15 September (b) and the morning during fog at 23:00 hours UTC on 15 September (07:00 hours AWST, 16 September) (c) at Perth Airport. Study area indicated with red rectangle on MSLP map (a).
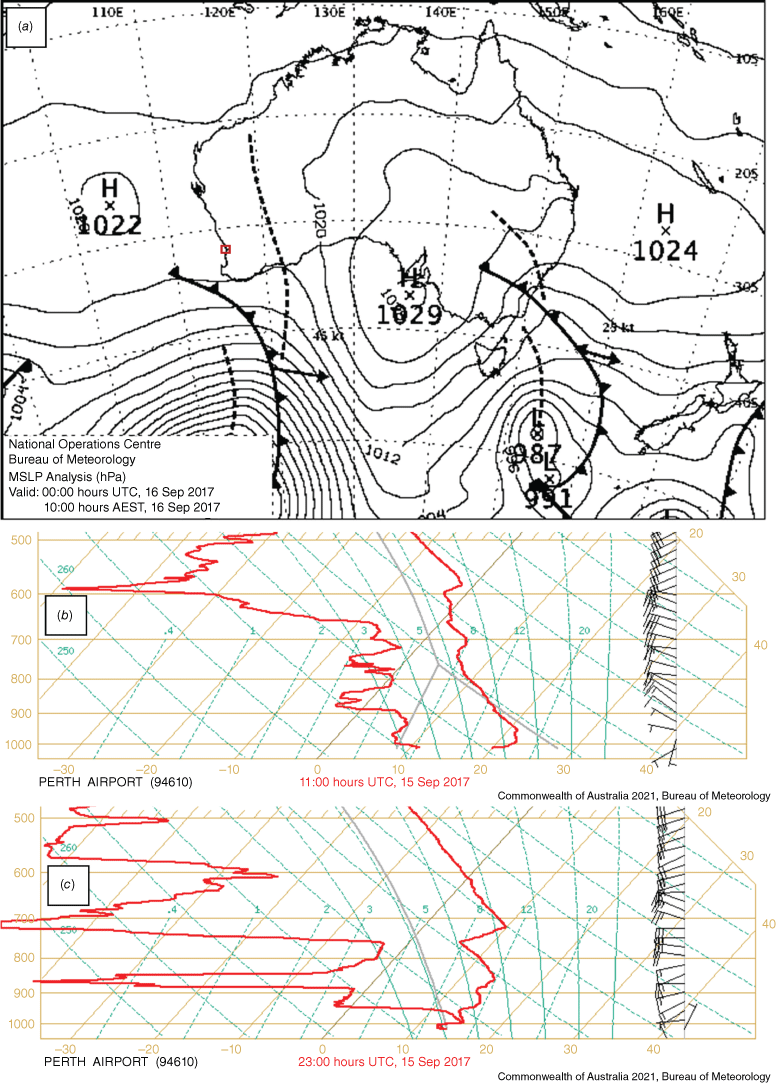
The Perth Airport upper air soundings for 19:00 hours AWST on 15 September (11:00 hours UTC) and 07:00 hours AWST on 16 September (23:00 hours UTC, 15 September) (Fig. 4b, c) show a deep westerly airflow above 950 hPa with a small shift to the south-west below 850 hPa by 23:00 hours UTC. The near-surface wind shifted from south-west at 11:00 hours UTC to north to north-east by 23:00 hours UTC. The temperature profiles show substantial warming in the 750–600-hPa layer with the development of a subsidence inversion at 750 hPa consistent with the development of the ridge across the coast. There was also substantial cooling in the layer below 850 hPa indicating an airmass change, likely associated with the West Coast Trough moving east and the wind direction shifting more south-west. The dew point profiles show drying below 600 hPa apart from a substantial increase in moisture below 950 hPa (~600 m above ground level) associated with the sea breeze moving inland. The morning sounding (Fig. 4c) shows a strong inversion above the surface level fog.
The National Oceanic and Atmospheric Administration’s Hybrid Single-Particle Lagrangian Integrated Trajectory (HYSPLIT) (Draxler and Hess 1998) back trajectory model was run using The Bureau’s high resolution (1.5 km) operational NWP model output for a 24-h period ending at 05:00 hours AWST on 16 September (21:00 hours UTC, 15 September) (Fig. 5). This shows the warm–dry near-surface air originated from ~100 km north of Perth in the early morning of 15 September (21:00 hours UTC, 14 September) when the low was present. The air moved out to sea until c. 14:00 hours AWST (06:00 hours UTC) when the sea breeze developed, and the trough moved inland. The airmass then moved back to the east and across the coast. In the period over water, the trajectory data show a stable near-surface layer with a large increase in the relative humidity at the lowest levels. After moving across the coast, the trajectory of the air at 50 m changed sharply from an easterly direction to a southerly direction c. 20:00 hours AWST (12:00 hours UTC) as it approaches the Darling Scarp. This is consistent with the moist low-level air being trapped beneath the nocturnal inversion developing on the coastal plain (evident in Fig. 4b, c) and blocked by the scarp. There are also sharp changes in the trajectory of the airmass at 150 and 350 m though less significant than at 50 m.
Back trajectories ending at Perth Airport for 24-h period from 21:00 hours UTC on 14 September (from 05:00 hours AWST, 15 September). Trajectories end at Perth Airport with elevations of 50 m (red), 150 m (blue) and 350 m (green). Markers on each of the tracks are at 00:00, 06:00, 12:00 and 18:00 hours UTC on 15 September. AGL, above ground level.
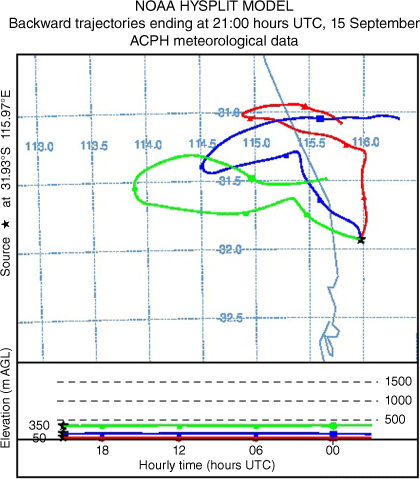
Fig. 6 shows plan views of the 10-m wind barbs from BARRA-PH (with AWS wind barbs overplotted in red) on the left and vertical east–west cross-sections through Perth Airport of the wind barbs and contours of potential temperature on the right. In the early evening on 15 September at 20:00 hours AWST (12:00 hours UTC) (Fig. 6a), the near surface winds were predominantly south to south-west over the ocean. On the coastal plain and over the plateau to the east of the escarpment the near-surface winds are generally light and variable. The vertical cross-section for the same time (Fig. 6b) shows predominantly southerly winds to the west of the airport up to a height of ~250 m, with the winds turning westerly aloft.
Plan views (left) of topography (shaded) and 10-m BARRA-PH wind barbs overplotted with red AWS wind barbs, together with east–west cross-sections through Perth Airport (right) with wind barbs, theta (contours, °C) and vertical winds (shades, m s−1) for 15 September at 12:00 hours UTC (20:00 hours AWST) (a, b), for 15 September at 22:00 hours UTC (06:00 hours AWST, 16 September) (c, d), and for 16 September at 02:00 hours UTC (10:00 hours AWST) (e, f). For the wind barbs, a half barb represents 5 kn (~2.57 m s−1), full barb represents 10 kt (~5.14 m s−1) and open red, black and blue circles are for calm winds (<5 kn, <~2.57 m s−1). Scale for vertical axis in cross sections is metres. Red transect line in (c) shows location of cross section and the closed red dot shows Perth Airport (YPPH).
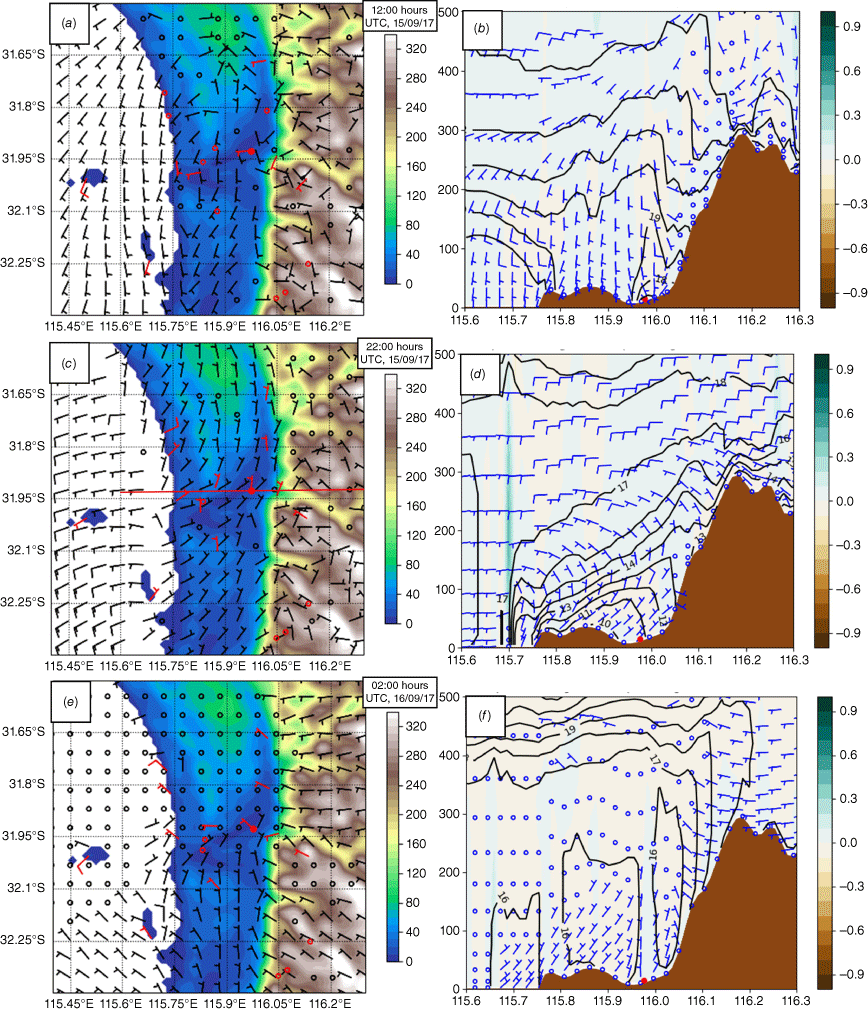
By 06:00 hours AWST on 16 September (22:00 hours UTC, 15 September) (Fig. 6c) when Perth Airport was in fog, there were west to south-westerly near-surface winds over the ocean and light north to north-easterly winds over most of the coastal plain. The winds east of the escarpment were mostly light west to north-westerlies. The vertical cross-section (Fig. 6d) shows calm winds right near the surface, with north to north-easterly winds on the coastal plain to a height ~200 m before the winds turned more westerly aloft. The contours of potential temperature show a relatively strong nocturnal inversion over the coastal plain to an altitude of ~250 m (~998 hPa). This is consistent with the vertical profiles observed in Fig. 4. The blue–green shading in the cross section indicates ascent on the coast and suggests the onshore flow is ascending over the colder airmass on the coastal plain.
With regard to temperature, BARRA-PH is also generally consistent with both the 11:00 and 23:00 hours UTC soundings, giving us confidence in the presence of the strong surface inversion on the coastal plain below 250 m shown in the cross section. In a stable boundary layer, colder and denser near-surface airflow approaching an escarpment or ridge may not be able to flow over the terrain, depending on the wind speed normal to the terrain, the stability and the height of the escarpment or ridge. In this situation, the blocked flow will be deflected by the synoptic-scale pressure gradient towards the south, in an airflow pattern akin to a coastally trapped low-level (barrier) jet (Stull 2017). A barrier jet forms when low-level winds approach a barrier and is blocked, causing the winds to turn parallel to the barrier, towards the area of lower pressure. The occurrence of blocking can be determined by the Froude number (Fr, Stull 2017) given as follows:
where U is the mean wind speed normal to the terrain, N is the Brunt–Vaisala frequency and h is the height of the escarpment. A low Fr value (Fr < 1) indicates that the flow will be blocked whereas a large Fr value (Fr > 1) indicates that the air will flow over the escarpment. In the above equation, N is given as follows:
where ϴ is the mean potential temperature through the layer and δϴ/δZ is the vertical potential temperature gradient in the stable layer. Taking the mean potential temperature and vertical temperature gradient in the layer below 250 m (Fig. 6d), N is 0.026 s−1. From the radiosonde flight on 15 September at 23:00 hours UTC, the gradient level (near the top of the escarpment) wind component normal to the escarpment was 4.4 m s−1. The height of the escarpment was 300 m, giving Fr = 0.56, meaning the cold near-surface air was unable to ascend the escarpment and the flow was blocked. The Fr value remained <1 under a range of adjacent temperatures and wind speeds, although the exact number may have varied due to spatial and temporal changes. The observed trajectory of the low-level air shown in Fig. 5 is consistent with these results.
The trapped moist airmass on the coastal plain below the nocturnal surface inversion, together with radiative cooling and weak turbulence from the light northerlies based on analysis of the turbulent kinetic energy field (not shown), provided a favourable environment for fog development. Local drainage flows (which can contribute to mixing) may have also be present around variations in the local topography along the escarpment but were not strong or persistent enough to be evident in the AWS observations or obvious in the reanalysis. With the limited height of the escarpment, any drainage flow would be expected to be weak and shallow, compared to drainage flows in more formidable mountain valleys. Tepper and Watson (1990) used katabatic models from Nappo and Rao (1987) and Manins and Sawford (1979) to show that katabatic flow over similar slopes and heights as for the Darling Scarp would be weak (<2 m s−1) and shallow (~10 m deep).
At 10:00 hours AWST on 16 September (02:00 hours UTC) (Fig. 6e, f), near the time of fog clearance, the wind increased east of the escarpment with a more consistent westerly component. Over the ocean and on the coastal plain the winds became light and variable. The northern half of the domain became stagnant at this time, whereas the southern half still had the northerlies on the coastal plain. Overall, there was good agreement between the observations and model reanalysis, which gives us confidence that the reanalysis is capturing the local circulations well.
To further investigate the role of the escarpment on the local circulations, additional forecast model simulations, with a control and a flat terrain experiment were performed, as discussed in Section 2. This model run was initialised at 00:00 hours UTC on 15 September with the 12-km BARRA-R reanalysis as initial and boundary conditions. As a first step in this experiment, the time series of surface observations were compared with corresponding forecast model fields for the period 00:00 hours UTC on 15 September to 06:00 hours UTC on 16 September. As previously discussed in relation to Fig. 3, observations at SWAN, on the coast (Fig. 7a), initially showed warm and dry easterly winds shifting south-west c. 05:00–06:00 hours UTC on 15 September with the development of the sea breeze. This was associated with a sharp decrease in the temperature and increase in the dew point followed by a more gradual decrease in temperature and increase in dew point until c. 14:00 hours UTC on 15 September when both were ~15°C. By this time, the wind at SWAN had shifted to a northerly direction. The air then remained saturated with some variation in the temperature until c. 02:00 hours UTC on 16 September.
Time series of AWS observations of wind, temperature (°C) and dew point (°C) (dashed lines, dark green barbs) and corresponding forecast model fields (solid lines, light green barbs) at Swanbourne AWS (a) and Perth Airport (b) for period 00:00 hours UTC, 15 September to 06:00 hours UTC, 16 September 2017. The forecast model base time was 00:00 hours UTC, 15 September 2017. For the wind barbs, a half barb represents 5 kn (~2.57 m s−1), full barb represents 10 kt (~5.14 m s−1) and open circle is calm (<5 kn, <~2.57 m s−1).
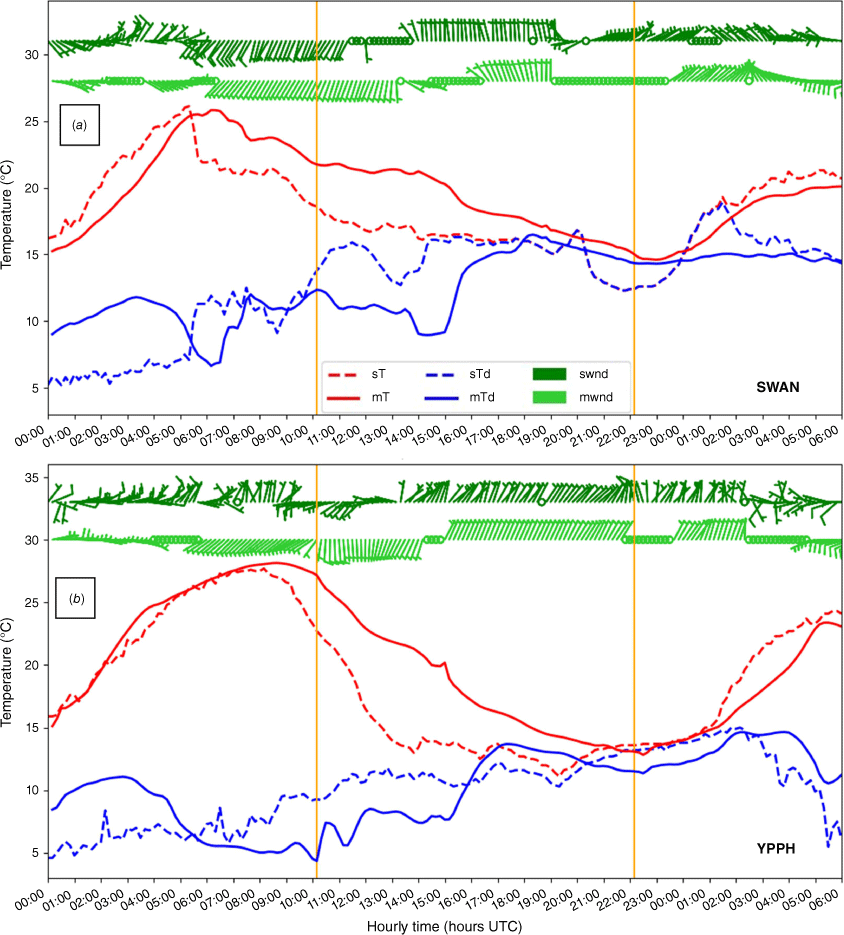
Differences are evident in the corresponding forecast model fields with the initial high dew point temperature indicating that there may have been some error in the initial conditions, the model air temperature biased high by 3–5°C in the evening, the model dew point biased low by 2–4°C in the evening and differences in the timing for wind changes. For Perth Airport, Fig. 7b shows differences in the forecast model fields similar to those for SWAN with the high initial value for the dew point, the model air temperature biased high in the evening, the model dewpoint biased low in the evening and differences in the timing for wind changes. There is also a 2°C difference between the temperature and dewpoint in the period when fog was present at Perth Airport. There are several factors that contribute to these differences but the changes in the temperatures and wind are mostly captured. These provide reasonable confidence in results from the forecast model simulations.
Fig. 8a, b shows the 10-m wind field and the east–west vertical cross section from the control simulation and valid for 22:00 hours UTC on 15 September. Comparing the control experiment (Fig. 8a, b) with the BARRA-R reanalyses for the same time (Fig. 6c, d) shows good agreement in the plan-view wind field and the cross section of wind and potential temperature. There are only small differences in the temperature and wind fields, with the near-surface temperature of the experiment slightly warmer and the temperature inversion over the coastal plain in the reanalysis cross-section a little stronger. However, the control experiment still simulates the blocked flow, with a Fr value of ~0.7 along the latitude of Perth Airport.
Plan views (left) of topography (shaded) and 10-m wind barbs, with east–west cross-sections along Perth Airport latitude (right) with wind barbs, vertical winds (shades, m s−1) and theta contours (°C) for the control (a, b) and flat terrain (c, d) experiment for 15 September at 22:00 hours UTC (06:00 hours AWST, 16 September). For the wind barbs, a half barb represents 5 kn (~2.57 m s−1), full barb represents 10 kt (~5.14 m s−1) and open red, black and blue circles are for calm winds (<5 kn, <~2.57 m s−1). The closed red dot shows Perth Airport (YPPH).
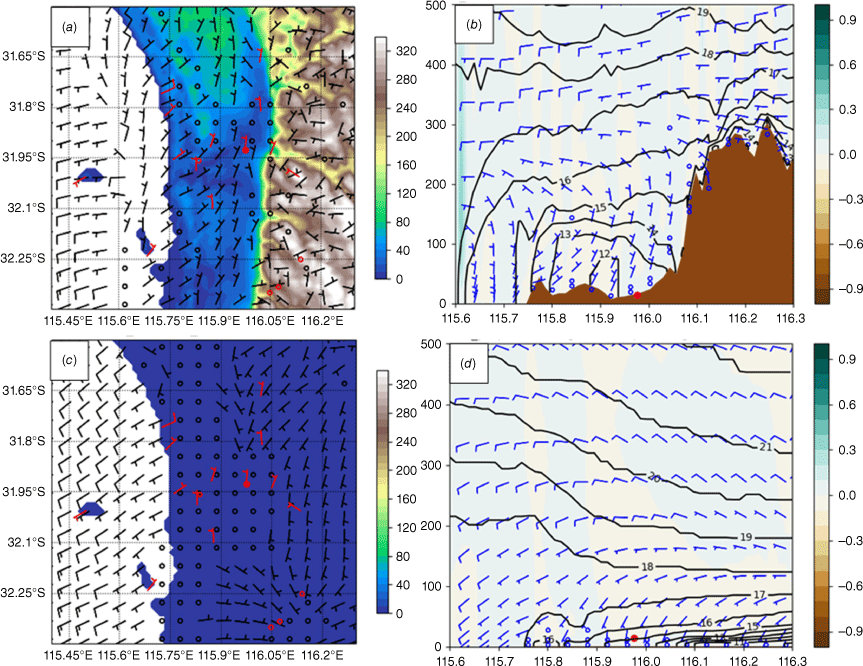
The corresponding output from the flat terrain experiment (Fig. 8c, d), with an imposed terrain elevation of 1 m, shows significant differences from the control run. The low-level plan view shows that the south-west winds extend across the domain with very little change as the airstream moves over land. The vertical cross-section also shows the south-west winds at lower altitudes across the coastal region where observations and the control run showed northerly winds. A strong surface inversion is evident inland from Perth Airport. This provides good evidence that the Darling Scarp causes the development of blocked flow on the coastal plain. At elevations above 200 m, the westerly onshore airflow is unperturbed by the terrain and winds shift more north-west inland with much warmer temperatures evident than in the control run.
Both the BARRA-PH reanalysis (Fig. 9a) and the control experiment (Fig. 9b) simulated an extensive area with a higher fog fraction in the Perth region. The BARRA-PH reanalysis has a more extensive and higher fog fraction due to differences in the simulations as mentioned previously. The timing and location of the simulated fog was not a perfect match compared with the satellite imagery (with the simulated fog in the area initiating and clearing a couple of hours earlier than observed) but the timing of the most extensive fog in the area was simulated well. The flat terrain experiment (Fig. 9c) did not simulate fog over Perth, although some fog was simulated to the north and south of the city. With the absence of the northerly near-surface flow in the flat terrain experiment, the fog or moisture to the north of the city did not get advected to the south. The flat terrain experiment did simulate fog to the south of the city, which in turn was not simulated by the BARRA-PH and control experiments.
4.Case 2: 4 July 2015
The second fog event in this study occurred during the night of 4 July 2015. This case differs from Case 1 in that there was a moist westerly air stream during the day on 4 July and into the evening with scattered to broken clouds and some showers before the fog developed. This is reasonably common, with the Roux et al. (2021) climatology showing 43% of fog events have precipitation in the 6 h prior to the fog formation. This case was classified as a radiation fog, which is the most common fog type in the Roux et al. (2021) classification, where 61% of events were classified as radiation fog.
Fog formed in the early morning at 02:30 hours AWST on 5 July (18:30 hours UTC, 4 July) at Perth Airport and cleared c. 08:45 hours AWST (00:45 hours UTC). This was the first (and most severe) of three consecutive fog event days at Perth Airport. The time series of AWS observations are given in Fig. 10. Visibility observations were only available at Pearce (YPEA) and Perth (YPPH) airports for this case. Both airports showed periods of reduced visibility earlier due to precipitation. In particular, it is apparent that showers moved across SWAN c. 14:00 hours AWST on 4 July (06:00 hours UTC) and eastward across Perth Airport c. 16:00 hours AWST (08:00 hours UTC). This was associated with a temperature decrease and increased dew point that persisted through the night. Pearce first reported low visibilities due to fog c. 01:30 hours AWST on 5 July (17:30 hours UTC, 4 July), with the airport being in and out of fog until 07:40 hours AWST (23:40 hours UTC), while the visibility was consistently low during the foggy period at Perth Airport. Winds were generally calm or light northerlies (ranging between 0 and 3.1 m s−1) on the coastal plain overnight at Perth and Jandakot Airports. Winds at Rottnest Island (YRTI) tended south-westerly for most of the night. Temperatures gradually decreased during the night for the stations on the coastal plain, with the dew point depression approaching zero in the early morning hours. After sunrise at 07:17 hours AWST on 5 July (23:17 hours UTC, 4 July), the temperature and dew point increased until the nocturnal inversion weakened, the fog dissipated and the dewpoint depression rapidly increased.
Time series of AWS observations for fog event of 4 July 2015. Note that visibility observations were not available at Rottnest Island (YRTI) and Swanbourne (SWAN) and Jandakot (YPJT). Note the visibility is presented on a log scale centred at 1 km. For the wind barbs, a half barb represents 5 kn (~2.57 m s−1), full barb represents 10 kt (~5.14 m s−1) and open circle is calm (<5 kn, <~2.57 m s−1).
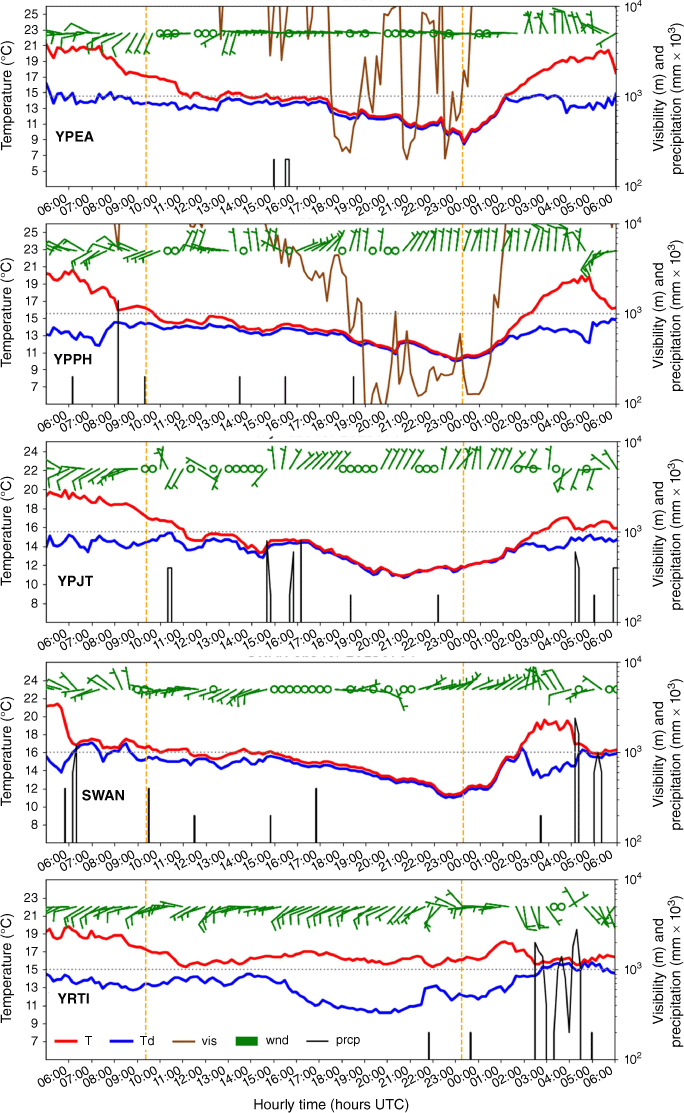
The presence of fog and low cloud was largely obscured from the satellite by overlying cloud, but there are indications from the Himawari-8 satellite images (Fig. 11) that fog was present on the coastal plain as well as over inland parts of south-west WA.
Himawari-8 night-microphysics and visible images from (a) 16:00 hours UTC and (b) 19:00 hours UTC on 4 July to (c) 01:00 hours UTC on 5 July (00:00–09:00 hours AWST, 5 July). The cyan coloured areas in the night-microphysics images (16:00 and 19:00 hours UTC) show the areas of fog. Black cross shows approximate location of Perth Airport.
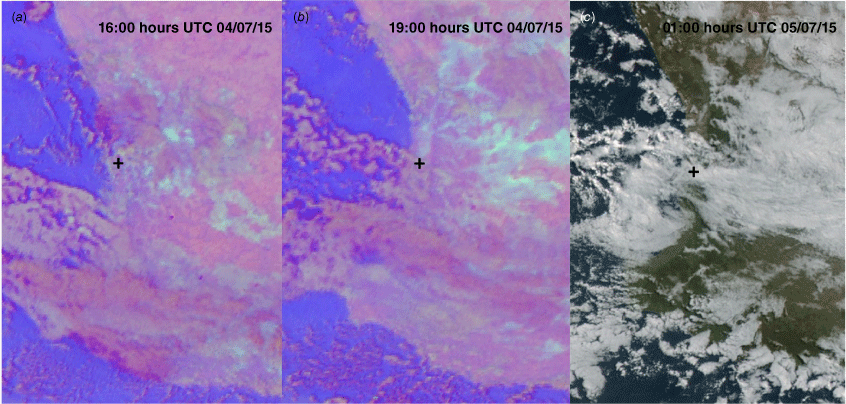
The MSLP analysis for 12:00 hours UTC on 4 July (20:00 hours AWST) (Fig. 12) showed a weak ridge lying across the west coast of WA to the north of Perth, and this was directing a moist westerly air stream over Perth with scattered to broken cloud and intermittent showers. A cold front approaching the south-west of WA at this time passed along the south coast of WA in the following 6–12 h. The early evening sounding at Perth Airport at 19:00 hours AWST (11:00 hours UTC) (Fig. 12b) showed westerly winds throughout the troposphere, except for the lowest level winds below 200 m, which were light northerly. In the early morning, 07:00 hours AWST on 5 July (23:00 hours UTC, 4 July), during the time of fog, the lowest level winds at the airport were still light northerly, with the winds above west to south-westerly and light up to the 700-hPa pressure level (Fig. 12c). The temperature and dewpoint profiles show a strong temperature inversion at ~790 hPa at 19:00 hours AWST on 4 July (11:00 hours UTC), with a very moist, cloudy layer below and dry air above, as well as a surface inversion. By 07:00 hours AWST on 5 July (23:00 hours UTC, 4 July), the air below 790 hPa had dried out while the nocturnal surface inversion had strengthened with a moist, foggy layer up to the 1000-hPa pressure level (180 m above ground level).
MSLP analysis at 12:00 hours UTC on 4 July 2015 (20:00 hours AWST) (a) and Perth Airport upper air soundings on 4 July at 11:00 hours UTC (19:00 hours AWST) (b) and at 23:00 hours UTC (07:00 hours AWST, 5 July) (c).
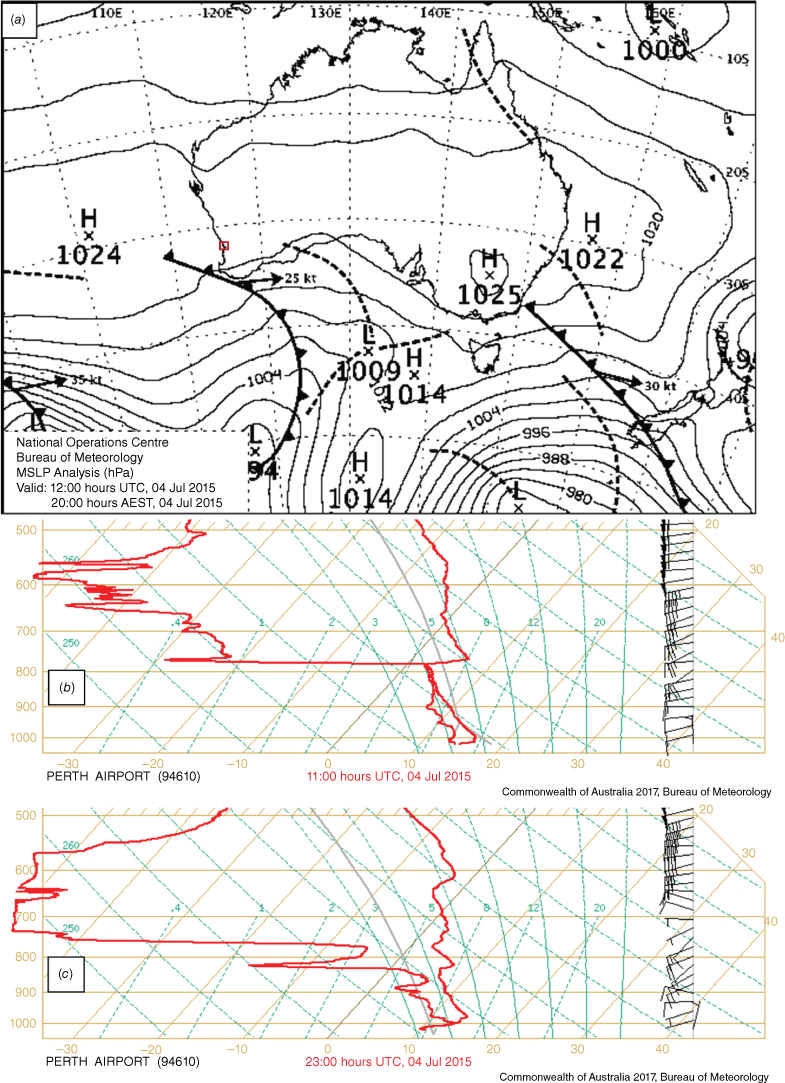
Plan views of the 10-m wind across the area as well as cross sections of potential temperature and winds at the latitude of Perth Airport from the BARRA-PH reanalysis show the structure and evolution of these variables overnight and into the morning (Fig. 13). It is noted that the observed near-surface winds were light and variable throughout the event, consistent with the fog type being radiation fog according to Roux et al. (2021). At 11:00 hours UTC on 4 July (19:00 hours AWST) (Fig. 13a, b), the winds were generally westerly across the area. Perth Airport reported northerly 10-m winds of 2.6 m s−1 at this time. By 20:00 hours UTC (04:00 hours AWST, 5 July) (Fig. 13c, d), the winds over the ocean were still westerly, while the winds on the coastal plain tended north to north-easterly. The cross-section shows a temperature inversion with northerly winds on the coastal plain up to a height of ~300 m, the height of the escarpment. The calculated Fr value at Perth Airport at this time was 0.64, indicating that the low-level onshore westerly winds are not able to flow over the Darling Scarp. The airflow is blocked and is diverted south towards lower pressures similar to the previous case. At 01:00 hours UTC on 5 July (09:00 hours AWST) (Fig. 13e, f), near the time of fog clearance, the winds on the coastal plain were still northerly. Fig. 13f also shows that the surface temperature at Perth was warming and the lapse rate in the lowest levels was more neutral.
Plan views (left) of topography (shaded) and 10-m BARRA-PH analysis wind barbs overplotted with observed AWS wind barbs (red), together with east–west cross-sections through Perth Airport (right) with wind barbs, theta contours (°C) and vertical winds (shades, m s−1) for 11:00 hours UTC on 4 July (19:00 hours AWST) (a, b), 20:00 hours UTC (04:00 hours AWST, 5 July) (c, d) and 01:00 hours UTC on 5 July (09:00 hours AWST) (e, f). For the wind barbs, a half barb represents 5 kn (~2.57 m s−1), full barb represents 10 kt (~5.14 m s−1) and open red, black and blue circles are for calm winds (<5 kn, <~2.57 m s−1). Scale for vertical axis in cross-sections is in metres. The closed red dot denotes Perth Airport.
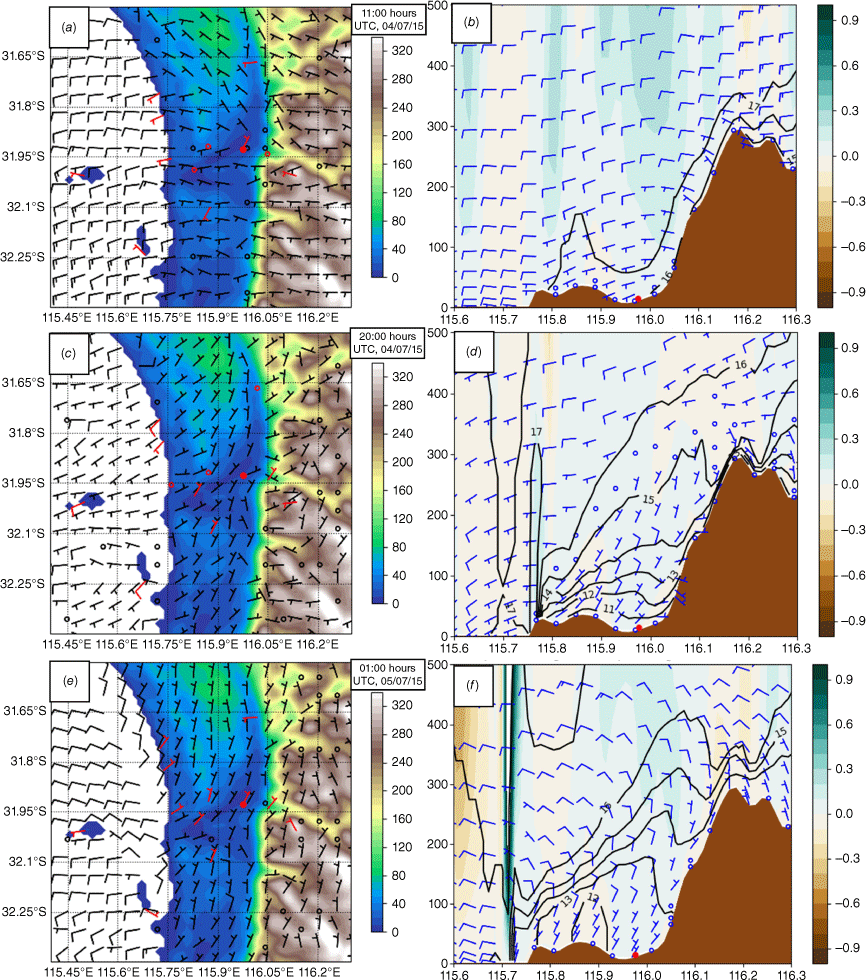
Similar to Case 1, a forecast modelling experiment was conducted with a control run and a run where a flat terrain with an elevation of 1 m is imposed across the land surface. These runs were initialised at 00:00 hours UTC on 4 July 2015, with the 12-km BARRA-R reanalysis providing initial and boundary conditions. Time series of the forecast model fields from the control run were compared with the surface observations at SWAN and Perth Airport (not shown) and like Case 1 there were differences. In particular, the model air temperature was biased high by 2–3°C and the model dew point was biased low by 2–3°C in the evening period. There was also 1–2°C separation between the temperature and dew point in the period when fog was present at Perth Airport. The forecast wind fields were in reasonable agreement with observations.
Fig. 14 shows the plan view of the 10-m winds and cross section through Perth Airport for the control run and flat terrain experiment at 20:00 hours UTC on 4 July 2015, when fog was observed. As with the previous case, there is reasonable agreement with results from this forecast experiment (Fig. 14a, b) and the BARRA-PH reanalysis (Fig. 13c, d), except that the near-surface temperature in the control experiment is a few degrees warmer than in the BARRA-PH reanalysis. It is believed this is largely due to a difference in the land surface description in the forecast model (e.g. Hertwig et al. 2020). The effect of the escarpment is clearly visible in the plan views, with the control showing northerly winds on the coastal plain and north-west winds on the plateau to the east. In the flat terrain experiment (Fig. 14c) there are north-west winds across all the land area in the north, tending west to south-west in the southern part of the domain.
Plan views (left) of topography (shaded) and 10-m wind barbs, with east–west cross-sections (right) along Perth Airport latitude with wind barbs, vertical wind (shades, m s−1) and theta contours (°C) for the control (a, b) and flat terrain (c, d) experiments at 20:00 hours UTC on 4 July (04:00 hours AWST, 5 July). For the wind barbs, a half barb represents 5 kn (~2.57 m s−1), full barb represents 10 kt (~5.14 m s−1) and open black and blue circles are for calm winds (<5 kn, <~2.57 m s−1). Scale for vertical axis in cross-sections is in metres. The closed red dot denotes Perth Airport.
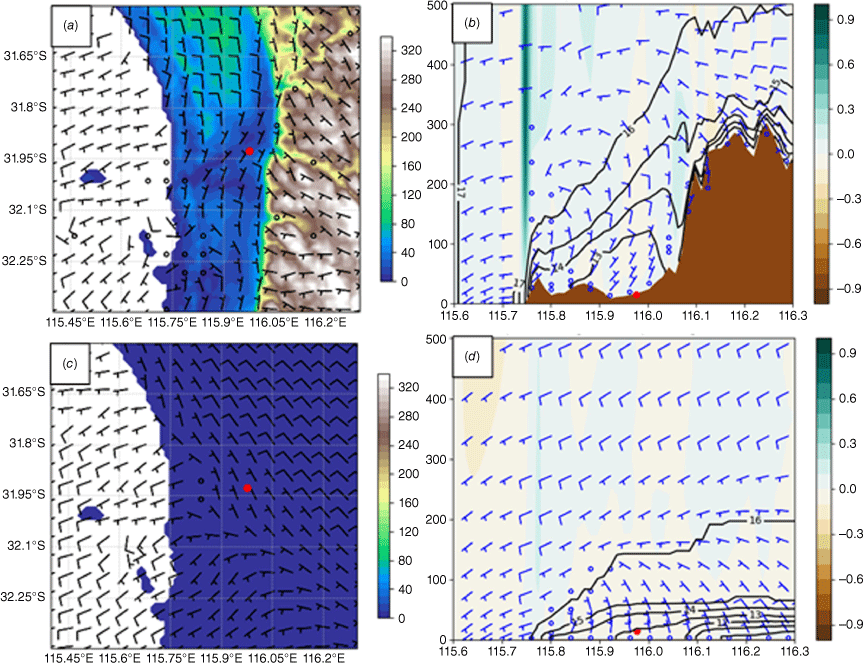
The cross section for the control experiment (Fig. 14b) shows that the escarpment does affect the flow on the coastal plain, with north to north-easterly winds present up to the height of the escarpment (~300 m) and westerly winds over the ocean and aloft. For the flat terrain experiment, Fig. 14d shows a strong temperature inversion over land to an altitude ~100 m with a constant potential temperature above that. There are light south-west winds across the domain tending north-west below the inversion over land. With the light winds and strong inversion, it is reasonable to expect a decoupling of the surface flow from the synoptic flow and a shift in wind direction to the north-west due to surface friction.
Both the BARRA-PH reanalysis (Fig. 15a) and control experiment (Fig. 15b) simulated an extensive area with a higher fog fraction in the Perth region. The BARRA-PH reanalysis has a more extensive and higher fog fraction due to differences in the simulations as mentioned previously. The timing and location of the simulated fog was not a perfect match compared with the satellite imagery (with the simulated fog in the area initiating and clearing a couple of hours earlier than observed) but the timing of the most extensive fog in the area was simulated well. The flat terrain experiment (Fig. 15c) did not simulate fog over Perth, although some fog was simulated to the north and south of the city. With the absence of the northerly near-surface flow in the flat terrain experiment, the fog or moisture to the north of the city did not get advected to the south. The flat-terrain experiment did simulate fog to the south of the city, which in turn was not simulated by the BARRA-PH and control experiments.
5.Discussion
The main aim of this work was to study the physical processes associated with the development of the north to north-easterly near-surface winds frequently observed overnight during fog events at Perth Airport, and their effect on the development of fog. In this study we extend the work of Golding (1993) with 3-D modelling studies, which demonstrate that in light to moderate wind situations (wind speeds of ~2.5 m s−1 or more) the surface flow largely results from topographic blocking.
Two case studies are presented that allow some conclusions on the mechanism for the development of the north to north-east near-surface winds and fog at the airport. There are significant differences between these cases, particularly in the period leading up to the fog event but in both cases the result was a moist airmass at low levels across the area.
For the advection fog case (Case 1, 15 September 2017) there was a West Coast Trough along the west coast directing a warm and dry easterly airflow over Perth during the day. This air moved offshore in the morning with the near-surface air cooling and became more moist due to evaporation from the water. In the early afternoon the air was recirculated back towards the coast following the development of a sea breeze and initiation of the eastward movement of the West Coast Trough. This brought a west to south-west synoptic flow over the Perth area. The back trajectory data showed that the relative humidity of the near-surface recirculated air increased significantly with its passage over water. Changes in the sounding data between 11:00 and 23:00 hours UTC on 15 September suggest that eastward movement of the trough was associated with a cooler drier airmass moving over Perth with a stable layer extending to ~850 hPa. The moist near-surface air trapped by a deep stable layer and blocked by the Darling Scarp created favourable conditions for the development of fog.
The radiation fog case (Case 2, 4 July 2015) differs from Case 1 in that there was a moist westerly air stream during the day on 4 July and into the evening with scattered to broken cloud and some showers before the fog developed, which would have increased the soil moisture content. This situation is relatively common, with the Roux et al. (2021) climatology showing that 43% of fog events have precipitation in the 6 h prior to the fog formation. For this case, AWS observations indicate there was some reduction in the cloud amount before development of the fog, resulting in an increase in radiative cooling.
The two prevailing theories are that the near-surface winds occur because of airmass blocking, or katabatic or drainage flow. In both case studies the northerly winds were constrained to the lower levels of the atmosphere on the coastal plain, below the height of the escarpment. Neither case clearly showed signs of a drainage or katabatic flow along the Darling Scarp, though it should be acknowledged that there may have been local katabatic flows in the vicinity of valleys along the escarpment that are not resolved by the model or available observations. In both cases the gradient airflow is westerly to south-westerly, whereas the near-surface winds on the coastal plain are north to north-easterly, approximately parallel to the escarpment. This supports the theory that the northerly near-surface winds occur as a result of airmass blocking by the escarpment. Both BARRA-PH and the control experiments with the forecast model were generally consistent with available observations, which increases confidence that the 3-D model fields are realistic, even in areas where there were no in situ observations present. Demonstration that blocked flow is an important process in the development of fog at Perth Airport and that the city-scale BARRA data and forecast model fields are realistic is an important result that will be beneficial for further studies directed at better understanding processes associated with the development of fog at airports in Australia.
For the advection fog case and flat terrain, the experiment results showed that the 10-m winds were south-west over water and southerly over the land, whereas the vertical cross-section showed south-west winds at lower altitudes shifting north-west at higher altitudes. A strong surface-based inversion was present over land to a depth of ~100 m. For the radiation fog case and flat terrain experiment results showed the 10-m winds were south-west over water shifting north-west over land. The cross section showed a strong temperature inversion over land to an altitude ~100 m with a constant potential temperature above that. There were light south-west winds across the domain tending north-west below the inversion over land. With the light winds and strong inversion, it is reasonable to expect a decoupling of the surface flow from the synoptic flow and a shift in wind direction to the north-west due to surface friction.
The trapped airmass and light winds associated with blocked flow creates conditions more favourable for the development of fog and this is an important process for Perth Airport given the high incidence of northerly winds on the coastal plain when fog occurs. However, it must be noted that other factors such as moisture content, radiative cooling, turbulence, land surface characteristics and microphysics will influence whether fog does develop.
The land surface appears to have a larger influence on the radiation fog case than on the advection fog case. Bari et al. (2015) postulates that advection fog is largely driven by dynamical processes whereas radiation fog is more driven by radiative processes, turbulence and surface–air interactions. The land surface was found to affect the development of fog in the Korean western peninsula (Choi and Speer 2006). However, sensitivity studies on an advection–radiation fog case in Morocco revealed that the land cover has less influence on numerical predictions of coastal fog than topography and urbanisation. It will be useful to quantify the sensitivity of the model to land cover changes at Perth under different conditions in a future study.
Although north to north-easterly near-surface winds are common (occurring on ~40% of the mornings in the cool season), it is not often that there is enough moisture available for fog to form. The environmental flow coming from the ocean (west) is usually necessary to provide a source for the moisture, but if the winds are too strong there will not be blocking or a strong nocturnal inversion and it will either force the air over the escarpment or simply transport the moisture away before fog can form. The inherently complex interactions of the different processes involved in fog formation and evolution, together with the relative scarcity of fog occurrence at Perth, add to the challenge of understanding the dominant fog processes there. However, detailed examination of the case studies presented in this paper (and other cases not shown), suggest that the combination of the near-surface cooling below a nocturnal inversion, ample moisture from the environmental flow, light near-surface winds and land surface characteristics are all factors creating conditions favourable for the development of fog at Perth Airport. The fog dissipates as temperatures increase after sunrise and the nocturnal inversion weakens.
Data availability
All observations were obtained from the Australian Bureau of Meteorology. The Himawari-8 satellite data were obtained from the Bureau of Meteorology Satellite Observations Collection (https://doi.org/10.25914/61A609F9E7FFA) and the BARRA-PH reanalysis from the BARRA Product suite (https://doi.org/10.4225/41/5993927b50f53) through the National Research Data Repository at NCI.
Conflicts of interest
Steven Siems is the Editor-in-Chief for the Journal of Southern Hemisphere Earth Systems Science (JSHESS) but did not at any stage have editor-level access to this manuscript while in peer review, as is the standard practice when handling manuscripts submitted by an editor to this journal. JSHESS encourages its editors to publish in the journal and they are kept totally separate from the decision-making processes for their manuscripts. The authors have no further conflicts of interest to declare.
Declaration of funding
This research was undertaken with the assistance of resources and services from the National Computational Infrastructure (NCI), which is supported by the Australian Government.
References
Bari D, Bergot T, El Khlifi M (2015) Numerical study of a coastal fog event over Casablanca, Morocco. Quarterly Journal of the Royal Meteorological Society 141, 1894-1905.
| Crossref | Google Scholar |
Bessho K, Date K, Hayashi M, Ikeda A, Imai T, Inoue H, Kumagai Y, Miyakawa T, Murata H, Ohno T, Okuyama A, Oyama R, Sasaki Y, Shimazu Y, Shimoji K, Sumida Y, Suzuki M, Taniguchi H, Tsuchiyama H, Uesawa D, Yokota H, Yoshida R (2016) An introduction to Himawari-8/9 – Japan’s new-generation geostationary meteorological satellites. Journal of the Meteorological Society of Japan 94, 151-183.
| Crossref | Google Scholar |
Best MJ, Pryor M, Clark DB, Rooney GG, Essery RLH, Ménard CB, Edwards JM, Hendry MA, Porson A, Gedney N, Mercado LM, Sitch S, Blyth E, Boucher O, Cox PM, Grimmond CSB, Harding RJ (2011) The Joint UK Land Environment Simulator (JULES), model description – part 1: energy and water fluxes. Geoscientific Model Development 4, 677-699.
| Crossref | Google Scholar |
Blockley JA, Lyons TJ (1994) Airflow over a two‐dimensional escarpment. III: nonhydrostatic flow. Quarterly Journal of the Royal Meteorological Society 120, 79-109.
| Crossref | Google Scholar |
Boutle IA, Eyre JEJ, Lock AP (2014) Seamless stratocumulus simulation across the turbulent gray zone. Monthly Weather Review 142, 1655-1668.
| Crossref | Google Scholar |
Bureau of Meteorology (2010) Operational implementation of the ACCESS Numerical Weather Prediction systems. NMOC Operations Bulletin Number 83. (The Bureau: Melbourne, Vic., Australia) Available at http://www.bom.gov.au/australia/charts/bulletins/apob83.pdf
Bush M, Allen T, Bain C, Boutle I, Edwards J, Finnenkoetter A, Franklin C, Hanley K, Lean H, Lock A, Manners J, Mittermaier M, Morcrette C, North R, Petch J, Short C, Vosper S, Walters D, Webster S, Weeks M, Wilkinson J, Wood N, Zerroukat M (2020) The first Met Office Unified Model/JULES regional atmosphere and land configuration, RAL1. Geoscientific Model Development Discussions 13(4), 1999-2029.
| Crossref | Google Scholar |
Bush M, Boutle I, Edwards J, Finnenkoetter A, Franklin C, Hanley K, Jayakumar A, Lewis H, Lock A, Mittermaier M, Mohandas S, North R, Porson A, Roux B, Webster S, Weeks M (2023) The second Met Office Unified Model/JULES regional atmosphere and land configuration, RAL2. Geoscientific Model Development 16(6), 1713-1734.
| Crossref | Google Scholar |
Choi H, Speer MS (2006) The influence of synoptic-mesoscale winds and sea surface temperature distribution on fog formation near the Korean western peninsula. Meteorological Applications 13, 347-360.
| Crossref | Google Scholar |
Clark PA, Harcourt SA, Macpherson B, Mathison CT, Cusack S, Naylor M (2008) Prediction of visibility and aerosol within the operational Met Office Unified Model. I: model formulation and variational assimilation. Quarterly Journal of the Royal Meteorological Society 134, 1801-1816.
| Crossref | Google Scholar |
Clark DB, Mercado LM, Sitch S, Jones CD, Gedney N, Best MJ, Pryor M, Rooney GG, Essery RLH, Blyth E, Boucher O, Harding RJ, Huntingford C, Cox PM (2011) The Joint UK Land Environment Simulator (JULES), model description – part 2: carbon fluxes and vegetation dynamics. Geoscientific Model Development 4, 701-722.
| Crossref | Google Scholar |
Cuxart J, Prtenjak MT, Matjacic B (2021) Pannonian basin nocturnal boundary layer and fog formation: role of topography. Atmosphere 12, 712.
| Crossref | Google Scholar |
Dare R (2017) Evaluation of numerical model forecasts of visibility and fog at Australian Airports. Research Report Number 024. (Bureau of Meteorology: Melbourne, Vic., Australia) Available at http://www.bom.gov.au/research/publications/researchreports/BRR-024.pdf
Dare RA, Potts R (2019a) A sensitivity study of the fog fraction employed in the ACCESS NWP models. Research Report Number 036. (Bureau of Meteorology: Melbourne, Vic., Australia) Available at http://www.bom.gov.au/research/publications/researchreports/BRR-036.pdf
Dare RA, Potts R (2019b) Sensitivity and tuning of the visibility scheme employed in the ACCESS NWP models. Research Report Number 035. (Bureau of Meteorology: Melbourne, Vic., Australia) Available athttp://www.bom.gov.au/research/publications/researchreports/BRR-035.pdf
Davies T, Cullen MJP, Malcolm AJ, Mawson MH, Staniforth A, White AA, Wood N (2005) A new dynamical core for the Met Office’s global and regional modelling of the atmosphere. Quarterly Journal of the Royal Meteorological Society 131, 1759-1782.
| Crossref | Google Scholar |
Dee DP, Uppala SM, Simmons AJ, Berrisford P, Poli P, Kobayashi S, Andrae U, Balmaseda MA, Balsamo G, Bauer P, Bechtold P, Beljaars ACM, van de Berg L, Bidlot J, Bormann N, Delsol C, Dragani R, Fuentes M, Geer AJ, Haimberger L, Healy SB, Hersbach H, Hólm E V, Isaksen L, Kållberg P, Köhler M, Matricardi M, Mcnally AP, Monge‐Sanz BM, Morcrette JJ, Park BK, Peubey C, de Rosnay P, Tavolato C, Thépaut JN, Vitart F (2011) The ERA-Interim reanalysis: configuration and performance of the data assimilation system. Quarterly Journal of the Royal Meteorological Society 137, 553-597.
| Crossref | Google Scholar |
Draxler RR, Hess GD (1998) An overview of the HYSPLIT_4 modelling system for trajectories, dispersion and deposition. Australian Meteorological Magazine 47, 295-308.
| Google Scholar |
Edwards JM, Slingo A (1996) Studies with a flexible new radiation code. I: choosing a configuration for a large-scale model. Quarterly Journal of the Royal Meteorological Society 122, 689-719.
| Crossref | Google Scholar |
Fernando HJS, Gultepe I, Dorman C, Pardyjak E, Wang Q, Hoch SW, Richter D, Creegan E, Gaberšek S, Bullock T, Hocut C, Chang R, Alappattu D, Dimitrova R, Flagg D, Grachev A, Krishnamurthy R, Singh DK, Lozovatsky I, Nagare B, Sharma A, Wagh S, Wainwright C, Wroblewski M, Yamaguchi R, Bardoel S, Coppersmith RS, Chisholm N, Gonzalez E, Gunawardena N, Hyde O, Morrison T, Olson A, Perelet A, Perrie W, Wang S, Wauer B (2021) C-FOG life of coastal fog. Bulletin of the American Meteorological Society 102, E244-E272.
| Crossref | Google Scholar |
Field PR, Hill A, Shipway B, Furtado K, Wilkinson J, Miltenberger A, Gordon H, Grosvenor DP, Stevens R, Van Weverberg K (2023) Implementation of a double moment cloud microphysics scheme in the UK met office regional numerical weather prediction model. Quarterly Journal of the Royal Meteorological Society 149, 703-739.
| Crossref | Google Scholar |
Golding BW (1993) A study of the influence of terrain on fog development. Monthly Weather Review 121, 2529-2541.
| Crossref | Google Scholar |
Haeffelin M, Dupont JC, Boyouk N, Baumgardner D, Gomes L, Roberts G, Elias T (2013) A comparative study of radiation fog and quasi-fog formation processes during the ParisFog Field Experiment 2007. Pure and Applied Geophysics 170, 2283-2303.
| Crossref | Google Scholar |
Hang C, Nadeau DF, Gultepe I, Hoch SW, Román-Cascón C, Pryor K, Fernando HJS, Creegan ED, Leo LS, Silver Z, Pardyjak ER (2016) A case study of the mechanisms modulating the evolution of valley fog. Pure and Applied Geophysics 173, 3011-3030.
| Crossref | Google Scholar |
Hertwig D, Grimmond S, Hendry MA, Saunders B, Wang Z, Jeoffrion M, Vidale PL, McGuire PC, Bohnenstengel SI, Ward HC, Kotthaus S (2020) Urban signals in high-resolution weather and climate simulations: role of urban land-surface characterisation. Theoretical and Applied Climatology 142, 701-728.
| Crossref | Google Scholar |
Hope PK, Drosdowsky W, Nicholls N (2006) Shifts in the synoptic systems influencing southwest Western Australia. Climate Dynamics 26, 751-764.
| Crossref | Google Scholar |
Lensky IM, Rosenfeld D (2008) Clouds-Aerosols-Precipitation Satellite Analysis Tool (CAPSAT). Atmospheric Chemistry and Physics 8, 6739-6753.
| Crossref | Google Scholar |
Manins PC, Sawford BL (1979) A model of katabatic winds. Journal of the Atmospheric Sciences 36, 619-630.
| Crossref | Google Scholar |
Müller MD, Masbou M, Bott A (2010) Three-dimensional fog forecasting in complex terrain. Quarterly Journal of the Royal Meteorological Society 136, 2189-2202.
| Crossref | Google Scholar |
Nappo CJ, Rao KS (1987) A model study of pure katabatic flows. Tellus A 39 A, 61-71.
| Crossref | Google Scholar |
O’Brien TA, Sloan LC, Chuang PY, Faloona IC, Johnstone JA (2013) Multidecadal simulation of coastal fog with a regional climate model. Climate Dynamics 40, 2801-2812.
| Crossref | Google Scholar |
Pitts RO, Lyons TJ (1989) Airflow over a two‐dimensional escarpment. I: observations. Quarterly Journal of the Royal Meteorological Society 115, 965-981.
| Crossref | Google Scholar |
Pitts RO, Lyons TJ (1990) Airflow over a two‐dimensional escarpment. II: hydrostatic flow. Quarterly Journal of the Royal Meteorological Society 116, 363-378.
| Crossref | Google Scholar |
Puri K, Dietachmayer G, Steinle P, Dix M, Rikus L, Logan L, Naughton M, Tingwell C, Xiao Y, Barras V, Bermous I, Bowen R, Deschamps L, Franklin C, Fraser J, Glowacki T, Harris B, Lee J, Le T, Roff G, Sulaiman A, Sims H, Sun X, Sun Z, Zhu H, Chattopadhyay M, Engel C (2013) Implementation of the initial ACCESS numerical weather prediction system. Australian Meteorological and Oceanographic Journal 63, 265-284.
| Crossref | Google Scholar |
Roux B (2017) ACCESS sensitivity experiments for fog cases at Perth Airport. Research Report 033. (Bureau of Meteorology) Available at http://www.bom.gov.au/research/publications/researchreports/BRR-033.pdf
Roux B, Potts R, Siems S, Manton M (2021) Towards a better understanding of fog at Perth Airport. Journal of Hydrology 600, 126516.
| Crossref | Google Scholar |
Stull R (2017) Practical meteorology: an algebra-based survey of atmospheric science. BMJ 1858, s4-1-57.
| Crossref | Google Scholar |
Su CH, Eizenberg N, Steinle P, Jakob D, Fox-Hughes P, White CJ, Rennie S, Franklin C, Dharssi I, Zhu H (2019) BARRA v1.0: the Bureau of Meteorology atmospheric high-resolution regional reanalysis for Australia. Geoscientific Model Development 12, 2049-2068.
| Crossref | Google Scholar |
Su CH, Eizenberg N, Jakob D, Fox-Hughes P, Steinle P, White CJ, Franklin C (2021) BARRA v1.0: kilometre-scale downscaling of an Australian regional atmospheric reanalysis over four midlatitude domains. Geoscientific Model Development 14, 4357-4378.
| Crossref | Google Scholar |
Tepper G, Watson A (1990) The wintertime nocturnal northeasterly wind of Adelaide, South Australia: an example of topographic blocking in as stably stratified air mass. Australian Meteorological Magazine 38, 281-291.
| Google Scholar |
Van Weverberg K, Morcrette CJ, Boutle I (2021a) A bimodal diagnostic cloud fraction parameterization. Part II: evaluation and resolution sensitivity. Monthly Weather Review 149, 859-878.
| Crossref | Google Scholar |
Van Weverberg K, Morcrette CJ, Boutle I, Furtado K, Field PR (2021b) A bimodal diagnostic cloud fraction parameterization. Part I: motivating analysis and scheme description. Monthly Weather Review 149, 841-857.
| Crossref | Google Scholar |
Wright PB (1974) Seasonal rainfall in southwestern Australia and the general circulation. Monthly Weather Review 102, 219-232.
| Crossref | Google Scholar |
Yimin M, Lyons TJ (2000) Numerical simulation of a sea breeze under dominant synoptic conditions at Perth. Meteorology and Atmospheric Physics 73, 89-103.
| Crossref | Google Scholar |
Yimin M, Lyons TJ, Blockley JA (2001) Surface influences on the Australian West Coast Trough. Theoretical and Applied Climatology 68, 207-217.
| Crossref | Google Scholar |