Atmospheric rivers impacting mainland China and Australia: climatology and interannual variations
Xian-Yun Wu A , Chengzhi Ye B E , Weiwei He C , Jingjing Chen B , Lin Xu B and Huqiang Zhang D EA Hunan Climate Center, Changsha, Hunan, China.
B Hunan Meteorological Observatory, Changsha, Hunan, China.
C Spic Energy Techonology & Engineering Company, Shanghai, China.
D Bureau of Meteorology, GPO Box 1289k, Vic. 3001, Australia.
E Corresponding authors. Email: wfziyuye2001@aliyun.com; Huqiang.Zhang@bom.gov.au
Journal of Southern Hemisphere Earth Systems Science 70(1) 70-87 https://doi.org/10.1071/ES19029
Submitted: 21 November 2019 Accepted: 19 March 2020 Published: 17 September 2020
Journal Compilation © BoM 2020 Open Access CC BY-NC-ND
Abstract
In this study we have built two atmospheric river (AR) databases for mainland China and Australia using Japanese 55-year Reanalysis data with manual detections. By manually checking the magnitude, shape and orientation of vertically integrated vapour transport fields calculated from the reanalysis data and analysing its embedded synoptic patterns and other meteorological information, we detected 625 AR events over mainland China during 1986–2016 and 576 AR events over the Australian continent during 1977–2016. This manuscript documents the mean climatology, spatial distributions, seasonality and interannual variations of ARs occurring in these two regions. We also assessed possible underlying drivers influencing AR activities. Our results showed that: (i) most ARs over mainland China occured in its lower latitudes, including southern, eastern and central China, but ARs also reached its far north and northeast regions. In Australia, most ARs occurred in the states of Western Australia, South Australia and part of New South Wales and Victoria. These regions of high AR frequencies also frequently experienced Northwest Cloud Bands during the cool season; (ii) ARs in China reached their peak during the East Asian summer monsoon season (May–September). This was also the period when AR frequency in the Australian region tended to be higher, but its seasonal variation was weaker than in China; (iii) ARs exhibited large interannual variations in both regions and a declining trend in central and eastern China; (iv) there was a notable influence of tropical sea surface temperatures (SSTs) on the AR activities in the region, with the ARs in Australia being particularly affected by Indian Ocean SSTs and El-Niño Southern Oscillation (ENSO) in the tropical Pacific. ARs in China appear to be affected by ENSO in its decaying phase, with more ARs likely occurring in boreal summer following a peak El Nino during its preceding winter; (v) the Western Pacific Subtropical High plays a dominant role in forming major moisture transport channels for ARs in China, and South China Sea appears to be a key moisture source. In the Australian region, warm and moist air from the eastern part of the tropical Indian Ocean plays a significant role for ARs in the western part of the continent. In addition, moisture transport from the Coral Sea region was an important moisture source for ARs in its east. Results from this study have demonstrated the value of using AR diagnosis to better understand processes governing climate variations in the A–A region.
Keywords: atmospheric rivers, Australia–Asian region, BoM, China, extreme rainfall, integrated vapour transport, interannual variations, manual detection, mean climatology, monsoon.
1 Introduction
An atmospheric river (AR) refers to a narrow and long band of strong water vapour transport, usually originating in tropical or subtropical oceans at lower latitudes and navigating poleward towards the middle and high latitudes (Zhu and Newell 1994; Ralph et al. 2004; Neiman et al. 2008a, 2008b; Lavers et al. 2012). As pointed out by Ye et al. (2020), it is termed a ‘river’ because the amount of atmospheric water vapour flux carried by such a band is close to the water volume of big rivers on the ground. This kind of narrow and long water vapour band, usually associated with a low-level jet ahead of a cyclonic cold front, produces significant rainfall along the North American western coast when encountering coastal mountains and forced by orographic uplift (Newell et al. 1992; Zhu and Newell 1998; Ralph et al. 2004; Bao et al. 2006). As a result, ARs often lead to significant natural disasters, such as floods, mudslides, avalanches, etc. ARs have drawn increasing attention in meteorological and hydrological research communities in recent decades (Newell et al. 1992; Zhu and Newell 1998; Ralph et al. 2004; Bao et al. 2006; Neiman et al. 2008a, 2008b; Lavers et al. 2012; Jiang et al. 2014; Gimeno et al. 2014; Paltan et al. 2017). A large part of early AR research focused on regions such as the west coasts of North America, South America and western Europe.
With increasing AR research occurring in recent years (Ralph et al. 2017), we see more studies documenting AR characteristics in various parts of the world, including the frequency of their occurrence, landing positions, orientations and trajectories. For example, Neiman et al. (2008b) estimated that about 10 ARs on average made landing in California each year. Lavers et al. (2012) suggested that at least 10 ARs that lasted more than 18 hours affected Great Britain in its winter. Guan and Waliser (2015) recently published a set of global AR datasets (https://ucla.box.com/ARcatalog) by applying certain thresholds to analyse vertically integrated vapour transport (IVT) calculated from reanalysis datasets. Based on these results, they quantified averaged AR occurrences, durations, locations and their seasonality and modulations by key climate drivers. A follow-up study by Paltan et al. (2017) further estimated that precipitation from ARs contributed to 22% of total global runoff, or as high as 50% or more in some regions. In some areas, including East Asia, ARs may increase the occurrence of floods by 80%, while absence of ARs may increase the occurrence of hydrological drought events by up to 90% in regions such as the Murry-Darling basin in Australia.
Moreover, the frequency of AR occurrence could change under global climate change. Using regional climate model simulations, Jeon et al. (2015) showed that more ARs of longer durations occurred during 2017–2020 than those during 1981–2005. Radic (2015) suggested that intense AR frequency in autumn in British Columbia could increase with global warming under the Intergovernmental Panel on Climate Change Fifth Assessment Report (IPCC AR5) Representative Concentration Pathway (RCP) 4.5 and RCP 8.5 scenarios. Similarly, Ramos et al. (2016) projected increased AR frequencies and risks of intense precipitation in the European region. Meanwhile, another study by Espinoza et al. (2018) suggested that the ARs will be about 25% longer and 25% wider with 10% less occurrence. They also noted considerable intermodel differences in projected changes over regions that were strongly affected by ARs. These studies provided valuable insights for us to investigate AR activities and their long-term trends in the Australia-Asian (A–A) region, as discussed in this manuscript.
As discussed by Ye et al. (2020), several recent studies showed AR activities in the A–A region (e.g. Guan and Waliser 2015; Hirota et al. 2016; Paltan et al. 2017; Yang et al. 2017). Nevertheless, detailed analyses for ARs are still lacking in the A–A region, which own some unique climatic features. Firstly, the Asian summer monsoon itself is characterised by strong warm and moist air transport from the tropics into the extratropics (Chen et al. 1991; Chang 2004; Ding et al. 2004; Wang 2006; Zhang 2010), but it is unclear to what extent such transport is realised by ARs and/or other processes (Ye et al. 2020). Secondly, China’s geographic location is unique, with a great plateau to its west, warm oceans to its south and a long coastline along its east. This means that the dominant southwesterly monsoon flow from the Bay of Bengal and South China Sea region propagates northeastward along the hillside of the highlands. This is quite different from the AR concept applied to other regions, such as the west coast of North America where ARs directly encountering high coastal mountains is the primary mechanism for producing significant rainfall.
The relevance of AR research to Australian weather and climate is related to its river-like synoptic system known as the Northwest Cloud Band (NWCB). Ye et al. (2020) provided a comprehensive review on the potential connections between ARs and NWCBs in Australia. The NWCB is a long and narrow band seen from satellite images (Tapp and Barrell 1984). It typically extends over 2500 km and exists for two to four days. It often originates from the warm and moist Indian Ocean, south and west of Indonesia. NWCB features bear a strong similarity to the AR phenomenon as discussed. However, there is still a lack of good understanding of the varying effectiveness of NWCBs in generating heavy precipitation (Ummenhofer et al. 2011), with some producing heavy rainfall whereas others just present as cloud bands. Therefore, a detailed AR analysis in the Australian region could help us to better understand the moisture sources and transport associated with NWCBs and how they link to its rainfall generation.
Because of the lack of detailed AR studies in the A–A region, an AR research project was conducted over the period of 2017–2018 involving scientists from the China Meteorological Administration (CMA) and the Australian Bureau of Meteorology (BoM) (Ye et al. 2020). It was designed to fill this research gap and help explore potential AR influences in the A–A region. Ye et al. (2020) discussed the scientific background, key scientific questions and some progresses from this collaboration. Following that, two case studies by Chen et al. (2020) and Xu et al. (2020a) provided some analyses of detailed AR features in China and Australia. They found some different characteristics in AR-induced rainfall generation and potential connections between ARs in China and Australia. In this paper, we document the mean climatologies, seasonalities, spatial distributions and interannual variations of ARs occurring in the two regions and possible underlying drivers for their year-to-year variations.
Accordingly, the manuscript is arranged in the following way. In Section 2, we discuss the method used for building the AR databases for the two countries over the extended periods, the reasons why we devoted significant efforts for conducting AR manual detections and the comparison of ARs from our datasets with some results using automatic detection algorithms. Section 3 is used to document the AR mean climatology, its spatial distributions and interannual variations in mainland China and Australia. In Section 4, we discuss some potential drivers affecting AR activities in the A–A region and the dominant moisture sources for these ARs. Finally, the main conclusions from this study and some future activities are discussed in Section 5.
2 Data and methods
In this study, we used the Japanese 55-year Reanalysis (JRA-55) (https://jra.kishou.go.jp/JRA-55/index_en.html) data for the period of 1977–2016 in the detection of our ARs. JRA-55 has a horizontal resolution of 0.5625°×0.5625° and four times per day (00, 06, 12, 18 UTC). Nevertheless, we only used the data at 00 and 12 UTC in the analysis due to the huge process of manual detection. In order to detect AR events, the IVT was first calculated at each grid as:
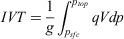
where g is gravitational acceleration, q is specific humidity, V is horizontal wind vector, p is atmospheric pressure, Psfc and Ptop represent atmospheric pressure values at the surface and top of the atmosphere respectively. In our analysis, the IVT was integrated to 300 hPa to ease the task of data processing because a large part of the atmospheric moisture is contained in the troposphere. The same calculation was used in Chen et al. (2020) and Xu et al. (2020a). Similar to many studies (Zhu and Newell 1998; Lavers et al. 2012; Lavers and Villarini 2013; Wick 2014; Guan and Waliser 2015; Mahoney et al. 2016; Mo and Lin 2019), we chose the IVT method for defining ARs, as it contains both wind and moisture information for quantifying moisture transport in contrast to methods that use precipitable water.
As reviewed by Shields et al. (2018), different definitions and thresholds have been used in different studies to define ARs; therefore, ARs detected by different studies for a region could also be different. An intercomparison project is under way to assess the differences in ARs using different detection algorithms (Shields et al. 2018). Of the varied methods used to detect ARs, the one from Guan and Waliser (2015) appeared most attractive to us. In their method, rather than using fixed thresholds, they developed a technique for objective AR detection with varying thresholds based on IVT distributions at each data point derived from a long period. This fit our analysis because ARs in the A–A region are embedded within a large-scale monsoonal environment with significant seasonal variations and large dry–wet transitions (Zhang 2010).
Nevertheless, because there was no reference dataset that we could use to evaluate different AR detection algorithms within such a monsoon climate background, we made great efforts to conduct manual AR detections by combining IVT calculations from JRA-55 data with manual checks of daily synoptic charts and satellite images. This allowed us to ensure that the detected ARs not only satisfied certain IVT thresholds used in automatic detection schemes, but also realistically reflected weather patterns within which ARs form and influence. The detailed procedures used in our manual detections were as follows:
We calculated and visualised twice daily (00 and 12 UTC) IVTs based on the JRA-55 reanalysis over the East Asian and Australian regions separately to obtain detailed IVT spatial features;
Considering the methods applied in Lavers et al. (2012); Nayak et al. (2014) and Guan and Waliser (2015), in which varying thresholds were used to define ARs and to take into account different climate backgrounds where ARs formed, we used different thresholds of IVTs for China and Australia. For mainland China, we largely followed the thresholds used in Guan and Waliser (2015): (i) the maximum IVT for an IVT band is equal to or greater than 250 kg m−1 s−1; (ii) the length of the AR band was equal to or longer than 2000 km; (iii) the ratio of length to width of the AR band was greater than two; and (iv) such a band lasted longer than 24 hours. For Australia, given that it has a relatively dry climate compared to the East Asian monsoon region, we required the AR’s length to be equal to or longer than 1500 km (in contrast to the 2000 km threshold used in China). Our manual detection then ensured that such an IVT band still had the narrow-and-long feature, which is critical for ARs, and it was embedded within suitable weather patterns for its formation and development;
After manually checking the IVT intensity, its geometry and orientation, we then checked some basic synoptic features, clouds and satellite images of any IVT band that met the IVT thresholds. This was to make sure these ARs were supported by large-scale synoptic patterns, such as frontal activities and low-level jets, which are important for AR formation;
The period of AR manual detections was divided into three 10-year chunks analysed by three groups of meteorologists. The results were then cross-checked by these groups to deliver a good quality AR database from manual detections. This also helped to maintain a good consistency of results across the whole period studied by the three groups. Due to limited resources and a large amount of work involved in such a manual detection procedure, we were only able to perform manual AR detection for a 32-year period (1985–2016) in China and a 40-year period (1977–2016) in Australia. These databases only covered the AR events that occurred over the land in these two countries. We did not consider any event that occurred over oceans so that we could concentrate on exploring the potential contribution of ARs to the occurrence of extreme rainfall over land and their hydrological consequences.
Prior to the analysis of the datasets, we conducted some comparisons between ARs detected from our manual detection against ones from the global AR detection dataset released by Guan and Waliser (2015), which we downloaded from https://ucla.box.com/ARcatalog. Obviously, manual detection has a very limited application potential, as it cannot be implemented for operational application nor can it be used in detecting AR variations for a long period due to the amount of work involved. Nevertheless, it is of great value to be used as a reference dataset when developing, comparing and/or improving automatic AR detection algorithms in the A–A region.
During the 31-year period (1986–2016) we found a general agreement between ARs detected from Guan and Waliser (2015) and the ones from our manual detections, but there were also cases where the two datasets differed. Fig. 1 shows such a case during the period of late January–early February 1981. In our manual detection, an AR across the Australian continent was declared at 00 UTC on 21 January 1981, but it was not detected until 06 UTC of 25 January from the dataset of Guan and Waliser (2015). As shown in Fig. 1, a branch of strong moisture transport across the Australian continent had classic AR features, but it was missed in the automatic detection, probably due to some discontinuity or geometry requirement in its detection thresholds. Another undesirable feature in the automatic detection was a break in the system evolution. An AR was declared at 06 UTC of 25 January, dismissed six hours later (at 12 UTC of 25 January) and then re-detected at 18 UTC. In contrast, manual detection viewed these ARs as a single event according to its consistent synoptic evolution during the period. Therefore, manual detection had the advantage of capturing the AR system evolution, continuity and durations based on manually checking the large-scale features where these ARs developed. Another notable result from this comparison was an elongated and intense moisture transport over the tropical Western Pacific Ocean towards the middle latitudes, and this system persisted for a long period. This feature showed the fundamental characteristics of ARs. However, likely due to the fact that this region has a high IVT climatology, such a band did not pass the 85-percentile threshold used in Guan and Waliser (2015), and an AR was only briefly detected at 06 UTC of 25 Jan 1981. We have found a number of such cases over the Asian monsoon region where strong and elongated moisture transport was observed but was not detected in the Guan and Waliser (2015) dataset. This may partly explain the reason why there were less AR occurrences in the Asian monsoon in their results.
![]() |
Note that the focus of this AR collaborative project was not purely on ARs themselves but on using ARs to better understand rainfall variations in these regions. Therefore, in our analysis, we used more than 2400 station rainfall records in mainland China collected by the CMA (available at http://data.cma.cn) and the gridded Australian Water Availability Project rainfall dataset at 0.25°×0.25° resolution (Jones et al. 2009) for the Australian region. These datasets were used to investigate the relationship between ARs and rainfall in these regions.
It is widely acknowledged that tropical sea surface temperatures (SSTs) have significant impacts on the A–A monsoon climate (Webster and Yang 1992; Chang 2004; Ding et al. 2004; Wang 2006; Zhang 2010; Zhang and Moise 2016) through their influence on tropical moisture transport into the extratropics (e.g. Frederiksen and Balgovind 1994; Frederiksen and Frederiksen 1996; Risbey et al. 2009a, 2009b; Frederiksen et al. 2014). Therefore, in our analysis, we used several key climate indices for diagnosing potential underlying drivers of AR variations in the regions, including the Niño 3.4 and the Indian Ocean Dipole (IOD) indices provided by the China National Climate Center (https://cmdp.ncc-cma.net/Monitoring/cn_index_130.php).
3 AR mean climatologies in the A–A region
3.1 Spatial distribution
Based on our manual detections, we identified 625 AR events that occurred over mainland China during 1986–2015, and 576 ARs that occurred in Australia during 1977–2016. Fig. 2 further shows the geographical distributions of these AR events. We divided mainland China into seven regions (southern, eastern, central, southwestern, northwestern, northern and northeastern China) according to their climate features and provincial boundaries (Fig. 2a). In this figure, the size of the red circles represents the total number of AR events that impacted the region. Note that in most cases, an AR event could have impacted more than one region. Results show that China’s southern, central and eastern regions were more likely to be affected by ARs. The maximum number of AR occurrence was 509 in eastern China, suggesting that more than 80% of ARs occurring in China (509/625) impacted its eastern region. The second biggest occurrence was over southern China (486 times), which was equivalent to over 75% of the total occurrence in mainland China. The reason why eastern China has more AR occurrence than its southern part is likely due to the influence of the Western Pacific Subtropical High (WPSH) during the East Asian summer monsoon. As reviewed in Ye et al. (2020) and the case studies by Chen et al. (2020) and Xu et al. (2020a) in this CMA–BOM collaborative project, the anticyclonic flow along the western flank of the WPSH acts as a major moisture channel for the East Asian summer monsoon. The north and westward movement of the WPSH during the boreal summer can lead to strong long and narrow bands of moisture penetrating towards eastern China, resulting in more AR occurrences. Furthermore, with the poleward movement of WPSH, central, north and northeast China also experience an AR influence. Indeed, Chen et al. (2020) showed that ARs in China were supported by both southwesterly monsoon flow that originated from tropical Indian Ocean and the southeasterly flow that originated from the western Pacific associated with WPSH. These two flows formed a strong AR penetrating into north China and caused significant rainfall and floods. Note that in Fig. 2a, there are even a number of ARs detected in the southwest and northwest inland regions. Such results seem difficult to interpret, but recent studies have shown possible mechanisms for the transportation of warm and moist tropical air from the Bay of Bengal and tropical Indian Ocean into such inland regions. One hypothesis is that strong low-level moisture transports along the eastern periphery of the Tibetan Plateau can bring water vapour from the Bay of Bengal and pass it around the Tibetan Plateau to influence rainfall in inland Asia, such as northwest China (Huang et al. 2015). One of our future studies will focus on assessing the potential role of these ARs in linking tropical warm SSTs with heavy inland rainfall in the arid region.
Fig. 2b shows the mean climatology of AR occurrence over the Australian continent. We divided the whole continent into regions based on its administrative states when we counted the total number of ARs for the period of 1977–2016. Western Australia and South Australia were the two states that had the highest number of AR events: about 350 over the 40-year period. They account for over 60% (350/576) of the ARs that occurred over the Australian continent. In addition, there were about 280–330 AR events that further penetrated the southeast states of New South Wales and Victoria. This was roughly 45% of the total AR events detected for Australia during the 40-year period. These results indeed support the notion that moisture sources that originate from the tropics are important for weather and climate in the southeastern corner of the continent (Frederiksen and Balgovind 1994; Cai and Cowan 2008; Ummenhofer et al. 2011; Frederiksen et al. 2014). The overall spatial distribution patterns in Fig. 2b were also in a good agreement with the NWCBs spatial patterns reported by Telcik and Pattiaratchi (2014), which showed high frequencies of NWCBs over the Western Australia and South Australia regions.
3.2 AR seasonality
It is well known that there are significant seasonal reversals of prevailing wind conditions in the A–A monsoon region (ref. Wang 2006; Zhang 2010); thus, we expected the AR activity embedded within such a monsoonal background to have significant seasonal variations. Fig. 3a shows the overall AR seasonal cycle as represented by the total number of ARs identified over mainland China for the period of 1986–2015. These results showed a remarkable seasonal cycle, with most of the AR events occurring in the boreal warm season starting from April/May, reaching their peak in June–July and then decaying from September onwards.
In the Australian region, the total number of ARs occurring over the continent did not show significant seasonal variations (Fig. 3b). Nevertheless, there was a tendency for these events to occur more during the austral cool season from May to July. This agrees with a number of previous studies. Wright (1997) showed that NWCBs tend to occur more frequently in the austral cool season than in other seasons. A study by Telcik and Pattiaratchi (2014), which used outgoing longwave radiation, total cloud cover and surface latent heat flux data to detect NWCBs over the Australian continent between April and October, also showed a higher number of ARs for the months of March–June. Indeed, our results (Fig. 3c) showed that the ARs in Western Australia had an obvious seasonal variation, with higher frequencies from April to September than other seasons, consistent with the results of a NWCB.
3.3 Interannual variations of ARs
Fig. 4 shows the interannual variations of AR occurrence in mainland China and Australia during the two periods we studied. These numbers are the annual totals of ARs that occurred within the two domains respectively. More detailed features are shown in Figs 5 and 6. Based on our manual detection, there was a significant decline in the total number of ARs in mainland China (Fig. 4a), from about 30 per year in the 1980s and 1990s to around 20 per year in the 2010s. There were also significant year-to-year variations, with 1997, 2001/2002 and 2006 having low numbers of ARs. These years also had a short or even empty Meiyu season in eastern China (pers. comm. with Dr. Ping Liang of Shanghai Regional Climate Center in January 2020). Given that ARs in the Asian region mainly occur in the boreal summer, as shown in Figs 5 and 6, the large reduction of ARs in China was mainly contributed by the reduction of ARs in central and eastern China during the boreal summer in June-July-August (JJA). Although exploring the underlying results leading to these reductions warrants further detailed studies, which will be pursued in a separate study, here we only make some brief discussions. Firstly, such a result appears to be contradictory to the findings from other studies, which assessed the potential changes of ARs in other regions under a warm climate. For example, Radic (2015) showed that the British Columbia region could expect more intense ARs with global warming under IPCC AR5 RCP 4.5 and RCP 8.5 scenarios. Ramos et al. (2016) also reported more ARs and increased risks of intense precipitation in the European region. Recent observational and modelling studies also showed increased precipitable water and atmospheric humidity in China (Zhao et al. 2012; Zhang and Zhao 2019). However, the study by Xu et al. (2020b) in this project showed that by analysing 17 CMIP5 models over the Asian region, AR frequency in central and eastern China was reduced under IPCC AR5 RCP 8.5 simulations. This is different from the increases found in other regions as reported by the other aforementioned studies. Xu et al. (2020b) further suggested that the reduction of AR in these regions was likely linked to the trend of a westward expansion of WPSH towards the Asian continent, although the WPSH becomes stronger. The more westward shift means the eastern and central part of China were more impacted by the downward motion of the WPSH, which had significant impacts on regional features of tropical moisture transport into the region (Choi and Kim 2019). Therefore, it is possible that such a reduction of AR frequency, as seen in our analysis, could be partly linked to the potential changes of atmospheric circulation in the region under a warmed climate. Again, we acknowledge this is still an open question that requires further detailed analysis in the future.
Over the Australian continent, annual ARs have significant interannual variations without any significant trend (see Fig. 4b). Our results suggest that the 1980s and late 1990s–early 2000s were periods with lower AR occurrences. In Section 4, we will explore possible climate drivers that led to such variations.
![]() |
![]() |
![]() |
Considering that AR occurrences had significant regional variations (Fig. 2), we further explored some detailed regional features of AR activities and potential linkages to rainfall interannual variations (Fig. 5). We selected three regions (southern, eastern and central China) where most of the ARs in China occurred, as illustrated in Fig. 2a. The AR occurrence in southern China (Fig. 5a) did not show a notable trend over the 31-year period, and its variations did not correlate highly with its rainfall interannual variations. In contrast, AR occurrences in eastern China (Fig. 5b) showed a significant downward trend, with a high number of AR intrusions during the 1980s and a much-reduced number of ARs in the recent decade. Similar patterns were seen in the results for central China (Fig. 5c), where there was a significant downward trend of AR occurrences and a poor correlation between AR occurrences and rainfall variations.
Overall, we did not find any significant connection between ARs and rainfall in the East Asian summer monsoon season. There are a number of possible reasons for this. One could be that East Asia is dominated by strong Asian monsoon flow that transports significant moisture into this region. As a result, any direct contributions from ARs becomes secondary. Secondly, the results in Fig. 5 only showed the total rainfall, and we need to examine the connection between ARs and extreme rainfall in this region. In another study, Liang et al. (2020) already found some significant connections between ARs and extreme rainfall over the lower reaches of the Yangtze River region.
Indeed, there was a much closer connection between ARs and extreme rainfall in China. Fig. 6 shows the correlations between AR frequencies in southern, eastern and central China (the same as shown in Fig. 5) with extreme rainfall amounts over these regions during the AR events. Extreme rainfall is defined as daily rainfall reaching 25 mm. Clearly, the correlations became much stronger, with correlation coefficients reaching 0.58 and 0.47 over central and eastern China respectively. Such results suggest that ARs in the Asian monsoon region affect rainfall extremes in its rainy season. In an unpublished study (pers. comm. with Dr Ping Liang of Shanghai Regional Climate Center), a significant downward trend of extreme rainfall spatial coherence and extreme rainfall durations were seen during the eastern China Meiyu season, which is consistent with the downward trend of ARs in the eastern China region from our results (Fig. 6b). All these factors suggest that although ARs did not significantly influence total rainfall during the Asian monsoon rainy season, they have the potential to change rainfall intensity and temporal and spatial distributions through their strong and steady moisture supply into the region. This needs further thorough investigation in our future studies.
Detailed AR and rainfall interannual variations over the Australian continent are shown in Fig. 7. The analysed regions include Western Australia, South Australia, New South Wales and Victoria, where most of the ARs occurred. We analysed the seasons of May– September over which ARs tended to occur more often. In contrast to the results shown in Fig. 5, here we found strong correlations between the number of ARs and the total rainfall received over these regions. Western Australia had the highest correlation, where we expected more AR events. Strong correlations also existed in the southeast part of the continent where the correlation between AR frequency and rainfall total was around 0.5. Due to the influence of the Great Dividing Ridge, rainfall correlations showed some striking differences between the inland regions to the west of the Great Dividing Ridge and the coastal regions in New South Wales and Victoria. Such a contrast was also shown in other studies (e.g. Pepler et al. 2014). For instance, the El-Niño Southern Oscillation (ENSO) and IOD impacts on rainfall over southeast Australia also showed a difference between the western and eastern sides of the ridge (ref. http://www.bom.gov.au/climate/enso/history/ln–2010–12/ENSO-rainfall.shtml). The stronger AR-rainfall correlation in the Australian region compared to China can also be partly understood by the fact that Australia is a dry continent and moisture supply is critical to its rainfall generation. Therefore, moisture transport associated with ARs plays an important role in influencing its rainfall variations. These results support previous studies (Frederiksen and Balgovind 1994; Frederiksen and Frederiksen 1996; Cai et al. 2011; Ummenhofer et al. 2011) that showed a remote influence of Indian Ocean SST conditions on rainfall in southeast Australia via tropical moisture transport towards the Australian continent.
3.4 Potential climate drivers modulating AR activities in the A-A region
In section 3.2, we showed considerable year-to-year variations of the number of ARs that occurred in China and Australia. In this section, we focus on exploring potential underlying drivers leading to such variations. It is well known that climate in Australia and China is impacted by tropical Pacific and tropical Indian Ocean SSTs. Many studies (ref. Webster and Yang 1992; Chang 2004; Ding et al. 2004; Wang 2006) have shown such impacts on the Asian monsoon climate. Further, the influence from the tropical Pacific and Indian oceans on the Australian climate has also been well documented (Nicholls et al. 1982; Drosdowsky 1996; Frederiksen and Frederiksen 1996; Cai et al. 2011; Ummenhofer et al. 2009, 2011; Risbey et al. 2009b).
In our analysis, to represent impacts from the tropical Pacific, we used the well-known Southern Oscillation Index (SOI) and Niño 3.4 index, whereas for the Indian Ocean influence, we used the Dipole Mode Index (DMI: Saji et al. 1999), which measures the anomalous zonal SST gradient across the equatorial Indian Ocean defined as the difference between SST anomaly in western (60°E–80°E, 10°S–10°N) and eastern (90°E–110°E, 10°S–0°S) tropical Indian Ocean. In addition, we also used the Tropical Indian Ocean Dipole (TIOD) from Ramsay et al. (2017), which they identified as a leading mode of Indian Ocean SSTs in the southern hemisphere. The TIOD measures the difference in SSTs anomalies between the areas of 20–5°S, 90–120°E and 30–15°S, 60–85°E, and Ramsay et al. (2017) showed its influence on the Australian climate. Note that we acknowledge the complexity of the connections between SST warmings over the Indian Ocean and tropical Pacific. Studies have shown that some of the Indian Ocean SST variations could be in response to the effects of tropical Pacific SSTs (e.g. Krishnamurthy and Kirtman 2003; Chowdary and Gnanaseelan 2007), whereas others have argued that the Indian Ocean SSTs influence SST variations in the tropical Pacific (e.g. Annamalai et al. 2007; Luo et al. 2012). However, separating out such processes was not the focus of this analysis.
Fig. 8 shows the correlations of AR frequencies in three Australian regions (Western Australia, South Australia, and New South Wales and Victoria) with the four indices for the AR peak season in May–September. Clearly, both Indian Ocean and tropical Pacific Ocean SSTs had significant impacts on the AR activities in these regions. Fewer ARs were expected when IOD or TIOD had their positive phases. Indeed, during the IOD positive phases, the SST over the eastern tropical Indian Ocean was cooler than its climatology. Such SST conditions and their induced atmospheric circulation responses were not favourable for the transport of tropical warm moisture from the Indian Ocean into the Australian continent. The highest correlation occurred over the southeast part of the region, and this supports the results from many previous studies (Frederiksen and Frederiksen 1996; Ummenhofer et al. 2009, 2011) that highlighted the role of Indian Ocean SSTs in influencing the climate in the southeast part of the continent. In addition, our results showed significant positive correlations between AR frequency and SOI in the Australian regions, suggesting significant ENSO influence. During the El Nino years (with negative SOI and positive Niño 3.4 SSTs), eastern Australia tended to experience a dry climate, particularly during the austral winter/spring season (ref: http://www.bom.gov.au/climate/enso/ninocomp.shtml). The shift of tropical Walker circulation towards the eastern Pacific and the weakened upward motion over the maritime continent region did not favour the formation of AR in the Australian region due to a more poleward shift of the subtropical high. The study by Xu et al. (2020a) underlined the importance of the position of this high system in determining the formation of AR in the region. Their results support the statistical relationship shown in Fig. 8.
![]() |
In contrast, the ENSO or IOD impacts on the AR activities in China were much weaker. This was not unexpected, as many studies have revealed complex relationship between the Asian monsoon and the tropical SSTs in the Pacific and Indian basins (ref. Chen et al. 1991; Ding et al. 2004; Chang 2004; Wang 2006). Many other factors, such as the Eurasian and Tibetan Plateau land-surface conditions and internal variability of atmospheric circulations (e.g. middle latitude blockings), are also important contributing factors to the activity of the East Asian summer monsoon. In addition, the monsoon-SST relationship depends on ENSO developing and decaying phases. Fig. 9 suggests no correlations between these key climate indices and the AR activities in the boreal summer over mainland China. Nevertheless, we did see some delayed impacts when correlating these AR activities with their preceding boreal winter SOI or Niño 3.4 index (Fig. 10). For the AR frequency, the most significant correlations existed for the central China region, with more ARs occurring after the decaying phase of ENSO (warm Niño 3.4 SSTs and negative SOIs) in its preceding winter. This can be understood by the fact that tropical Indian Ocean SSTs, following the ENSO decaying phase, tend to intensify and shift westward of the WPSH (Xie et al. 2009; Matsumura and Horinouchi 2016). This can result in more AR occurrence in the central part of China because the intensity and location of the WPSH is critical for the formation of ARs in the region (Xu et al. 2020a). Such processes can also result in longer durations of ARs once they are formed because the WPSH is a semistationary system that can sustain large-scale circulation setups required for the ARs. Furthermore, these correlation results agree with results from previous studies that indicated East Asian summer rainfall tended to increase during the decaying phase of ENSO (Zhang et al. 1999; Wang et al. 2000; Zhang et al. 2016). In addition, our results suggested some potential impacts of TIOD on the AR occurrences in southern and eastern parts of China. Note that the TIOD was identified as the leading mode for affecting austral winter (boreal summer) rainfall in the Australian region by Ramsay et al. (2017). The mechanism for understanding its impacts on the Asian summer monsoon is unclear and warrants more thorough investigations.
![]() |
4 Characteristics of atmospheric moisture transport associated with ARs
Understanding AR moisture source was an important part of this study. In this section, we firstly use the Empirical Orthogonal Function (EOF) method to analyse the 625 ARs’ IVT fields that impacted mainland China (Fig. 11). As the majority of the ARs in mainland China occurred in boreal summer (ref. Fig. 3), these results largely reflected the dominant moisture transport patterns in its summer season. Fig. 11 shows the first three leading EOF patterns of IVT fields corresponding to the 625 ARs. Together they explain 36% of the total IVT field variance. As the eigenvalue of the fourth EOF drops significantly (not shown), these three patterns largely reflected the dominant processes for understanding atmospheric moisture sources of ARs in the region. These results clearly showed three dominant moisture transport channels within the East Asian monsoon system. As discussed in Ye et al. (2020), these well-known moisture channels (ref. Chen et al. 1991; Chang 2004; Wang 2006) are important for the formation and maintenance of ARs in the East Asian monsoon region. The EOF1 (Fig. 11a) represented moisture transport associated with a westward extension of the WPSH, with an anticyclonic flow dominating the South China Sea and warm pool region. This led to significant moisture transport by southwesterly flows that penetrated towards southern, central and eastern China. This mode of transport accounted for more than 20% percent of the total IVT field variance and was clearly the most important one.
![]() |
EOF2 (Fig. 11b) accounted for 8% of the total IVT variance and was the second leading IVT pattern for ARs in mainland China. In contrast to EOF1, its circulation pattern corresponded to a northerly shift of the WPSH with an anomalous anticyclonic flow that dominated far East Asia, Japan and the Korean Peninsula. This southeasterly anticyclonic flow, together with an enhanced southerly flow from the South China Sea and tropical western Pacific, brought more tropic moisture into eastern and northern China and supported the AR occurrence in the region.
The third dominant pattern (Fig. 11c) was very much constrained in the tropics. It started from the Bay of Bangel, moved eastward through the Indo-China Peninsula and then strengthened over the South China Sea and Philippines region. This pattern explained about 7% of the total IVT variance and was the third leading component. In the context of this analysis, IVT influence on AR activities was therefore largely limited to southern China.
Note that one common feature among these three patterns was that all of them had a component of moisture source from the South China Sea. Many studies have shown that the South China Sea is a key region of water vapour supply for supporting the regional rainfall for southeast China, where most floods are closely related to atmospheric circulation (South China Sea monsoon) and deep convections in the region (Chen et al. 1991; Chang 2004; Ding et al. 2004). There are increasing studies of the South China Sea monsoon and how it influences summer weather and climate in the extratropics of East Asia (Ding et al. 2004; Huang and Mao 2015). The EOF analysis of the AR IVT fields successfully captured this important role.
Nevertheless, the same EOF analysis applied to the 576 ARs in the Australian region did not deliver meaningful results (not shown). We attributed this to the fact that the AR seasonality in the Australia region is weak (ref. Fig. 3). Considering that a large part of the Australian continent is dominated by the Australian monsoon, which has significant seasonal circulation variations (Zhang and Moise 2016), without separating out ARs over different seasons, the same EOF analysis as shown in Fig. 11 would not reveal meaningful results. Therefore, for the Australian region, we conducted EOF analysis only for the ARs that occurred during the May-June-July-August period (Fig. 12).
![]() |
Fig. 12a shows the first EOF pattern of IVT that highlights the importance of strong moisture transport from the eastern part of the tropical Indian Ocean towards the western part of the Australian continent. The pattern is closely associated with the passage of a deep middle latitude cyclonic system from the southern Indian Ocean that moved westward into the southwest part of the Australian continent. The northwest–southeast orientation resembled the NWCB patterns, which often occurred during the austral cool season. This pattern explained about 13% of the total IVT variance and was the most important pattern corresponding to the ARs in the Australian region during its cool season.
The second leading EOF pattern (Fig. 12b) also included a component of strong moisture transport from the eastern part of the tropical Indian Ocean. At the same time, there was significant moisture input from the Coral Sea region over the eastern part of the continent. This EOF pattern was close to the circulation patterns shown in the case study by Xu et al. (2020a), which showed two ARs influencing the Australian continent: a west one that largely contributed by moisture from the Indian Ocean and an east one from the Coral Sea. In contrast to EOF1, this EOF pattern appeared to be dominated more by the influence of tropical systems. This pattern explained about 9.5% of the total IVT variance and is the second dominant IVT pattern associated with ARs over the Australian continent.
The third dominant pattern (Fig. 12c) showed the influence of a low system that dominated the Tasman Sea. It explained about 8% the total IVT variance. This cyclonic system, resembling the features of East Coast Lows (Hopkins and Holland 1997; Mills et al. 2010), was favourable for bringing in a warm and moist airmass from the tropical Coral Sea region into the eastern part of the continent. Indeed, the close linkage between East Coast Lows and extreme rainfall over the eastern seaboard of the Australian continent has been acknowledged by many studies, of which a chief factor is the significant tropical moisture transport into the region (Hopkins and Holland 1997; Mills et al. 2010; Pepler et al. 2014). These studies reported that rains associated with the East Coast Lows extended for some distance and were often constrained to a narrow rainband (~100 km across), as recently documented by Bruyere et al. (2019). All these moisture transport channels share the same characteristics of ARs, and the EOF analysis in this study successfully captured these features.
5 Conclusion and discussions
As detailed by Ye et al. (2020), as part of a larger collaborative project between the CMA and the BoM, we documented AR (Zhu and Newell 1994; Ralph et al. 2004; Neiman et al. 2008a, 2008b; Lavers et al. 2012) mean climatology, seasonality and interannual variations over mainland China and Australia. Our results were based on AR databases built using JRA-55 reanalysis with manual detections. The database was developed by manually checking the magnitude, shape and orientation of vertically IVT fields at 00 and 12 UTC, calculated from the six-hourly reanalysis data and other synoptic information. A range of definitions and thresholds for ARs have been used in various recent studies (ref. Shields et al. 2018), which will be used as a reference dataset for developing and selecting a suitable method for AR automatic detections in the A–A monsoon region in our future work.
From our results, we found there were 625 AR events that impacted mainland China during 1986–2016 and 576 AR events that occurred over the Australian continent during 1977–2016. Most ARs over mainland China occurred in its lower latitudes, including southern, eastern and central China. However, ARs also reached its north and northeast. These results are supported by case studies from Chen et al. (2020) and Xu et al. (2020a). In Australia, most ARs occurred in the states of Western Australia, South Australia and part of the New South Wales and Victoria. These regions had high frequencies of ARs and tended to experience more NWCBs (Tapp and Barrell 1984).
ARs affecting mainland China showed a significant seasonality associated with strong seasonal changes of prevailing winds and moisture transport in the East Asian monsoon (Chen et al. 1991; Chang 2004; Wang 2006; Zhang 2010). They reached their peak during the East Asian summer monsoon season (May–September). In addition, they exhibited large interannual variations and showed a declining trend in China. This declining trend was consistent with the AR projections based on 17 CMIP5 model simulations by Xu et al. (2020b). In contrast, although ARs over the Australian continent tended to occur more often in its cool season (particularly in the western part of the continent), the seasonality was much weaker than in China. There was no clear trend in the Australian region for the period of 1977–2016, but they exhibited significant year-to-year variations.
The underlying climate drivers modulating the AR activities in these regions was also investigated by this study. We showed that the ARs in Australian were significantly affected by Indian Ocean SSTs and ENSO in the tropical Pacific. However, such an influence was much weaker for ARs in China, largely due to the complex processes governing the Asian monsoon (Webster and Yang 1992; Chang 2004; Wang 2006). Nevertheless, ARs in China appeared to be affected by ENSO in its decaying phase, with more ARs tending to occur in the boreal summer following peak El Nino during its preceding winter.
Finally, we investigated the key regions of moisture sources that support the ARs in the two regions. Using EOF analysis, we showed that the WPSH plays a dominant role in forming the dominant moisture transport channels for ARs in China. This is supported by the results from Chen et al. (2020) and Xu et al. (2020a), which demonstrated processes related to WPSH for influencing atmospheric moisture transport in the East Asian region. Furthermore, our analysis identified the South China Sea as a key moisture source influencing ARs in China, which is in good agreement with many studies (Ding et al. 2004; Huang and Mao 2015). In the Australian region, as many previous studies have shown (Frederiksen and Frederiksen 1996; Ummenhofer et al. 2009, 2011), the warm and moist air from the eastern part of the tropical Indian Ocean played a significant role for ARs in the western part of the continent. We also found that moisture transport from the Coral Sea region into the eastern part of the continent was important for ARs in the eastern part of the Australia. Such moisture transport was linked to a low system over the Tasman Sea, which shares a high similarity with East Coast Lows and associated heavy rainfall (Hopkins and Holland 1997; Mills et al. 2010; Pepler et al. 2014; Bruyere et al. 2019).
Despite the efforts in this project to develop valuable AR datasets that covered Australia and mainland China and allowed us to document the AR climatology, seasonality and interannual variations, there were still significant issues that need to be addressed in our future studies. Firstly, although extreme care was taken when doing our AR manual detections, there are inevitably some human errors in these detections. We are willing to share our datasets with others, which can further validate these results and improve our understanding of ARs in the A–A region. Secondly, as we have acknowledged in the analysis, we only calculated some simple correlations between ARs and rainfall and have not yet explored further linkages between ARs and extreme rainfall. Our preliminary analysis clearly showed that ARs in East Asia had significant impacts on the rainfall extremes, although their direct impacts on total rainfall was weak during the monsoon rainy season. A recent study by Liang et al. (2020) from this project reported stronger connections between ARs and extreme rainfall in the lower reaches of the Yangtze River Basin in part of eastern China, although the correlation between ARs and total rainfall in the monsoon region was low. We need to further investigate this important aspect. Finally, we found a significant declining trend of ARs in China for the period we examined. Although such results were consistent with the analysis of 17 CMIP5 models by Xu et al. (2020b), we do not know the detailed processes leading to such a trend. Further investigation of the possible linkage of this trend with changes in large-scale circulation and East Asian monsoon activities is needed, including its potential linkage to global warming, as discussed by other studies (Ramos et al. 2016; Espinoza et al. 2018). This will be further addressed by the study from Xu et al. (2020b).
Conflicts of interest
The authors declare that they have no conflicts of interest.
Acknowledgements
The work was conducted under a bilateral project (JWG–16 4.1) between the Australian Bureau of Meteorology and China Meteorological Administration on atmospheric river studies in the Australia-Asian region. Scientists from Hunan were supported by the Key Scientific Research Project of Hunan Meteorological Bureau (XQKJ16A001, XQKJ17D001). The authors highly appreciate email exchanges with Dr B. Guan and discussions with Dr. D. Waliser of JPL about their AR research and their publication of a global AR dataset, which was used in this study. Discussions with Drs A. Moise, R. Mo, G. Martin, P. Wu, K. Furtado, R. Clark, P. Liang, G. Dong, B. Chen and Y. Xu during the study are acknowledged. We thank the comments and suggestions from Drs. V. Kumar and L. Qi during the internal review process. We appreciate the very constructive comments and suggestions from the two anonymous reviewers.
References
Annamalai, H., Hamilton, K., and Sperber, K. R. (2007). The South Asian summer monsoon and its relationship with ENSO in the IPCC AR4 simulations. J. Climate 20, 1071–1092.| The South Asian summer monsoon and its relationship with ENSO in the IPCC AR4 simulations.Crossref | GoogleScholarGoogle Scholar |
Bao, J.-W., Michelson, S. A., and Neiman, P. J. (2006). Interpretation of enhanced integrated water vapor bands associated with extratropical cyclones: Their formation and connection to tropical moisture. Mon. Wea. Rev. 134, 1063–1080.
| Interpretation of enhanced integrated water vapor bands associated with extratropical cyclones: Their formation and connection to tropical moisture.Crossref | GoogleScholarGoogle Scholar |
Bruyère, C., Holland, G., Prein, Done, J., Buckley, B., Chan, P., Leplastrier, M. and Dyer, A. (2019). Severe Weather in a Changing Climate. Insurance Australia Group, 67pp
Cai, W., and Cowan, T. (2008). Dynamics of late autumn rainfall reduction over southeastern Australia. Geophys. Res. Lett. 35, L09708.
| Dynamics of late autumn rainfall reduction over southeastern Australia.Crossref | GoogleScholarGoogle Scholar |
Cai, W., van Rensch, P., Cowan, T., and Hendon, H. H. (2011). Teleconnection pathways of ENSO and the IOD and the mechanisms for impacts on Australian rainfall. J. Climate 24, 3910–3923.
| Teleconnection pathways of ENSO and the IOD and the mechanisms for impacts on Australian rainfall.Crossref | GoogleScholarGoogle Scholar |
Chang, C. P. (Editor) (2004). East Asian Monsoon. World Scientific, 572pp
Chen, L. X., Zhu, Q. G. and Luo, H. B. (1991). East Asian Monsoon. (China Meteorological Press), 362 pp. (in Chinese)
Chen, J., Zhang, H., Ye, C., Chen, H., and Mo, R. (2020). Case studies of atmospheric rivers over China and Australia: new insight into their rainfall generation. J. South. Hemisph. Earth Syst. Sci. , .
| Case studies of atmospheric rivers over China and Australia: new insight into their rainfall generation.Crossref | GoogleScholarGoogle Scholar |
Choi, W., and Kim, K. (2019). Summertime variability of the western North Pacifc subtropical high and its synoptic influences on the East Asian weather. Scientific Report. (2019) 9, 7865.
| Summertime variability of the western North Pacifc subtropical high and its synoptic influences on the East Asian weather.Crossref | GoogleScholarGoogle Scholar |
Chowdary, J. S., and Gnanaseelan, C. (2007). Basin-wide warming of the Indian Ocean during El Nino and Indian Ocean dipole years. Int. J. Climatol. 27, 1421–1438.
| Basin-wide warming of the Indian Ocean during El Nino and Indian Ocean dipole years.Crossref | GoogleScholarGoogle Scholar |
Ding, Y., Li, C., and Liu, Y. (2004). Overview of the South China Sea monsoon experiment. Adv. Atmos. Sci. 21, 343–360.
| Overview of the South China Sea monsoon experiment.Crossref | GoogleScholarGoogle Scholar |
Drosdowsky, W. (1996). Variability of the Australian summer monsoon at Darwin: 1957–1992. J. Climate 9, 85–96.
| Variability of the Australian summer monsoon at Darwin: 1957–1992.Crossref | GoogleScholarGoogle Scholar |
Espinoza, V., Waliser, D., Guan, B., Lavers, D., and Ralph, F. (2018). Global analysis of climate change projection effects on Atmospheric Rivers. Geophys. Res. Lett. 45, 4299–4308.
| Global analysis of climate change projection effects on Atmospheric Rivers.Crossref | GoogleScholarGoogle Scholar |
Frederiksen, C. S., and Balgovind, R. C. (1994). The influence of the Indian Ocean/Indonesian SST gradient on the Australian winter rainfall and circulation in an atmospheric GCM. Quart. J. Roy. Meteor. Soc. 120, 923–952.
| The influence of the Indian Ocean/Indonesian SST gradient on the Australian winter rainfall and circulation in an atmospheric GCM.Crossref | GoogleScholarGoogle Scholar |
Frederiksen, C. S., and Frederiksen, J. S. (1996). A Theoretical Model of Australian Northwest cloudband disturbances and Southern Hemisphere storm tracks: The role of SST anomalies. J. Atmos. Sci. 53, 1410–1432.
| A Theoretical Model of Australian Northwest cloudband disturbances and Southern Hemisphere storm tracks: The role of SST anomalies.Crossref | GoogleScholarGoogle Scholar |
Frederiksen, C. S., Zheng, X., and Grainger, S. (2014). Teleconnections and predictive characteristics of Australian seasonal rainfall. Clim. Dyn. 43, 1381–1408.
| Teleconnections and predictive characteristics of Australian seasonal rainfall.Crossref | GoogleScholarGoogle Scholar |
Gimeno, L., Nieto, R., Vázquez, M., and Lavers, D. A. (2014). Atmospheric rivers: a mini-review. Front. Earth Sci. 2, 2.
| Atmospheric rivers: a mini-review.Crossref | GoogleScholarGoogle Scholar |
Guan, B., and Waliser, D. E. (2015). Detection of atmospheric rivers: Evaluation and application of an algorithm for global studies. J. Geophys. Res. Atmos. 120, 12514–12535.
| Detection of atmospheric rivers: Evaluation and application of an algorithm for global studies.Crossref | GoogleScholarGoogle Scholar |
Hirota, N., Takayabu, Y. N., Kato, M., and Arakane, S. (2016). Roles of an atmospheric river and a cutoff low in the extreme precipitation event in Hiroshima on 19 August 2014. Mon. Wea. Rev. 144, 1145–1160.
| Roles of an atmospheric river and a cutoff low in the extreme precipitation event in Hiroshima on 19 August 2014.Crossref | GoogleScholarGoogle Scholar |
Hopkins, L. C., and Holland, G. J. (1997). Australian heavy-rain days and associated east coast cyclones: 1958–92. J. Climate 10, 621–635.
| Australian heavy-rain days and associated east coast cyclones: 1958–92.Crossref | GoogleScholarGoogle Scholar |
Huang, H., and Mao, W. (2015). The South China Sea Monsoon Experiment—Boundary Layer Height (SCSMEX-BLH): Experimental design and preliminary results. Mon. Wea. Rev. 143, 5035–5053.
| The South China Sea Monsoon Experiment—Boundary Layer Height (SCSMEX-BLH): Experimental design and preliminary results.Crossref | GoogleScholarGoogle Scholar |
Huang, W., Feng, S., Chen, J., and Chen, F. (2015). Physical mechanisms of the summer precipitation variations in the Tarim Basin, Northwestern China. J. Climate 28, 3579–3591.
| Physical mechanisms of the summer precipitation variations in the Tarim Basin, Northwestern China.Crossref | GoogleScholarGoogle Scholar |
Jeon, S., Prabhat, , Byna, S., Gu, J., Collins, W. D., and Wehner, M. F. (2015). Characterization of extreme precipitation within atmospheric river events over California. Adv. Stat. Clim. Meteorol. Oceanogr. 1, 45–57.
| Characterization of extreme precipitation within atmospheric river events over California.Crossref | GoogleScholarGoogle Scholar |
Jiang, T., Evans, K. J., Deng, Y., and Dong, X. (2014). Intermediate frequency atmospheric disturbances: A dynamical bridge connecting western U.S. extreme precipitation with East Asian cold surges. J. Geophys. Res. Atmos. 119, 3723–3735.
| Intermediate frequency atmospheric disturbances: A dynamical bridge connecting western U.S. extreme precipitation with East Asian cold surges.Crossref | GoogleScholarGoogle Scholar |
Jones, D. A., Wang, W., and Fawcett, R. (2009). High-quality spatial climate data-sets for Australia. Aust. Meteorol. Oceanogr. J. 58, 233–248.
| High-quality spatial climate data-sets for Australia.Crossref | GoogleScholarGoogle Scholar |
Krishnamurthy, V., and Kirtman, B. P. (2003). Variability of the Indian Ocean: relation to monsoon and ENSO. Quart. J. Roy. Meteor. Soc. 129, 1623–1646.
| Variability of the Indian Ocean: relation to monsoon and ENSO.Crossref | GoogleScholarGoogle Scholar |
Lavers, D. A., and Villarini, G. (2013). The nexus between atmospheric rivers and extreme precipitation across Europe. Geophys. Res. Lett. 40, 3259–3264.
| The nexus between atmospheric rivers and extreme precipitation across Europe. Geophys. Crossref | GoogleScholarGoogle Scholar |
Lavers, D. A., Villarini, G., Allan, R. P., Wood, E. F., and Wade, A. J. (2012). The detection of atmospheric rivers in atmospheric reanalyses and their links to British winter floods and the large‐scale climatic circulation. J. Geophys. Res. Atmos. 117, D20106.
| The detection of atmospheric rivers in atmospheric reanalyses and their links to British winter floods and the large‐scale climatic circulation.Crossref | GoogleScholarGoogle Scholar |
Liang, P., Dong, G., Zhang, H., Zhao, M., and Ma, Y. (2020). Atmospheric rivers associated with summer heavy rainfall over the Yangtze Plain. J. South. Hemisph. Earth Syst. Sci. , .
| Atmospheric rivers associated with summer heavy rainfall over the Yangtze Plain.Crossref | GoogleScholarGoogle Scholar |
Luo, J. J., Sasaki, W., and Masumoto, Y. (2012). Indian Ocean warming modulates Pacific climate change. Proc. Nat. Acad. Sci. USA 109, 18701–18706.
| Indian Ocean warming modulates Pacific climate change.Crossref | GoogleScholarGoogle Scholar | 23112174PubMed |
Mahoney, K., Jackson, D. L., Neiman, P., Hughes, M., Darby, L., Wick, G., White, A., Sukovich, E., and Cifelli, R. (2016). Understanding the role of atmospheric rivers in heavy precipitation in the southeast United States. Mon. Wea. Rev. 144, 1617–1632.
| Understanding the role of atmospheric rivers in heavy precipitation in the southeast United States.Crossref | GoogleScholarGoogle Scholar |
Matsumura, S., and Horinouchi, T. (2016). Pacific Ocean decadal forcing of long-term changes in the western Pacific subtropical high. Sci. Rep. 6, 37765.
| Pacific Ocean decadal forcing of long-term changes in the western Pacific subtropical high.Crossref | GoogleScholarGoogle Scholar | 27901052PubMed |
Mills, G. A., Webb, R., Davidson, N. E., Kepert, J., Seed, A. and Abbs, D. (2010). The Pasha Bulker east coast low of 8 June 2007. CAWCR Technical Report No. 023. Available at https://www.cawcr.gov.au/technical-reports/CTR_023.pdf
Mo, R., and Lin, H. (2019). Tropical-midlatitude interactions: Case study of an inland-penetrating atmospheric river during a major winter storm over North America. Atmos.-Ocean. 57, 208–232.
| Tropical-midlatitude interactions: Case study of an inland-penetrating atmospheric river during a major winter storm over North America.Crossref | GoogleScholarGoogle Scholar |
Nayak, M. A., Villarini, G., and Lavers, D. A. (2014). On the skill of numerical weather prediction models to forecast atmospheric rivers over the central United States. Geophys. Res. Lett. 41, 4354–4362.
| On the skill of numerical weather prediction models to forecast atmospheric rivers over the central United States.Crossref | GoogleScholarGoogle Scholar |
Neiman, P. J., Ralph, F. M., Wick, G. A., Kuo, Y.-H., Wee, T.-K., Ma, Z., Taylor, G. H., and Dettinger, M. D. (2008a). Diagnosis of an intense atmospheric river impacting the Pacific Northwest: Storm summary and offshore vertical structure observed with COSMIC satellite retrievals. Mon. Wea. Rev. 136, 4398–4420.
| Diagnosis of an intense atmospheric river impacting the Pacific Northwest: Storm summary and offshore vertical structure observed with COSMIC satellite retrievals.Crossref | GoogleScholarGoogle Scholar |
Neiman, P. J., Ralph, F. M., Wick, G. A., Lundquist, J. D., and Dettinger, M. D. (2008b). Meteorological characteristics and overland precipitation impacts of atmospheric rivers affecting the West Coast of North America based on eight years of SSM/I satellite observations. J. Hydrometeor. 9, 22–47.
| Meteorological characteristics and overland precipitation impacts of atmospheric rivers affecting the West Coast of North America based on eight years of SSM/I satellite observations.Crossref | GoogleScholarGoogle Scholar |
Newell, R. E., Newell, N. E., Zhu, Y., and Scott, C. (1992). Tropospheric rivers?–A pilot study. Geophys. Res. Lett. 19, 2401–2404.
| Tropospheric rivers?–A pilot study.Crossref | GoogleScholarGoogle Scholar |
Nicholls, N., McBride, J. L., and Ormerod, R. J. (1982). On predicting the onset of the Australian west season at Darwin. Mon. Wea. Rev. 110, 14–17.
| On predicting the onset of the Australian west season at Darwin.Crossref | GoogleScholarGoogle Scholar |
Paltan, H., Waliser, D., Lim, W. H., Guan, B., Yamazaki, D., Pant, R., and Dadson, S. (2017). Global floods and water availability driven by atmospheric rivers. Geophys. Res. Lett. 44, 10387–10395.
| Global floods and water availability driven by atmospheric rivers.Crossref | GoogleScholarGoogle Scholar |
Pepler, A., Timbal, B., Rakich, C., and Coutts-Smith, A. (2014). Indian Ocean Dipole overrides ENSO’s influence on cool season rainfall across the eastern seaboard of Australia. J. Climate 27, 3816–3826.
| Indian Ocean Dipole overrides ENSO’s influence on cool season rainfall across the eastern seaboard of Australia.Crossref | GoogleScholarGoogle Scholar |
Pepler, A., Coutts‐Smith, A., and Timbal, B. (2014). The role of East Coast Lows on rainfall patterns and inter‐annual variability across the East Coast of Australia. Int. J. Climatol. 34, 1011–1021.
| The role of East Coast Lows on rainfall patterns and inter‐annual variability across the East Coast of Australia.Crossref | GoogleScholarGoogle Scholar |
Radić, V., Cannon, A. J., Menounos, B., and Gi, N. (2015). Future changes in autumn atmospheric river events in British Columbia, Canada, as projected by CMIP5 global climate models. J. Geophys. Res. Atmos. 120, 9279–9302.
| Future changes in autumn atmospheric river events in British Columbia, Canada, as projected by CMIP5 global climate models.Crossref | GoogleScholarGoogle Scholar |
Ralph, F. M., Dettinger, M., Lavers, D., Gorodetskaya, I. V., Martin, A., Viale, M., White, A. B., Oakley, N., Rutz, J., and Spackman, J. R. (2017). Atmospheric rivers emerge as a global science and applications focus. Bull. Amer. Meteor. Soc. 98, 1969–1973.
| Atmospheric rivers emerge as a global science and applications focus.Crossref | GoogleScholarGoogle Scholar |
Ralph, F. M., Neiman, P. J., and Wick, G. A. (2004). Satellite and CALJET aircraft observations of atmospheric rivers over the eastern North Pacific Ocean during the winter of 1997/98. Mon. Wea. Rev. 132, 1721–1745.
| Satellite and CALJET aircraft observations of atmospheric rivers over the eastern North Pacific Ocean during the winter of 1997/98.Crossref | GoogleScholarGoogle Scholar |
Ramos, A. M., Nieto, R., Tomé, R., Gimeno, L., Trigo, R. M., Liberato, M. L. R., and Lavers, D. (2016). Atmospheric rivers moisture sources from a lagrangian perspective. Earth Syst. Dyn. 7, 371–384.
| Atmospheric rivers moisture sources from a lagrangian perspective.Crossref | GoogleScholarGoogle Scholar |
Ramsay, H. A., Richman, M. B., and Leslie, L. M. (2017). The modulating influence of Indian Ocean sea surface temperatures on Australian region seasonal tropical cyclone counts. J. Climate 30, 4843–4856.
| The modulating influence of Indian Ocean sea surface temperatures on Australian region seasonal tropical cyclone counts.Crossref | GoogleScholarGoogle Scholar |
Risbey, J. S., Pook, M. J., McIntosh, P. C., Ummenhofer, C. C., and Meyers, G. (2009a). Characteristics and variability of synoptic features associated with cool season rainfall in southeastern Australia. Int. J. Climatol. 29, 1595–1613.
| Characteristics and variability of synoptic features associated with cool season rainfall in southeastern Australia.Crossref | GoogleScholarGoogle Scholar |
Risbey, J. S., Pook, M. J., McIntosh, P. C., Wheeler, M. C., and Hendon, H. H. (2009b). On the remote drivers of rainfall variability in Australia. Mon. Wea. Rev. 137, 3233–3253.
| On the remote drivers of rainfall variability in Australia.Crossref | GoogleScholarGoogle Scholar |
Saji, N. H., Goswami, B. N., Vinayachandran, P. N., and Yamagata, T. (1999). A dipole mode in the tropical Indian Ocean. Nature 401, 360–363.
| A dipole mode in the tropical Indian Ocean.Crossref | GoogleScholarGoogle Scholar | 16862108PubMed |
Shields, C. A., Coauthors. (2018). Atmospheric River tracking method intercomparison project (ARTMIP): Project goals and experimental design. Geosci. Model Dev. 11, 2455–2474.
| Atmospheric River tracking method intercomparison project (ARTMIP): Project goals and experimental design.Crossref | GoogleScholarGoogle Scholar |
Tapp, R. G., and Barrell, S. L. (1984). The north-west Australian cloud band: Climatology, characteristics and factors associated with development. Int. J. Climatol. 4, 411–424.
| The north-west Australian cloud band: Climatology, characteristics and factors associated with development.Crossref | GoogleScholarGoogle Scholar |
Telcik, N., and Pattiaratchi, C. (2014). Influence of Northwest Cloudbands on Southwest Australian rainfall. J. Climatology 2014, 671394.
| Influence of Northwest Cloudbands on Southwest Australian rainfall.Crossref | GoogleScholarGoogle Scholar |
Ummenhofer, C. C., England, M. H., McIntosh, P. C., Meyers, G., Pook, M. J., Risbey, J. S., Sen Gupta, A., and Taschetto, A. S. (2009). What causes southeast Australia’s worst droughts. Geophys. Res. Lett. 36, 1–5.
| What causes southeast Australia’s worst droughts.Crossref | GoogleScholarGoogle Scholar |
Ummenhofer, C. C., Coauthors. (2011). Indian and Pacific Ocean influences on southeast Aus-tralian drought and soil moisture. J. Climate 24, 1313–1336.
| Indian and Pacific Ocean influences on southeast Aus-tralian drought and soil moisture.Crossref | GoogleScholarGoogle Scholar |
Wang, B. (2006). The Asian Monsoon. (Springer: Heidelberg, Germany.)
Wang, B., Wu, R., and Fu, X. (2000). Pacific–East Asian teleconnection: How does ENSO affect East Asian climate? J. Climate 13, 1517–1536.
| Pacific–East Asian teleconnection: How does ENSO affect East Asian climate?Crossref | GoogleScholarGoogle Scholar |
Webster, P. J., and Yang, S. (1992). Monsoon and ENSO: Selectively interactive systems. Quart. J. Roy. Meteor. Soc. 118, 877–926.
| Monsoon and ENSO: Selectively interactive systems.Crossref | GoogleScholarGoogle Scholar |
Wright, W. J. (1997). Tropical–extratropical cloudbands and Australian rainfall: I. Climatology. Int. J. Climatol. 17, 807–829.
| Tropical–extratropical cloudbands and Australian rainfall: I. Climatology.Crossref | GoogleScholarGoogle Scholar |
Wick, G. A. (2014). Implementation and initial application of an atmospheric river detection tool based on integrated vapor transport. 2014 Fall Meeting, San Francisco, CA, Amer. Geophys. Union, Abstract A34E-06.
Xie, S.-P., Hu, K., Hafner, J., Tokinaga, H., Du, Y., Huang, G., and Sampe, T. (2009). Indian Ocean capacitor effect on Indo–western Pacific climate during the summer following El Niño. J. Climate 22, 730–747.
| Indian Ocean capacitor effect on Indo–western Pacific climate during the summer following El Niño.Crossref | GoogleScholarGoogle Scholar |
Xu, L., Zhang, H., He, W., Ye, C., and Moise, A. (2020a). Potential connections between atmospheric rivers in China and Australia. J. South. Hemisph. Earth Syst. Sci. , .
| Potential connections between atmospheric rivers in China and Australia.Crossref | GoogleScholarGoogle Scholar |
Xu, Y., Zhang, H., Liu, Y., Han, Z., and Zhou, B. (2020b). Atmospheric rivers in the Asia–Australian region under current and future climate in CMIP5 models. J. South. Hemisph. Earth Syst. Sci. , .
| Atmospheric rivers in the Asia–Australian region under current and future climate in CMIP5 models.Crossref | GoogleScholarGoogle Scholar |
Yang, Y., Zhao, T., Ni, G., and Sun, T. (2017). Atmospheric rivers over the Bay of Bengal lead to northern Indian extreme rainfall. Int. J. Climatol. 38, 1010–1021.
| Atmospheric rivers over the Bay of Bengal lead to northern Indian extreme rainfall.Crossref | GoogleScholarGoogle Scholar |
Ye, C., Zhang, H., Moise, A., and Mo, R. (2020). Atmospheric rivers in the Australia-Asian region: a BoM–CMA collaborative study. J. South. Hemisph. Earth Syst. Sci. , .
| Atmospheric rivers in the Australia-Asian region: a BoM–CMA collaborative study.Crossref | GoogleScholarGoogle Scholar |
Zhang, H. (2010). Diagnosing Australia-Asian monsoon onset/retreat and using large-scale wind and moisture indices. Clim. Dyn. 35, 601–618.
| Diagnosing Australia-Asian monsoon onset/retreat and using large-scale wind and moisture indices.Crossref | GoogleScholarGoogle Scholar |
Zhang, H. and Moise, A. (2016). The Australian summer monsoon in current and future climate. In ‘The Monsoons and Climate Change’. (Eds L. M. V. de Carvalho and C. Jones) pp. 67–120. (Springer.)
Zhang, J., and Zhao, T. (2019). Historical and future changes of atmospheric precipitable water over China simulated by CMIP5 models. Clim. Dyn. 52, 6969–6988.
| Historical and future changes of atmospheric precipitable water over China simulated by CMIP5 models.Crossref | GoogleScholarGoogle Scholar |
Zhang, W., Li, H., Stuecker, M. F., Jin, F.-F., and Turner, A. G. (2016). A new understanding of El Niño’s impact over East Asia: Dominance of the ENSO combination mode. J. Climate 29, 4347–4359.
| A new understanding of El Niño’s impact over East Asia: Dominance of the ENSO combination mode.Crossref | GoogleScholarGoogle Scholar |
Zhang, R., Sumi, A., and Kimoto, M. (1999). A diagnostic study of the impact of El Niño on the precipitation in China. Adv. Atmos. Sci. 16, 229–241.
| A diagnostic study of the impact of El Niño on the precipitation in China.Crossref | GoogleScholarGoogle Scholar |
Zhao, T., Dai, A., and Wang, J. (2012). Trends in tropospheric humidity from 1970 to 2008 over China from a homogenized radiosonde dataset. J. Climate 25, 4549–4567.
Zhu, Y., and Newell, R. E. (1994). Atmospheric rivers and bombs. Geophys. Res. Lett. 21, 1999–2002.
| Atmospheric rivers and bombs.Crossref | GoogleScholarGoogle Scholar |
Zhu, Y., and Newell, R. E. (1998). A proposed algorithm for moisture fluxes from atmospheric rivers. Mon. Wea. Rev. 126, 725–735.
| A proposed algorithm for moisture fluxes from atmospheric rivers.Crossref | GoogleScholarGoogle Scholar |