The spatial distribution of soil nitrogen determines responses of Sorghum bicolor to banded phosphorus fertiliser
Megan A. Hunter
A
B
C
D
E
Abstract
The grain-growing areas of north-eastern Australia are a major producer of grain for human and livestock consumption, but declining soil nitrogen (N) and phosphorus (P) fertility is increasing fertiliser requirements to sustain productivity. Adding a concentrated zone of fertiliser P to the subsoil (i.e. a ‘deep P’ band) is an effective strategy to increase plant P uptake in farming systems reliant on stored soil water. However, crop responses to deep P with contrasting soil N status remain unclear.
This study aimed to assess responses of sorghum (Sorghum bicolor) to fertiliser P with contrasting distributions of soil N.
A lysimeter experiment was conducted in semi-controlled environment, where sorghum was grown to physiological maturity in P depleted Vertosol with contrasting fertiliser N and P additions.
Responses of sorghum to deep P were optimised when bands were placed in N enriched soil in the 0–20 cm layer, producing comparable biomass to when P was dispersed throughout the soil volume. Localised root proliferation around the deep P band was maximised when bands were placed into N-enriched soil, however plant P uptake was only 77% of that with dispersed P.
Sorghum responses to deep P were affected by the distribution of soil N within the top 60 cm of the soil profile, with maximum dry matter production, N and P uptake occurring when high concentrations of N and P were co-located in the 0–20 cm layer.
Adequate N status of the upper soil profile is required to optimise sorghum responses to deep P.
Keywords: deep P, lysimeter, nitrogen, northern grains region, P responsiveness, phosphorus, soil N distribution, Vertisol.
Introduction
The grain-growing regions of north-eastern Australia extend from mid-northern New South Wales to central Queensland and cover approximately 4 million ha of agricultural land (Webb et al. 1997; Chauhan and Rachaputi 2014). Vertosols, Chromosols, and Sodosols are the predominant soil types, which were historically of high soil fertility (Webb et al. 1997). However, long-term cropping of these soils with consistently negative nutrient budgets has caused soil fertility to decline and resulted in an increased reliance on mineral fertilisers to overcome crop nutrient deficiencies (Webb et al. 1997; Angus et al. 2020). In general, nitrogen (N) and phosphorus (P) are the two most limiting nutrients affecting crop production within the region (Dalal and Probert 1997), with potassium (K) limitations of increasing concern (Angus et al. 2020). Crop responses to the addition of N and P fertiliser are now widespread across the region, however knowledge relating to the most effective fertiliser management strategies to optimise crop growth is lacking.
The placement of P fertiliser in a concentrated zone or ‘band’ within the subsoil layer (15–20 cm below the soil surface) is often referred to as ‘deep P’ placement. In general, this has been an effective management strategy to improve crop P uptake and yield responses within grains-growing region of north-eastern Australia (Bell et al. 2015; Sands et al. 2020; Bell et al. 2020; Lester et al. 2022). This can be largely attributed to:
The frequent drying of topsoil layers caused by sporadic and highly variable in-season rainfall. This increases the reliance of crops on nutrients (and water) sourced from subsoil layers (Singh et al. 2005; Ma et al. 2009).
The widespread adoption of minimal and zero-till systems across the region, the regular addition of fertiliser to the topsoil layer, and nutrient removal at depth by crops. These have increasingly caused the vertical stratification of available nutrients in the soil (Wang et al. 2007; Angus et al. 2020). This stratification is particularly severe for nutrients such as P that are comparatively ‘immobile’, and limits plant root access to soil P during large parts of the growing season because topsoil layers dry out (Wang et al. 2007).
Consequently, the application of deep P supports the more effective co-location of plant-available P, adequate soil moisture, and active root systems for nutrient acquisition compared to P situated in shallow topsoil layers in this region (Singh et al. 2005; Ma et al. 2009; Raymond et al. 2021). However, there are situations where crop responses to deep P have been variable (Lester et al. 2022). A better understanding of factors determining crop responsiveness will help improve the recovery of added fertiliser P and crop production in the region.
Variable crop responses to deep P across the north-eastern cropping region of Australia may be due to several co-limiting factors. Most notably, soil N status is likely to affect uptake of deep P by cereals due to differences in nutrient accessibility and crop demand. Given that increased tiller production is a critical mechanism through which cereal yields are increased by improved N or P supply (Youngquist and Maranville 1992; Chen et al. 2019), the effect of N supply on tillering may be a key factor governing cereal P responsiveness. Specifically, the timing of N access relative to the finite growth period when tillers are initiated (Hammer et al. 2023) may be important, with insufficient N supply during this time likely to constrain tillering responses despite access to sufficient P, even if more N becomes accessible later in growth.
Despite their importance, the spatio-temporal relationships between N and P remain poorly understood. The diverse range of soil N dynamics experienced across different locations and seasonal conditions means that the quantity of N available for crop uptake is highly variable through time and space, complicating the ability to identify if, or when, cereal deep P responses are limited by N. Moreover, the timing of N access is highly dynamic, largely driven by the frequency and movement of water through the soil profile (Cox and Strong 2017). Considering this complexity and variability, an improved understanding of the N supply dynamics (amount, spatial and temporal availability) to crops and its interaction with fertiliser P will require experimental conditions that carefully simulate the target environment, including precise control of water dynamics in realistic soil conditions, whilst avoiding potential artefacts such as changed root–shoot biomass partitioning due to root volume restrictions at later stages of growth (Yang et al. 2010).
We hypothesise that the responses of sorghum (Sorghum bicolor) to fertiliser P will be affected by the spatial distribution of soil N, with responses limited by delayed access to N relative to when N is accessed early in growth. To assess this, we conducted a semi-controlled experiment in which different contrasting combinations of fertiliser N and P were placed in large (60 kg) repacked soil cores. Sorghum was grown to physiological maturity using an automated lysimeter platform to carefully control water availability over time. The objectives of this experiment were to assess: (1) sorghum growth responses to fertiliser P with contrasting soil N concentrations in different parts of the soil profile; (2) sorghum responses to P fertiliser placement including deep P bands; and (3) plant N and P uptake with contrasting soil N distribution and fertiliser P placement.
Materials and methods
Soil collection and preparation
A Grey Vertosol soil (Isbell 2016) was collected from a long-term cropping site near Hopelands in the Western Downs region of south-eastern Queensland, Australia. Soil was taken from the subsoil (10–60 cm) layer, air-dried, passed through a crusher (JC3020 jaw crusher, Alsto, Perth, Australia) to <1 cm, and homogenised. Background chemical properties of this soil are in Table 1. Notably, the concentration of plant-available P, as estimated by the method of Colwell (1963), was 6 mg P kg−1, indicating that a crop response to added fertiliser P was highly likely in this soil (Moody and Bolland 1999). Furthermore, the alkaline pH of the soil used in this study indicates that much of the banded P fertiliser was likely to have remained in plant available forms throughout the experimental period (Raymond et al. 2023; McKenna et al. 2024).
Soil parameter | Value | |
---|---|---|
Colwell P (mg kg−1) A | 6.0 | |
Nitrate-N (mg kg−1) B | 46.5 | |
Sulfur (mg kg−1) C | 26.2 | |
Colwell K (mg kg−1) D | 393.5 | |
Electrical conductivity (dS m−1) E | 0.5 | |
pH E | 8.0 | |
PBI F | 113.0 | |
Copper (mg kg−1) G | 1.0 | |
Iron (mg kg−1) G | 11.8 | |
Manganese (mg kg−1) G | 11.7 | |
Zinc (mg kg−1) G | 0.6 | |
Exc. aluminium (cmol(+) kg−1) E | 0.1 | |
Exc. calcium (cmol(+) kg−1) E | 17.6 | |
Exc. magnesium (cmol(+) kg−1) E | 8.5 | |
Exc. potassium (cmol(+) kg−1) E | 0.9 | |
Exc. sodium (cmol(+) kg−1) E | 2.2 | |
Organic carbon (g kg−1) H | 5.4 | |
Total carbon (g kg−1) I | 10.6 |
Analyses were carried out on air-dry soil ground to <2 mm.
Experimental design
The experiment involved nine treatments with five replicates, arranged in a randomised incomplete block design. Treatments included:
Two N rates of ammonium nitrate (NH4NO3) to add either 70 mg N kg−1 air-dry soil (‘moderate’) or 150 mg N kg−1 air-dry soil (‘high’);
Four N placement strategies that included three zones of N enrichment in discrete soil layers (0–20 cm, ‘top’; 20–40 cm, ‘middle’; or 40–60 cm, ‘bottom’), and N enrichment of the total soil volume (‘uniform’); and
Two P placement strategies that included the supply of 60 mg P kg−1 (equivalent to 3.6 g P per pot) as monoammonium phosphate (MAP) to air-dry soil throughout the total soil volume (‘dispersed P’), and 600 mg P per column in a deep band placed at 20 cm below the soil surface (‘banded P’) (Fig. 1).
This experiment comprised a total of nine treatments, including two P placement strategies (dispersed P and banded P); two soil N concentrations (high at 150 mg N per kg soil and moderate at 70 mg N per kg soil); and four soil N distributions (uniformly distributed, and three discrete N enriched layers in either the top (0–20 cm), middle (20–40 cm), or bottom (40–60 cm) of the column). The dispersed P treatment supplied 60 mg P kg−1 as MAP uniformly supplied throughout the soil volume to provide 3.6 g P per pot. The banded P treatment comprised a horizontal band containing 600 mg P per column as MAP placed at 20 cm deep (i.e. ‘deep P’). Both P placements received an additional 44 mg of P per column placed at 3 cm deep (i.e. ‘starter P’, not shown).
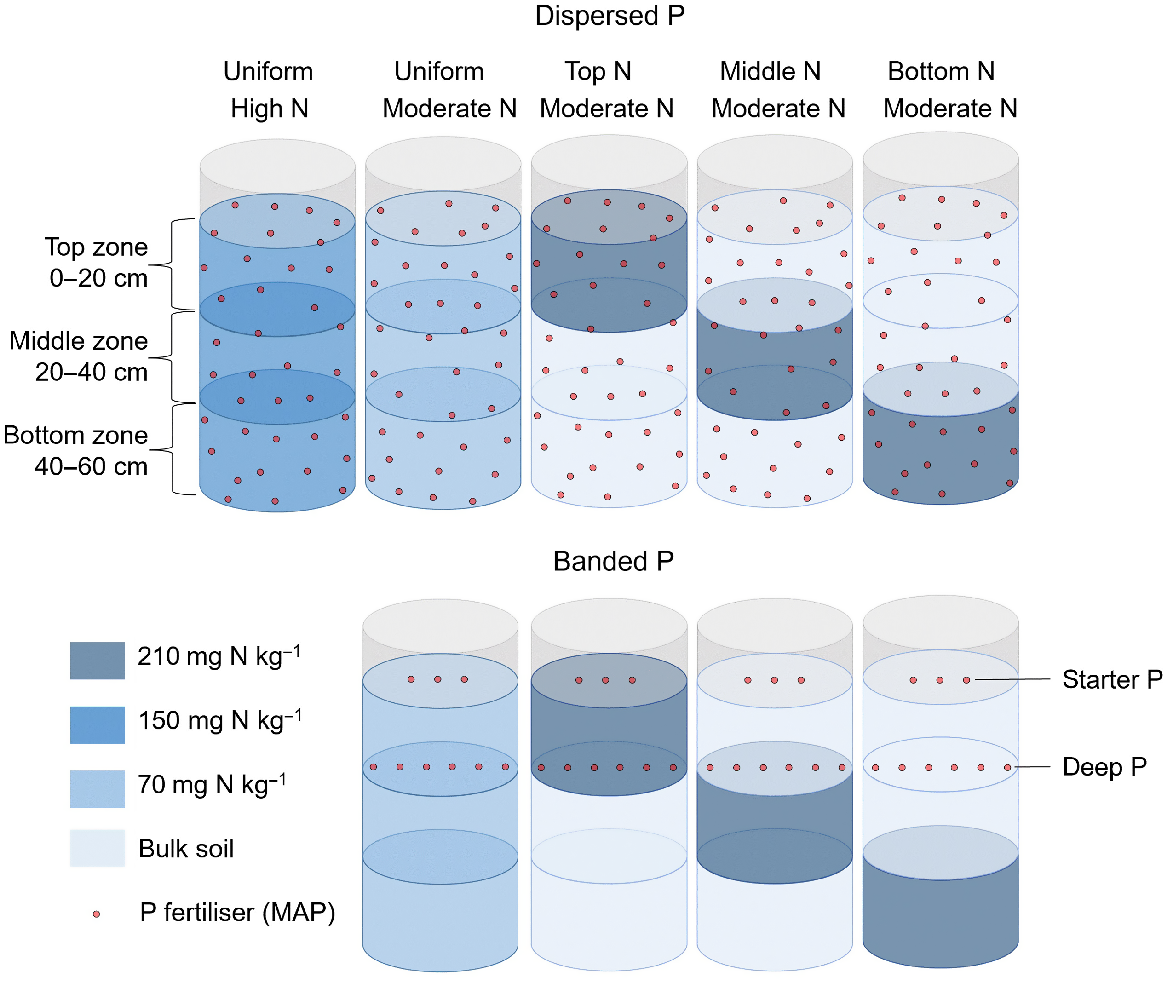
The lower rates of P addition in the banded treatments (compared to the dispersed P treatments that represented a ‘positive P control’) were consistent with P concentrations in deep bands used in commercial practice, i.e. 40 kg P ha−1 at 0.5 m band spacings (Angus et al. 2020). These applications have been shown to promote localised root proliferation and improved plant P uptake in sorghum in a previous lysimeter study with this soil (van der Bom et al. 2023a). The ‘Dispersed P/Uniform N’ treatments were included as a benchmark for optimal plant growth and nutrient uptake in this soil.
All treatments received granular MAP to supply 44 mg P per column as ‘starter P’, placed in a band that was 3 cm below the soil surface adjacent to the seeding furrow. This achieved an application rate equivalent to 3 kg P ha−1 in bands spaced 0.5 m apart, analogous to typical fertiliser rates in the region (Bell et al. 2010; Bell and Lester 2014). The dispersed and banded P treatment received further additions of MAP or NH4NO3 to reach a common basal rate of 27.4 mg N kg−1 air-dry soil, taking into account that MAP not only contains P, but also has a content of ca. 10% N. Potassium was added as ground muriate of potash (MOP) to supply 150 mg K kg−1 air-dry soil. In addition, foliar zinc (Zn) was applied as a solution of 0.5% zinc sulfate heptahydrate and 1.5% urea at 29, 34, 40 and 47 days after sowing (DAS).
Location of experiment
The experiment was carried out in the root lysimeter facility at the Gatton Campus of The University of Queensland, Australia (28.06°S, 151.96°E). This facility has been described in detail by van der Bom et al. (2023a). Briefly, this involved 10 temperature-controlled tables that each held eight soil columns (‘pots’) containing 60 kg of air-dried soil that were each mounted on individual load cells. The temperature within the root compartment of each table and the irrigation schedule of each individual soil column was controlled through a wireless connection to a central user interface. This interface also allowed for the real time monitoring of column weight (kg), volumetric soil water (%) and soil temperature (°C).
All tables were situated in a screenhouse made of UV stabilised polyurethane (PE) that prevented rainfall entering the facility while allowing penetration of approximately 70–80% of incident radiation. Fans at either end of the space promote lateral air flow to prevent excessive heat accumulation. A weather station located within the screenhouse recorded relative humidity (RH%), radiation (kWh m−2), and ambient temperature (°C). Minimum and maximum ambient temperatures within the facility averaged 18.4 (°C) and 33.2 (°C), respectively, during the experimental period.
Column construction
Soil columns were constructed from polyvinyl chloride (PVC) cylinders that were 73 cm high and 30 cm in diameter. Each was lined with a polyethylene (PE) liner to facilitate removal of soil and roots after harvest. To minimise the risk of waterlogging, 2 L of expanded perlite was placed in the bottom of each cylinder. A total of 60 kg of air-dry soil was placed in each column in three discrete 20 kg soil layers to allow the creation of different N distributions for each P placement (Fig. 1). A thin layer of expanded pearlite (0.5 L) was placed between each soil layer to facilitate the identification of soil layers at harvest.
A loose weave nylon bag with a mesh size of 2.5 mm, and containing 2.5 kg of air-dry topsoil, was placed in each column at a soil depth of 20 cm. The P fertiliser band was placed within these bags in the banded P treatments. By adding a consistently sized mesh bag in the same location in each column, root growth in the band zone could be directly compared across P placements and soil N distributions for comparison to the positive P controls (i.e. the dispersed P treatments).
All columns were wet up to approximately 83% of field capacity over a 7-day period prior to sowing. This was based on soil water characteristic curves established for this soil by van der Bom et al. (2023a). Three soil sensors (RS485 Modbus Soil Temperature Moisture Sensor, model BND-SNS, Modbus, Shenzhen, China) were placed into each column at approximate soil depths of 5, 10, and 40 cm to monitor gravimetric water content (%) over time and depth, and ensure that no parts of the soil profile became too wet (due to bypass flow through upper soil layers) or too dry (due to water extraction in deeper profile layers).
Plant growth and management
Sorghum (Sorghum bicolor L. Moench) cv. MR-Buster, a widely grown commercial variety, was sown on the 6 January 2022. Six seeds were sown in each column at a depth of approximately 2 cm below the soil surface, and subsequently thinned to two uniformly sized plants per column at 14 days after sowing (DAS). The establishment of two plants per column was chosen to ensure adequate biomass accumulation and nutrient demand to promote potential treatment effects. Furthermore, this planting density also approximates industry recommendations for irrigated sorghum (Butler et al. 2003). All columns were hand watered for the first 2 weeks to ensure plant establishment.
Water was periodically added to each column through irrigation probes located approximately 5 cm and 20 cm below the soil surface to maintain the column target weights equivalent to 83% field capacity for the duration of the study. Regular insecticide was applied as required to ensure optimal plant health.
Data collection and harvest
Tiller number (tillers per plant) was recorded weekly on the same plant in each column.
Destructive biomass sampling was carried out on a single occasion at physiological maturity (105 DAS). Aboveground biomass was collected by cutting the stem at the surface of the soil and partitioning biomass into shoots and panicles. Where applicable, tillers were separated from the main stem and similarly partitioned into shoots and panicles. All biomass samples were dried at 65°C until constant weight, with total aboveground dry matter accumulation expressed as g DM per column. All plant samples were ground to <2 mm using a cutting mill grinder (SM 400, Retsch, Haan, Germany) prior to chemical analysis.
Plant samples were analysed for total N via Dumas high-temperature combustion using a LECO analyser (LECO, Castle Hill, NSW, Australia) based on the method of Matejovic (1995) and total P via acid digestion followed by inductively coupled plasma-optical emissions spectroscopy (ICP-OES). For the P analyses, a nitric acid and hydrogen peroxide digest based on McQuaker et al. (1979) was carried out on most samples. However, insufficient mass of some samples (n = 36; primarily that of tiller shoot and panicle tissue) necessitated a nitric-perchloric digest based on Martinie and Schilt (1976). The P recovery of both digest methods on duplicate samples was determined and found to be similar. Concentrations of total N and P in plant samples were multiplied by the DM to determine plant N and P uptake (mg N and mg P per column).
The nylon bags were recovered from the soil following aboveground harvest. Soil was washed from the bags, with roots recovered, rinsed and stored in 30% ethanol solution prior to scanning. A flatbed scanner (Epson 12000XL, 600 dots per inch, Sydney, Australia) was used to determine root diameter and length within the band zone using WhinRhizo Pro 2020 software (Régent instruments, Québec, Canada). Root length density (RLD; cm cm−3) within the soil volume equivalent to the band zone was then calculated from root length and the volume of each bag (1785.71 cm3, based on 2.5 kg soil mass and 1.4 g cm−3 bulk density).
All data was transformed to be expressed as a percentage (%) of that achieved in the positive control (i.e. the uniform N, dispersed P treatment).
Statistical analyses
All statistical analyses were carried out using R statistical software (ver. 4.1.0, R Core Team 2021). Linear mixed effects models from the lme4 package (Bates et al. 2015) were used to compare treatment effects on biomass accumulation, RLD, and plant N and P uptake. The interactions between soil N distribution and P placement were assigned as fixed effects, and the incomplete replication across lysimeter tables was treated as a random effect. A repeated measures analyses of variance (ANOVAs) was carried out on all other temporal growth measures, with column ID assigned as a random effect, and the interactions between soil N distribution and P placement assigned as fixed effects.
All models were assessed for normality and homogeneity of variances using Shapiro-Wilk and Levene’s Tests, respectively. Where required, analyses were carried out using transformed data to ensure model assumptions were met. Pairwise comparisons were made using estimated marginal means with Tukey’s adjustments from the emmeans package (Lenth 2021).
Soil N concentration in the uniform N, dispersed P treatments (i.e. the positive controls) had no effect on biomass accumulation, N or P uptake (P > 0.05). Therefore, these treatments were combined to create one ‘positive control’ treatment where N was not limiting, referred to as such from hereafter.
Results
Biomass responses to soil N distribution
The concentration of N in deeper profile layers tended to reduce biomass production compared to when N was uniformly distributed or concentrated in the top 20 cm of the soil profile (Fig. 2). Compared to the positive control (uniformly distributed N and P), average biomass tended to decrease in response to an elevated N concentration within increasingly deeper soil layers. While this response was apparent across both P placements, it was much more substantial in the banded P (3, 22 and 26% reduction) than the dispersed P (4, 3 and 11% reduction) treatments with N concentrated in the top, middle and bottom layers, respectively (P > 0.05; Fig. 2).
Relative aboveground biomass (%) in sorghum grown with contrasting soil N and P distributions compared to the positive control (dispersed N and P; left-most column). Error bars, s.e.m (%). For further statistical details and the partitioning of biomass amongst aboveground plant structures, see Supplementary Table S1.
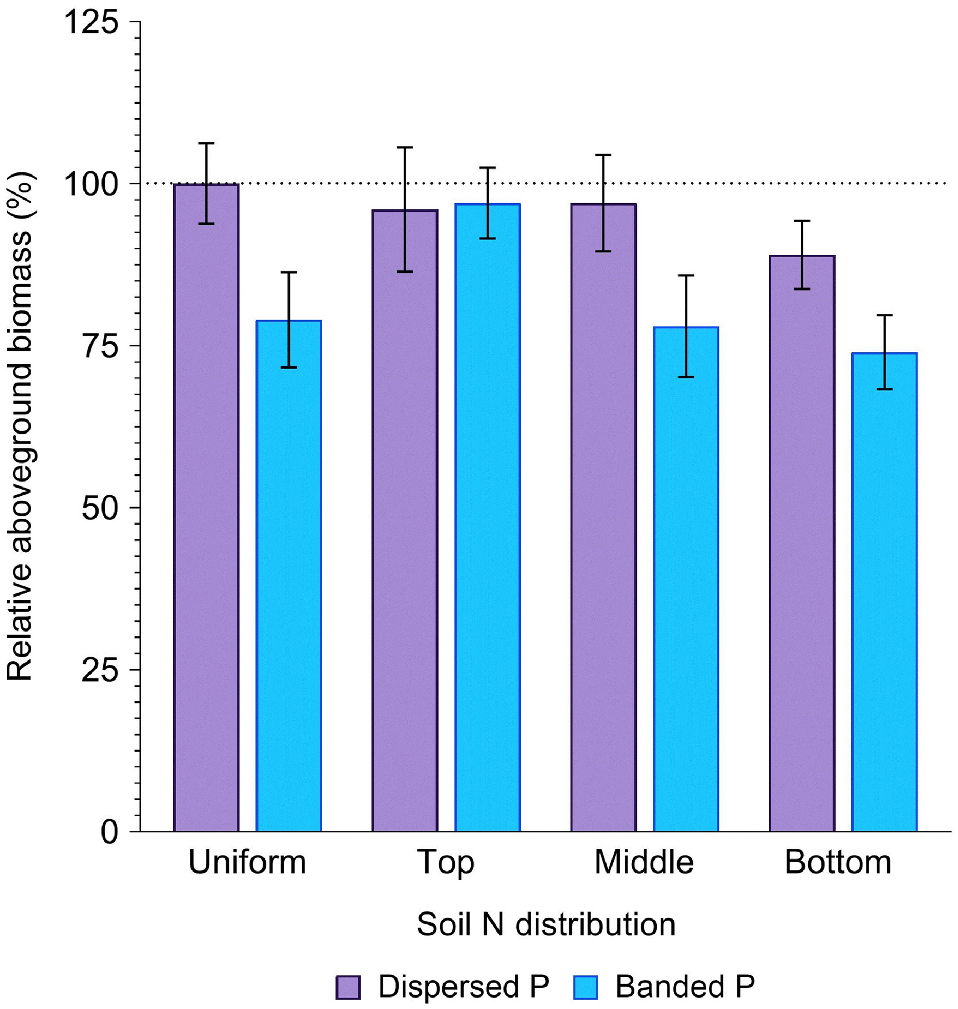
Biomass responses to P placement
Averaged across all soil N distributions, total aboveground biomass production in the dispersed P treatments (191.9 ± 6.9 g per pot) did not significantly differ from that produced by the positive control (202.3 ± 12.5 g per pot) (P = 0.89, Fig. 2). In contrast, biomass accumulation in the banded P treatments (165.7 ± 7.4 g per pot) was significantly (18.1%) less than the positive control (P = 0.01, Fig. 2). Some variability in the effect of P placement on biomass accumulation was evident depending on soil N distribution (P = 0.26). When N was uniformly distributed down the profile, banded P produced only 79% of the biomass as when P was also uniformly distributed in the positive control. However, when N was concentrated in the top 20 cm layer, biomass was comparable between the banded and dispersed P treatments (97% and 96% of the positive control for the banded and dispersed P, respectively). In contrast, when N was concentrated in deeper profile layers the banded P treatments produced only 78% (middle), or 74% (bottom) of the biomass in the positive control, compared to 97% (middle) and 89% (bottom) of the biomass in the positive control when P was dispersed (Fig. 2).
Tillering responses to N distribution and P placement
In general, tiller production was highly variable across the experiment (Fig. 3a). However, in the banded P treatments, the concentration of N in the top layer tended to produce more tillers compared to the positive control. This contrasts with all other soil N distributions, where banded P produced considerably less tiller biomass than the control (P > 0.05; Fig. 3a). Although variable, relative differences in tiller production with contrasting soil N distribution were less when P was dispersed (P > 0.05; Fig. 3a). In addition to differences in total tiller biomass, there were clear effects of N and P distribution on the formation of productive tillers (i.e. those that initiated a head and produced grain). When P was dispersed throughout the soil columns, concentration of N in the top, middle or bottom profile layers resulted in tiller panicle biomass that was only 5–25% of that in the positive control (P > 0.05; Fig. 3b). When P was banded, no panicles were produced on tillers when N was located in the middle and bottom profile layers, but when N was concentrated in the top 20 cm layer with the P bands, tiller panicle biomass was not significantly different to the positive control.
Relative aboveground tiller biomass (%) (a) and relative tiller panicle biomass (%) (b) in sorghum grown with contrasting soil N and P distributions compared to the positive control (dispersed N and P; left-most column). Error bars, s.e.m (%). Further statistical details and the partitioning of tiller biomass amongst shoots and panicles are in Table S1.
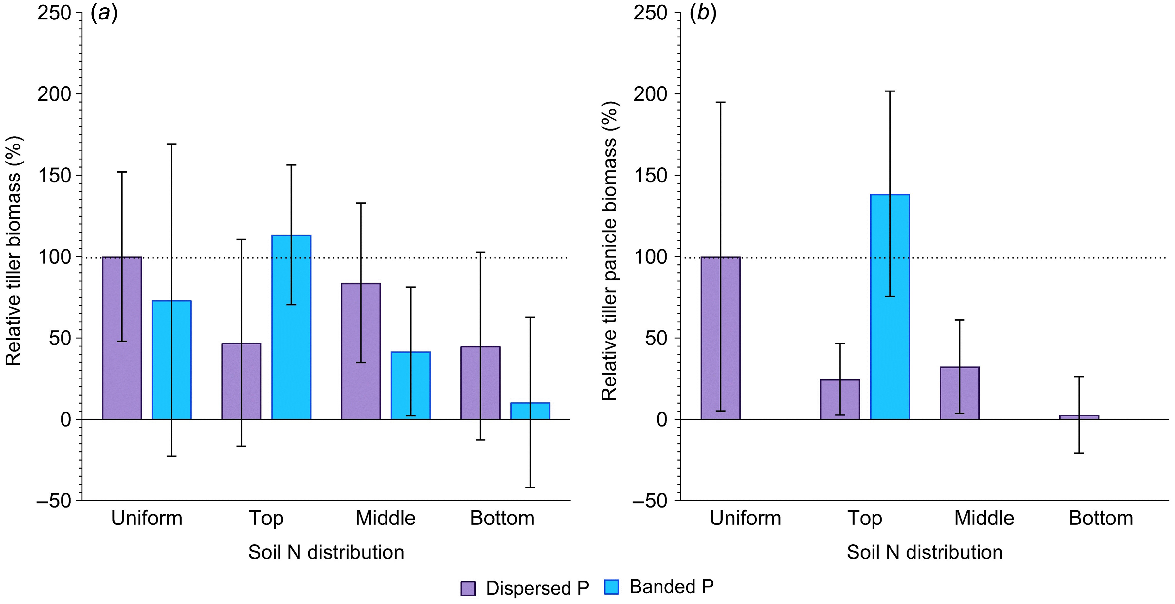
Plant P uptake with contrasting soil N distribution and P placement
Averaged across all soil N distributions, total P uptake in the dispersed P treatment (386.6 ± 20.7 mg P per pot) did not significantly differ from that in the positive control (440.1 ± 38.8 mg P per pot) (P = 0.84). In contrast, P uptake in the banded P treatments was much less (262.1 ± 16.6 mg P per pot), being only 60% of the positive control (P = 0.02, Fig. 4, Table S2). Averaged across both P placements, total P uptake was significantly less when N was concentrated in the bottom soil layer compared to all other distributions, resulting in only 61% of that achieved in the positive control (P = 0.04, Fig. 4). In the banded P treatments, plant P uptake was maximised when N was concentrated in the top layer (69% of the positive control), whilst plant P uptake was maximised in the dispersed P treatment when N was concentrated in the middle soil layer (98% of the positive control; Fig. 4).
Relative total P uptake (%) in sorghum grown with contrasting soil N and P distributions compared to the positive control (dispersed N and P; left-most column). Error bars, s.e.m (%). Asterisks denote treatments which significantly differ from the positive control (P < 0.05; l.s.d. = 34%). Further statistical details and the distribution of plant P amongst aboveground structures are in Table S2.
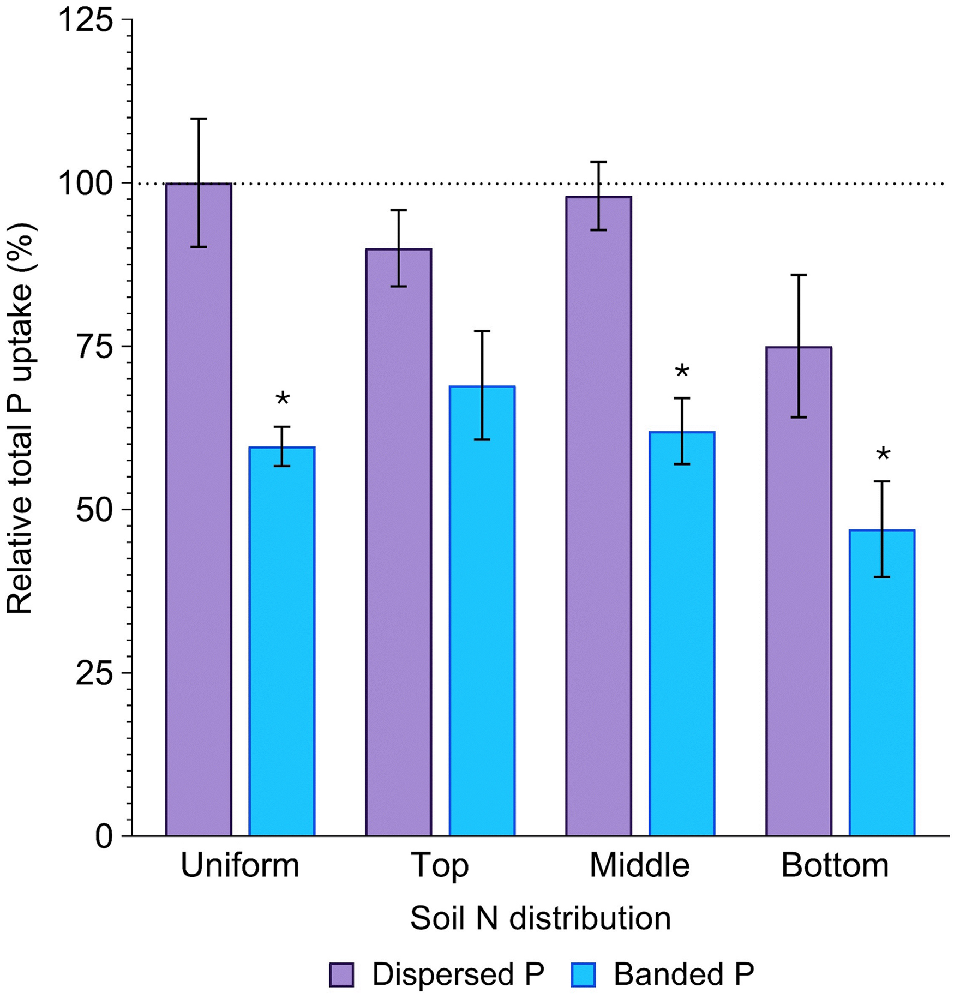
Plant N uptake with contrasting soil N distribution and P placement
Sorghum N uptake was significantly affected by P placement (P = 0.001). Averaged across all soil N distributions, N uptake in the dispersed P treatment (2578.8 ± 159.8 mg N per pot) did not significantly differ from that in the positive control (2750.2 ± 148.5 mg N per pot) (P = 0.95). In contrast, N uptake in the banded P treatment (2092.8 ± 115.2 mg N per pot) was 24% less than the positive control (P = 0.04), Fig. 5). However, N uptake was similar between the banded and dispersed P treatment when N was concentrated in the top layer (92 and 95% of the control, respectively). Total N uptake tended to decrease as the depth of the N enriched layer increased (94, 88 and 73% of the positive control with top, middle and bottom N enriched layers, respectively), with this increase more substantial in the banded P treatment (92, 73 and 62% of the positive control) than the dispersed P treatment (95, 103 and 84% of the positive control).
Relative total N uptake (%) in sorghum grown with contrasting soil N and P distributions compared to the positive control (dispersed N and P; left-most column). Error bars, s.e.m (%). Asterisks denote treatments which significantly differ from the positive control (P < 0.05; l.s.d. = 29%). Further statistical details and the distribution of plant N amongst aboveground structures are in Table S3.
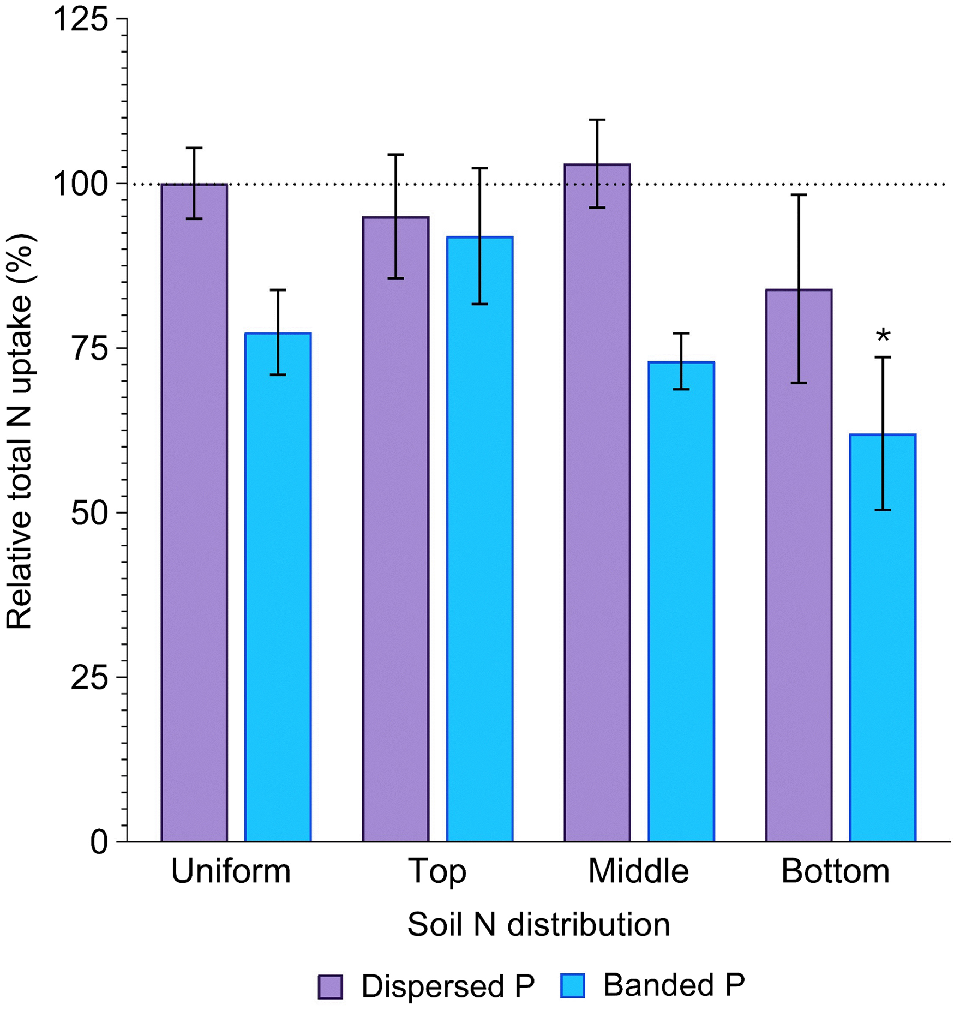
Root length density (RLD) in the band zone
Root length density in the band zone (cm cm−3) was affected by both P placement and soil N distribution (P = 0.03; P = 0.04; Fig. 6), with evidence that the extent of root proliferation in the deep P band was affected by soil N distribution. Differences in RLD in the band zone with contrasting soil N distribution were much more pronounced in the banded P treatment compared to the dispersed P treatment. Relative to the positive control, the banded P treatment produced 219, 420, 9, and 63% greater RLD in the band zone when N was uniformly distributed, concentrated in the top, middle and bottom layers, respectively, although this increase was only significant (P = 0.02) when N was concentrated in the top layer (Fig. 6). This contrasts with the dispersed P treatment, where RLD in the same part of the soil column was 95, 45 and 53% greater than the positive control (P > 0.05). Moreover, the relative difference in RLD with P placement varied with soil N distribution. When N was dispersed throughout the entire soil volume, RLD in the band zone of the banded P treatment was 219% greater than the positive control. However, when N was concentrated in the middle or bottom soil layers, RLD at the depth of the fertiliser band was more comparable between dispersed and banded P treatments (45 and 9% greater when N was concentrated in the middle soil layer, respectively; and 53 and 63% greater when N was concentrated in the bottom soil layer, respectively). In contrast, when N was concentrated in the topsoil layer, RLD at the band depth was considerably greater when P was banded compared to dispersed (420 and 95% greater than the control, respectively; Fig. 6).
Relative RLD (%) in the P band zone of sorghum grown with contrasting soil N and P distributions compared to the positive control (dispersed N and P; left-most column). Error bars, s.e.m (%). Asterisks denote treatments which significantly differ from the positive control (P < 0.05; l.s.d. = 417%).
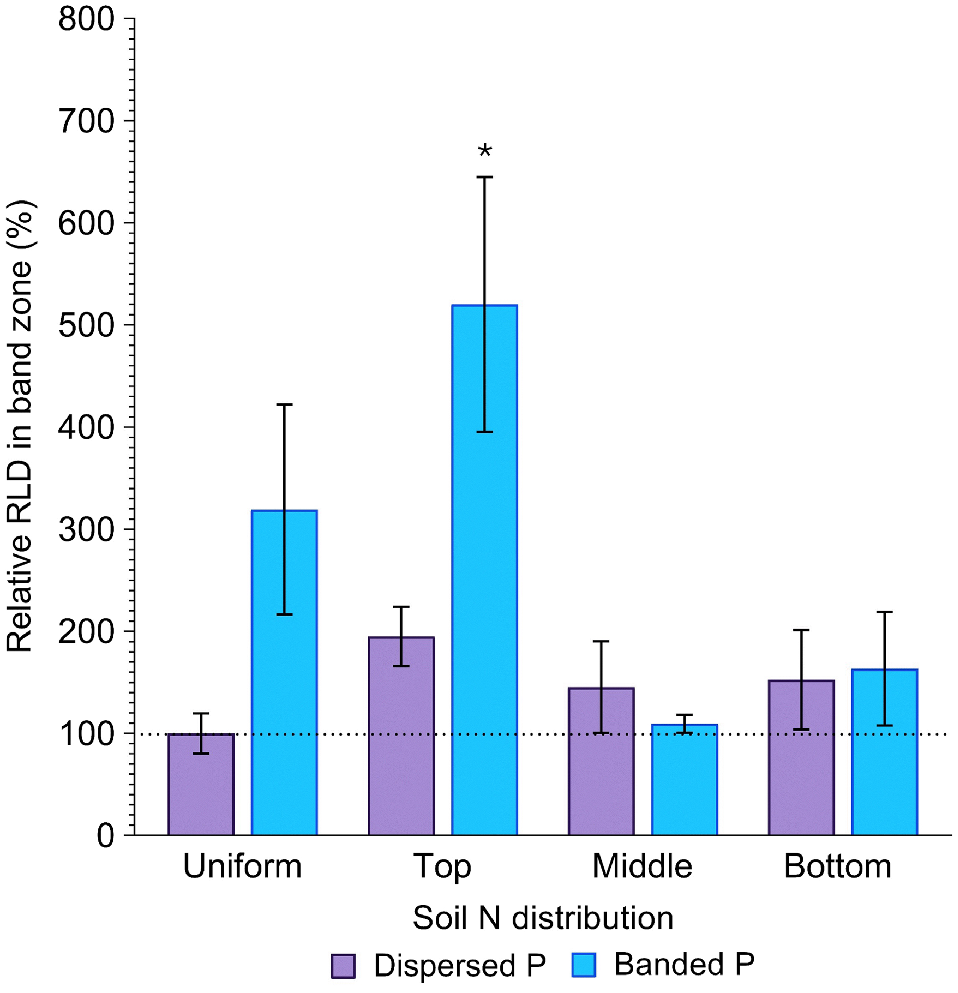
Discussion
Co-location of N and P in shallow soil layers can optimise sorghum growth responses to banded P
This experiment demonstrates that the placement of banded P in N enriched soil ensures that both nutrients are highly accessible during early growth and tiller initiation, and is likely to optimise sorghum growth responses to P applications. This is demonstrated by similar biomass production (cf. the positive control) in the banded P treatment when N was concentrated in the top soil layer, despite P banding resulting in considerably less biomass production (cf. the positive control) with all other soil N distributions.
While it is well understood that P supply during early growth is critical to maximise potential grain number (Grant et al. 2001), and that some delay in plant access to a deep P band is probable, starter P is likely to have provided a critical interim P source (van der Bom et al. 2023b). Once plant roots were established, they were able to exploit P in the deep band (i.e. later in growth) sustaining main stem and tiller development once plant demands had exceeded what the starter P could provide (van der Bom et al. (2023b). Consequently, when N was concentrated in the top layer, plant access to soil of high N and P concentrations during early growth was achieved, maximising growth responses to the deep P band (located near the bottom of that same top soil layer) compared to when N was concentrated deeper in the column (Figs 2, 3 and 6).
Although the total plant supply of P was greater in the dispersed P treatment than the banded P treatment (3.6 vs 0.6 g P per column), its distribution throughout the entire column resulted in an overall lower concentration of soil P relative to that within the deep P band. Similarly, the dispersed N treatment did not result in highly concentrated zones of elevated soil N concentration, while the N enriched layers provided diffuse zones of higher N status than the uniform treatment. In particular, the N-enriched layers situated in the middle and bottom thirds of the column likely supplied this additional N later in growth.
The benefit to plant growth from the co-location of N and P in shallow soil layers observed in the current experiment is consistent with previous studies that reported that the combined application of starter N and P provided a synergistic effect, consistently increasing maize and sorghum yields compared to when either nutrient was applied alone under field conditions (Blandino et al. 2022; Gordon and Whitney 1995). Although those studies focused on bands of N and P placed near the seed (cf. the present experiment involving discrete layers of N enriched soil and a deep P band), they highlight a similar relationship between early crop access to soil of high N and P concentrations, and the resulting positive effects on plant growth.
While the benefit of plant access to additional N early in plant development has been identified across numerous studies, the extent of this effect is probably influenced by overall soil N content (Sharma and Bali 2018). In this study, the NO3-N content of the background soil was moderate (46.5 mg NO3-N kg soil−1; Table 1). While plant uptake from background N was not directly quantified, it would definitely have contributed to total N uptake. This may have lessened the effects of the N enriched layer on P responsiveness, which suggests that this interaction may be stronger than demonstrated in this study.
The continued supply of N from a large soil volume (i.e. high background N) will help to support plant N demands under field conditions. The automated irrigation system used in the current experiment ensured plant access to N concentrated in shallow soil layers was maintained, which is not generally reflective of cropping systems in north-eastern Australia. In this environment, the high evaporative demands and sporadic rainfall patterns typical of this region mean that these systems are largely reliant on water and nutrients accumulated in subsoil layers, rather than on in-season rainfall and nutrients situated in the topsoil (Singh et al. 2005; Angus et al. 2020). Therefore, while this study has demonstrated that N concentrated in the topsoil layer may be required to optimise deep P responses, it is important to recognise that soil N located deeper in the soil profile is an important source of plant N in field conditions. Consequently, for deep P responses to be optimised, it can be hypothesised that at least some N needs to be concentrated in shallow soil layers for early plant access, while the remainder should be distributed through the subsoil to synchronise the supply of N and water during the growing season. The optimal distribution of N is likely to vary with seasonal conditions, and is worthy of further investigation.
N and P are required early and in combination to maximise tiller development
Tillering responses were highly variable across the experiment (Fig. 3a), although the general trends for banded P were reasonably consistent with those observed in total aboveground biomass production (Fig. 2). This provides support to the hypothesis that co-location of N and P enriched soil in shallow layers may maximise sorghum responses to P. Furthermore, the exclusive incidence of grain bearing tillers in the banded P treatment with N concentrated in the top layer (Fig. 3b) suggests that the co-location of both nutrients in the uppermost layer was required to support productive tillering. Improved P nutrition is a known mechanism by which fertile tiller production is enhanced, increasing cereal yields (Chen et al. 2019), and together with this study, highlights the importance of early plant access to N and P enriched soil to maximise P responses. Moreover, the finite period when tillers are initiated likely places further importance on early plant access to both N and P. Most tillers in the present experiment were produced within the first 28 DAS (Supplementary Fig. S1), and although the timing of plant access to the N enriched layers situated deeper in the soil profile was not quantified in this study, it is likely that root activity, and therefore nutrient acquisition, was concentrated in the uppermost soil layer for much of this time (Hauggaard-Nielsen et al. 2001).
While varying tillering production was observed in this study, further research could investigate genotypic variation for sorghum tillering in response to altered N and P placement. Similarly, investigations on species such as wheat (Triticum aestivum) and barley (Hordeum vulgare), which are prolific tillering crops (Hammer et al. 2023), would be likely to provide further insight into the effects of temporal N and P supply on tiller initiation and fertility in the context of deep P responses.
Banded P responses may be affected by localised root interactions with the band zone
The increase in deep P response when co-located with N enriched soil may be explained by several factors: (1) potential interactions between soil N concentration in and around the band zone; (2) localised root responses to the band; and (3) the subsequent ability of plants to acquire this P. While biomass accumulation was only measured at physiological maturity in the current experiment, it is likely that the co-location of concentrated N and P in the uppermost soil layer promoted increased early vigour compared to when concentrated P was found deeper in the soil profile (Ma et al. 2013; Blandino et al. 2022). This may have enhanced initial root growth, better facilitating localised root responses to the deep P band compared to when N was concentrated in deeper soil layers (Ma et al. 2013). Furthermore, potential increases in tiller production with early access to high concentrations of both N and P may have promoted additional root growth, including within the deep P band. While this has not been directly reported for sorghum, such relationships between increased tillering and root growth are evident in other fibrous root species including sugarcane (Matsuoka and Garcia 2011) and wheat (Wang and Below 1992).
It is widely understood that high concentrations of NH4+ within P bands can enhance root proliferation within the band zone e.g. (Jing et al. 2010; Jing et al. 2012; Ma et al. 2013). This relationship is largely attributed to decreases in rhizosphere pH and a consequent increase in P mobilisation arising from plant uptake of NH4+ (Jing et al. 2010). However, the relative effect of additional NH4+ on P availability and accompanying root responses will also be influenced by soil chemical characteristics and the form of P applied (Meyer et al. 2021). In this study, the alkaline experimental soil may support increased P availability in response to a decreasing soil pH within the acidified zones of MAP bands (Meyer et al. 2020; 2021). Thus, while the additional NH4+ situated within the deep P band when co-located with the N enriched layer may have mobilised additional P to further enhance root proliferation within this zone, the extent of its effect on P availability remains unclear.
The lack of strong localised root responses to the deep P band in the absence of highly N enriched soil are inconsistent with the understanding that cereal roots generally exhibit very clear morphological responses to P enriched zones of soil (e.g. van der Bom et al. 2023b) and requires further investigation. Nonetheless, this lack of localised root response to the P band does highlight trade-offs between application of a small volume of highly P enriched soil (i.e. a fertiliser band) with that of P application to a large soil volume at a lower P concentration. While the banding of P likely reduced P fixation by limiting soil-fertiliser contact (Meyer et al. 2020), this appeared insufficient to compensate for the overall reduction in P supply and root P interception relative to when more P was accessible throughout the entire soil volume in the absence of early N access and clear root proliferation in the band zone. Although the banded P was unable to maximise growth except for when situated in N-enriched soil, the absence of clear phasic delays and other P deficiency symptoms in the banded P treatment suggest that this placement strategy still enabled plants to avoid severe P limitation. Plant P uptake remained greater in the dispersed P treatment regardless of soil N distribution (Fig. 4), further suggesting that banded P was unable to meet P demand under optimal conditions and/or higher P demands that characterised this study. This is also reflected in tissue P concentrations, which averaged 0.16% in the banded P treatment compared to 0.21% in the dispersed P treatment (data not shown). This further shows that the dispersed P treatment was better able to alleviate P constraints compared to when P was applied as a band, achieving tissue P concentration around the critical value of 0.2% (Jones 1983). Despite the very low P status of the unamended soil (Colwell P 6 mg P kg−1; Table 1), the placement of starter and deep P bands proved to be reasonably effective at reducing the incidence of P deficiencies, even though they were unable to completely saturate plant P demand.
Conclusion
This experiment has provided evidence that the ability for sorghum to respond to P fertiliser can be affected by the concentration and spatial distribution of soil N. When deep P bands were applied to soil of moderate initial N fertility, aboveground biomass, plant N and P uptake were all maximised when additional N was concentrated in the 0–20 cm soil layer. While plant P uptake remained lower in the banded treatment regardless of soil N distribution, the concentration of N in the uppermost soil layer supported comparable biomass accumulation in the banded P treatment compared to when P was dispersed throughout the soil profile. This was likely influenced by differences in localised root responses to the deep P bands, depending on the timing of N access and soil N concentration, with more extensive root proliferation evident within the band zone when co-located with N enriched soil compared to when this N was dispersed throughout or concentrated deeper in the soil profile. Therefore, growers should ensure that adequate N is situated in upper soil layers to optimise plant responses and utilisation of banded P fertilisers.
Data availability
The data that support this study will be shared upon reasonable request to the corresponding author.
Declaration of funding
We acknowledge the financial support provided by an Australian Government Research Training Program (RTP) Scholarship.
Acknowledgements
We thank Tim Rossignol, Bec Archer, Melissa Schunemann, and Celso Cordova for their technical support throughout the experiment. We also thank Richard Sulman and Paul Kamel from Biosystems Engineering Pty Ltd. for their ongoing support using the lysimeter platform.
References
Angus J, Bell M, McBeath T, Scanlan C (2020) Nutrient-management challenges and opportunities in conservation agriculture. In ‘Australian agriculture in 2020: from conservation to automation’. (Eds J Pratley, J Kirkegaard) pp. 221–237. (Agronomy Australia and Charles Sturt University: Wagga Wagga, NSW, Australia)
Bates D, Mächler M, Bolker B, Walker S (2015) Fitting linear mixed-effects models using lme4. Journal of Statistical Software 67(1), 1-48.
| Crossref | Google Scholar |
Blair GJ, Chinoim N, Lefroy RDB, Anderson GC, Crocker GJ (1991) A soil sulfur test for pastures and crops. Australian Journal of Soil Research 29(5), 619-626.
| Crossref | Google Scholar |
Blandino M, Battisti M, Vanara F, Reyneri A (2022) The synergistic effect of nitrogen and phosphorus starter fertilization sub-surface banded at sowing on the early vigor, grain yield and quality of maize. European Journal of Agronomy 137, 126509.
| Crossref | Google Scholar |
Burkitt LL, Moody PW, Gourley CJP, Hannah MC (2002) A simple phosphorus buffering index for Australian soils. Australian Journal of Soil Research 40(3), 497-513.
| Crossref | Google Scholar |
Chauhan YS, Rachaputi RCN (2014) Defining agro-ecological regions for field crops in variable target production environments: a case study on mungbean in the northern grains region of Australia. Agricultural and Forest Meteorology 194, 207-217.
| Crossref | Google Scholar |
Chen X-X, Zhang W, Liang X-Y, Liu Y-M, Xu S-J, Zhao Q-Y, Du Y-F, Zhang L, Chen X-P, Zou C-Q (2019) Physiological and developmental traits associated with the grain yield of winter wheat as affected by phosphorus fertilizer management. Scientific Reports 9, 16580.
| Crossref | Google Scholar | PubMed |
Colwell JD (1963) The estimation of the phosphorus fertilizer requirements of wheat in southern New South Wales by soil analysis. Australian Journal of Experimental Agriculture 3(10), 190-197.
| Crossref | Google Scholar |
Colwell J, Esdaile R (1968) The calibration, interpretation, and evaluation of tests for the phosphorus fertilizer requirements of wheat in Northern New South Wales. Soil Research 6, 105-120.
| Crossref | Google Scholar |
Gordon WB, Whitney DA (1995) No-tillage grain sorghum response to starter nitrogen-phosphorus combinations. Journal of Production Agriculture 8(3), 369-373.
| Crossref | Google Scholar |
Grant CA, Flaten DN, Tomasiewicz DJ, Sheppard SC (2001) The importance of early season phosphorus nutrition. Canadian Journal of Plant Science 81(2), 211-224.
| Crossref | Google Scholar |
Hammer GL, McLean G, Kholová J, van Oosterom E (2023) Modelling the dynamics and phenotypic consequences of tiller outgrowth and cessation in sorghum. In Silico Plants 5(2), diad019.
| Crossref | Google Scholar |
Hauggaard-Nielsen H, Ambus P, Jensen ES (2001) Temporal and spatial distribution of roots and competition for nitrogen in pea-barley intercrops – a field study employing 32P technique. Plant and Soil 236, 63-74.
| Crossref | Google Scholar |
Jing J, Rui Y, Zhang F, Rengel Z, Shen J (2010) Localized application of phosphorus and ammonium improves growth of maize seedlings by stimulating root proliferation and rhizosphere acidification. Field Crops Research 119(2–3), 355-364.
| Crossref | Google Scholar |
Jing J, Zhang F, Rengel Z, Shen J (2012) Localized fertilization with P plus N elicits an ammonium-dependent enhancement of maize root growth and nutrient uptake. Field Crops Research 133, 176-185.
| Crossref | Google Scholar |
Jones CA (1983) A survey of the variability in tissue nitrogen and phosphorus concentrations in maize and grain sorghum. Field Crops Research 6, 133-147.
| Crossref | Google Scholar |
Lenth RV (2021) Emmeans: estimated marginal means, aka least-squares means. Available at https://rvlenth.github.io/emmeans/
Lindsay WL, Norvell WA (1978) Development of a DTPA soil test for zinc, iron, manganese, and copper. Soil Science Society of America Journal 42(3), 421-428.
| Crossref | Google Scholar |
Ma Q, Rengel Z, Rose T (2009) The effectiveness of deep placement of fertilisers is determined by crop species and edaphic conditions in Mediterranean-type environments: a review. Australian Journal of Soil Research 47(1), 19-32.
| Crossref | Google Scholar |
Ma Q, Zhang F, Rengel Z, Shen J (2013) Localized application of NH₄⁺-N plus P at the seedling and later growth stages enhances nutrient uptake and maize yield by inducing lateral root proliferation. Plant and Soil 372, 65-80.
| Crossref | Google Scholar |
Martinie GD, Schilt AA (1976) Wet oxidation efficiencies of perchloric acid mixtures for various organic substances and the identities of residual matter. Analytical Chemistry 48(1), 70-74.
| Crossref | Google Scholar |
Matejovic I (1995) Total nitrogen in plant material determinated by means of dry combustion: a possible alternative to determination by Kjeldahl digestion. Communications in Soil Science and Plant Analysis 26, 13-14 2217-2229.
| Crossref | Google Scholar |
Matsuoka S, Garcia AAF (2011) Sugarcane underground organs: going deep for sustainable production. Tropical Plant Biology 4, 22-30.
| Crossref | Google Scholar |
McKenna BA, Kopittke PM, Bell MJ, Lombi E, Klysubun W, McLaren TI, Doolette CL, Meyer G (2024) Phosphorus availability and speciation in the fertosphere of three soils over 12 months. Geoderma 447, 116913.
| Crossref | Google Scholar |
McQuaker NR, Brown DF, Kluckner PD (1979) Digestion of environmental materials for analysis by inductively coupled plasma-atomic emission spectrometry. Analytical Chemistry 51(7), 1082-1084.
| Crossref | Google Scholar |
Meyer G, Bell MJ, Doolette CL, Brunetti G, Zhang Y, Lombi E, Kopittke PM (2020) Plant-available phosphorus in highly concentrated fertilizer bands: effects of soil type, phosphorus form, and coapplied potassium. Journal of Agricultural and Food Chemistry 68(29), 7571-7580.
| Crossref | Google Scholar | PubMed |
Meyer G, Bell MJ, Lombi E, Doolette CL, Brunetti G, Novotny EH, Klysubun W, Zhang Y, Kopittke PM (2021) Phosphorus speciation in the fertosphere of highly concentrated fertilizer bands. Geoderma 403, 115208.
| Crossref | Google Scholar |
Raymond N, Kopittke PM, Wang E, Lester D, Bell MJ (2021) Does the APSIM model capture soil phosphorus dynamics? A case study with Vertisols. Field Crops Research 273, 108302.
| Crossref | Google Scholar |
Raymond NS, Kopittke PM, van der Bom FJT, Barrow NJ, Bell MJ (2023) Short-term phosphorus sorption and desorption in contrasting cropped Vertisols. European Journal of Soil Science 74(5), e13418.
| Crossref | Google Scholar |
Sharma LK, Bali SK (2018) A review of methods to improve nitrogen use efficiency in agriculture. Sustainability 10(1), 51.
| Crossref | Google Scholar |
Singh DK, Sale PWG, Routley RR (2005) Increasing phosphorus supply in subsurface soil in northern Australia: rationale for deep placement and the effects with various crops. Plant and Soil 269, 35-44.
| Crossref | Google Scholar |
van der Bom FJT, Williams A, Raymond N, Sulman R, McLean G, Bell M (2023a) Spatio-temporal distribution of water and phosphorus determine growth of sorghum genotypes with contrasting nodal root angle. Plant and Soil 499, 37-54.
| Crossref | Google Scholar |
van der Bom FJT, Williams A, Borrell AK, Raymond N, Bell MJ (2023b) Phosphorus management is key to effective deployment of root ideotypes in complex soil environments. Plant and Soil 489, 323-340.
| Crossref | Google Scholar |
Walkley A, Black IA (1934) An examination of the degtjareff method for determining soil organic matter, and a proposed modification of the chromic acid titration method. Soil Science 37(1), 29-38.
| Crossref | Google Scholar |
Wang X, Below FE (1992) Root growth, nitrogen uptake, and tillering of wheat induced by mixed-nitrogen source. Crop Science 32(4), 997-1002.
| Crossref | Google Scholar |
Wang X, Lester DW, Guppy CN, Lockwood PV, Tang C (2007) Changes in phosphorus fractions at various soil depths following long-term P fertiliser application on a Black Vertosol from south-eastern Queensland. Australian Journal of Soil Research 45(7), 524-532.
| Crossref | Google Scholar |
Yang Z, Hammer G, Van Oosterom E, Rochas D, Deifel K (2010) Effects of pot size on growth of maize and sorghum plants. In ‘1st Australian summer grains conference’, 21–24 June 2010, Gold Coast, Queensland, Australia. (Eds B George-Jaeggli, D Jordan) pp. 1–7. (Grains Research and Development Corporation: Canberra, Australia)
Youngquist JB, Maranville JW (1992) Patterns of nitrogen mobilization in grain sorghum hybrids and the relationship to grain and dry matter production. Journal of Plant Nutrition 15, 445-455.
| Crossref | Google Scholar |