Self-assembled monolayers: a journey from fundamental tools for understanding interfaces to commercial sensing technologies
Essam M. Dief A , Richard D. Tilley A and J. Justin Gooding
A
Abstract
Self-assembled monolayers were first described in the 1980s and have now become ubiquitous in many interfacial technologies. In this account, we discuss different self-assembled monolayer systems, outlining their positives and negatives. We then overview other researchers’ work and our own group’s journey in using self-assembled monolayers to develop new concepts in sensing and addressing general challenges faced by many types of sensors. Finally, we reflect on some of the challenges monolayer chemistry needs to address to facilitate further use of this powerful surface chemistry in commercial devices.
Keywords: continuous monitoring, DNA devices, electrochemical aptamer-based sensors, electrochemical biosensors, electrode functionalisation, molecular devices, molecular interfaces, self-assembled monolayers, single-molecule sensor.
Introduction
Self-assembled monolayers (SAMs) are, as the name suggests, monomolecular-thick films that form spontaneously on a surface under the right conditions.1 The spontaneous formation arises from the head group of a surface-active molecule having a high affinity for the surface. The higher the affinity of the headgroup for the surface, nominally the more stable the monolayer system. The distal chemistry of the SAM-forming molecules then defines the chemical characteristics of the surface and how that material interacts with the environment around it. For example, if the distal moieties are hydrophobic and the monolayer is well packed, then the surface will appear hydrophobic, whereas if the distal moieties are hydrophilic, it will appear hydrophilic. As they are a single molecule thick, the bulk characteristics of the material modified can still be utilised in many instances, which is highly advantageous. That is, if the surface is transparent, that transparency will be maintained or if it is conducting, the bulk conductivity will be maintained, and if it is an electrode, it may still even allow electron transfer through the monolayer to the electrode depending on the monolayer characteristics.
SAMs were first described in 1980 when Sagiv2 showed that chloro- and alkoxy-silanes reacted with hydroxyl-terminated surfaces such as glass, silica and metal oxides to afford a covalently bound molecular layer. Almost straight after, in 1983, Nuzzo and Allara reported alkanethiol SAMs on gold surfaces, where a pseudo-covalent bond is formed between the thiol headgroup and the gold surface.3 Following soon after was a variety of other monolayer, or monolayer-like, systems such as aryl diazonium salts on a vast array of surfaces in 1992,4 alkenes and alkynes on hydrogen-terminated silicon surfaces in 1993,5 and organophosphonates and alkanecarboxylic acids on oxide surfaces.6–8 In the space of a few short years, we had an array of monolayer systems for almost any application from the fundamental understanding of surface processes9,10 and dynamic surfaces11,12 to applications in optoelectronic devices,13,14 electrochemistry,15 cell biology16 and so much more.
The intense interest in SAMs comes from the capability to provide something akin to molecular-level control over the modification of a surface. This is because multiple different chemical species can be incorporated into the same SAM simply by having multiple different species in the solution from which the SAM is assembled. By altering the ratios of species in solution, significant control over the ratio of the different species on the surface can be achieved. This control then allows multifunctional interfaces to be prepared.17 In sensing, for example, this then allows the spacing of receptors; the diluting component (the diluent) may also provide resistance to non-specific adsorption of species, the diluent could be used to orient receptors or multiple different receptors could be added to the same interface. In one example of an ion-channel biosensor, there are in fact more than 10 different molecular components in the sensing interface.18 The power of the molecular control is not limited to just lateral control. Different-length SAM-forming molecules can be used to create well-defined spacings between the surface and a solution as is done in electron transfer studies.19 Furthermore, rigid molecules can be used to provide vertical control of individual molecules from a surface.20
In this account, we first compare and contrast the most common monolayer systems through our own experience with them; we then discuss how we used SAMs to make better-performing or completely new types of sensors and the issues related to the different monolayer systems. We discuss the fundamental surface chemistry and the challenges for such sensors to go from concept to being translated into commercial devices.
Self-assembled monolayer systems: their positives and negatives
The five SAM-forming systems we have used most commonly are the organosilane system, the alkanethiol system, aryl diazonium salts-derived layers, the hydrosilylation of alkenes and alkynes at silicon surfaces, and organophosphonates on indium tin oxide. Each of these systems is shown in Fig. 1 and they have their positives and negatives.17 We discuss each of these in turn.
Schematic depicting the different surface chemistry approaches that are commonly utilised to form monolayers on different electrode substrates, namely alkanethiols on gold spontaneous assembly, aryldiazonium salts on carbon materials by reductive adsorption, phosphonates on metal oxides (e.g. indium tin oxide, ITO) and unsaturated hydrocarbons (alkynes and alkenes) on oxide-free silicon by heat-assisted methods, and organosilane layers on glass by solvent-assisted approaches.
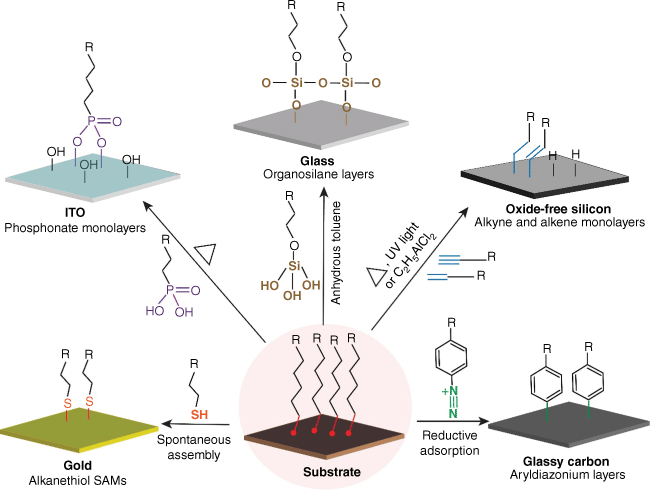
The organosilanes as depicted in Fig. 1 are an idealised view of how such a monolayer might look. The incredible advantage of organosilanes is that they are ideal for modifying silica surfaces, including glass, and changing the character of this surface or providing a platform for further functionalisation. This makes them very compatible with a whole range of optical applications including sensors and microscopy. The challenges with this surface chemistry are, however, extensive. The most apparent is that the idealised scheme probably seldom matches reality, as suggested in the literature.17 In many cases, the organosilane can be a multilayer with only a few points where the layer is coupled to the glass surface. This then has implications for the stability of such layers as the silico-oxygen bond is prone to hydrolysis in water, the medium relevant to most applications of this surface chemistry. This stability issue can limit the utility of this surface chemistry for applications where long-term exposure to water is required. That said, organosilane chemistry is still the most used surface chemistry for oxide-based surfaces because at present we do not have a better alternative.
The other widely used SAM chemistry is alkanethiol chemistry on noble metals, especially gold. In common with the organosilane system, it is very simple to make a monolayer, but as with organosilane chemistry, it is challenging to prepare a high-quality monolayer. That said, the alkanethiol SAMs are invariably monolayers. The power of this surface chemistry system is immense with regards to spatial control. For example, we have investigated how a surface affects the electrochemistry of a redox species anchored near that surface where we were able to alter the spatial separation between a surface and a redox species by a single carbon bond at a time, that is 0.11 nm,21,22 using rigid SAM-forming molecules. Alkanethiol SAMs have been used for a myriad of applications, both electrochemical and optical, where multiple different chemical species can be incorporated into the same layer. The greatest number of different species in an alkanethiol-based sensing layer that we are aware of is 17. Combine this with the huge amount of research into characterising and understanding these layers10 and we have close to the perfect SAM system. Close to because there are challenges in terms of stability of these layers. The stability revolves around the purported pseudo-covalent bond between the sulfur and gold. This bond has a strength of ~170 kJ mol−1.23 The stability challenge is two-fold. In electrochemical devices, this then relates to the electrochemical stability, where potentials more negative than −0.7 V versus Ag/AgCl (3 M KCl) start to show significant layers desorption and equally, potentials more positive than say +0.6 V (at pH 7) also start to show desorption.24 The other stability aspect is the long-term stability of the gold–thiolate bond as the thiol tends to oxidise over time to a sulfonate, which then binds to the gold much less strongly, and the monolayer starts to desorb.25
The quest for more stable monolayers saw our group start exploring newer systems. The first system we explored next was the aryl diazonium salt system (Fig. 2), where electrochemical potential is typically used to form a covalent bond between the phenyl ring and the underlying substrate. Initially, the approach was used for modifying carbon surfaces4 but later, it was shown that the approach could work for metals and conducting oxide surfaces. The power of this system is first, the bonds to the substrate are typically very strong, although differences exist between different substrate types, and second, that many different materials can be modified with the same surface chemistry. The disadvantages include the fact that commonly, these layers were often multilayers and seldom monolayers, the materials needed to be connected to an electrochemical circuit for the modification of a substrate, and the structure of the layers or the bonds formed with the substrate were not always well understood. In the case using aryl diazonium salt, a number of approaches have been developed to ensure monolayer formation. Furthermore, formation of the layers spontaneously on a variety of materials has also removed the need for connection of the substrate to a circuit. More work is needed to understand if ordered layers similar in control to the alkanethiol system can be achieved. This is important for sensing26 because to make multifunctional interfaces is still challenging although mixed layers have been developed.27,28
(a) Schematic of the Cu2+ sensing approach and mechanism using a glycine-glycine-histidine peptide-modified gold electrode. (b) Square wave voltammetry response of the copper sensor with the peak current increasing on addition of different concentrations of copper. (c) A calibration curve derived from the square wave voltammograms obtained at different CuII concentrations. Fig. 2 is adapted from Yang et al.,29 with permission from the Royal Society of Chemistry.
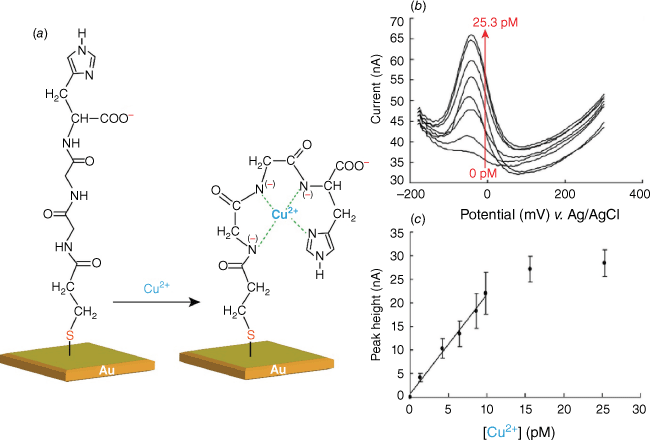
Perhaps even more stable than the aryl diazonium salt layers are SAMs formed by the hydrosilylation reaction between hydrogen-terminated silicon and SAM-forming molecules bearing a terminal alkene or alkyne. The stability of these layers is such that placing the substrates in hot boiling acids will not destroy the Si–C bond that forms. These were first developed by Chidsey and colleagues,30 and we showed with molecules bearing an alkyne at each end that such well-packed layers are formed that silicon surfaces modified with bisalkynes could be used as electrodes in aqueous solution without oxidation of the underlying silicon. Similarly to alkanethiols, the layers themselves can give exceptionally high spatial control with multiple functionalities incorporated within. The control was demonstrated by us when we used these layers to form electrically switchable surfaces to support cell adhesion. In this system, a potential was used to change the surface orientation of adsorption-resistant cells that hide or reveal cell coupling points31 or capture and release cells.32,33 The main drawback of this chemistry is that although the monolayers are stable, the underlying silicon substrate is highly unstable as it tends to oxidise, which of course changes its electrical behaviour. This places incredible demands on the monolayer system to protect the silicon, which is exceedingly challenging to achieve. Furthermore, formation of the SAMs requires considerable energy, usually as heat, to form in an inert atmosphere. The challenges in forming these SAMs mean that, more than likely, they are only viable for niche, high-value commercial applications.
The final SAM forming system we discuss is the organophosphonate SAM-forming molecules that are used to modify oxide surfaces, particularly indium tin oxide (ITO) or fluorine tin oxide. The SAM formation with surface chemistry involves an initial hydrogen bonding interaction followed by thermal treatment to dehydrate the surface and form a strong covalent bond, as shown in Fig. 1.34,35 The advantages of this monolayer system include it unambiguously being a monolayer, the layers being very stable and it being compatible with transparent surfaces, which allows microscopy and electrochemistry.36,37 Challenges include that the quality of the layer formed varies significantly depending on the surface structure of the ITO35 and the solvent used,38 the layers are nowhere near as well understood as alkanethiols on gold and, like with the hydrosilylation of alkenes, instability arises from the underlying substrate as distinct from the monolayer systems. This last statement pertains to the fact that ITO surfaces are only stable between −0.8 and +0.8 V and prone to dissolution if the pH drops below 6.39
Beyond the five SAM-forming chemistries discussed herein, there are two emerging SAM-forming systems that have not been extensively explored yet. One is the N-heterocyclic carbene system popularised by Crudden and co-workers,40 which binds to gold, and they argue is more stable than alkanethiols. The second is the just-reported non-covalent SAMs formed on multiple different electrode materials involving an electrostatic bond between a trimethyl ammonium derivative and the electrode. The authors state the system has a very wide potential range of stability up to 2.9 V on gold with molecules with an 18-carbon long aliphatic chain. The length of the chains is certainly crucial in the stability of the SAM-forming system as the products of the same chemistry, despite their potential stability, can be washed off the surface by rinsing with water.41
Taken together, we have a plethora of SAM-forming systems; all have their strengths and weaknesses, and no system is perfect. The experimentalists’ choice is determined by what they want to achieve and modify. Organosilanes are currently still the most suitable for glass surfaces, alkanethiols for noble metals, aryl diazoniums for carbon, alkynes for silicon and organophosphonates for ITO. If a high degree of spatial control is needed, then we would lean towards alkanethiol chemistry or the alkynes on silicon. If order is not so important but long-term stability is, then we would choose aryl diazonium salt layers on carbon. If order and transparency are needed, then the organophosphonates on ITO seem the best option.
The application of SAMs in biosensing
As indicated above, one of the advantages of monolayer modification of surfaces is that multiple different chemical functionalities have been incorporated into a single layer to allow the surface to perform multiple functionalities at the same time. This possibility is incredibly important for biosensing as biosensors are interfacial devices where the surface must allow the immobilisation of receptor molecules to facilitate the sensor being selective for the analyte of interest. At the same time, other species, which may give interfering signals, must be repelled from the surface. And in many cases, the sample to be analysed is a biological fluid full of cells and proteins, like whole blood. So, in many ways, biosensors are a major surface chemistry challenge.
We are one of the laboratories that have explored using SAMs for sensing over a few decades.42 Rather than developing sensors for a given analyte, our approach was to develop solutions to challenges that affected many different sensors. This strategy was motivated by the desire to make conceptual advances in sensing. We drew inspiration from the lateral flow devices, now familiar to us all through the COVID tests, which showed the power of a sensing concept that could be applied to many different analytes. Recognising the interfacial nature of biosensors, and that many of the demands a biosensor is required to fulfil are interfacial, we have used SAMs to address basic conceptual questions in sensing such as: (1) how simple can a biorecognition species be? (2) Can we develop biosensors where the signal only comes from the biorecognition event? (3) Can we make electrodes that can detect proteins in whole blood? (4) Can we develop electrochemical sensing arrays that do not require wires to each and every area of a surface? And (5) how do we develop biosensors that can detect ultralow amounts of analyte but still have a rapid response time? To answer many of these questions required advancing fundamental aspects of the monolayer question, but with a clear application focus in mind. We will now cover our work to address each of these questions and what we learnt about the surface chemistry along the journey.
How simple can a biorecognition species be?
One of the central challenges in biosensing has been the stability of the biomolecules that are employed. This is a particularly prevalent concern for enzyme biosensors where the activity of the biomolecule is central to the signal the biosensor gives but it is also relevant to other proteins where the selectivity comes directly from a binding event without an ensuing effector function. This is the case with affinity species such as antibodies, aptamers and peptides. We decided to strip the protein down to its simplest constituents, a few amino acids, to see if we could still get selective sensors. We started with peptides for detecting metal ions and showed a simple peptide of glycine–glycine–histidine could selectively detect Cu2+ with unprecedently low detection limits at the time, of below parts per trillion (Fig. 2).29 The sensor was prepared by modifying a gold electrode with 3-mercaptopropionic acid to which the peptide was attached. As the Cu2+ is electroactive, the short-chain SAM was used to anchor the peptide to the electrode without preventing electrochemistry at the underlying surface.
In variants of this work, we also sought to synthesise peptides off the SAM surface and attach several peptides to the same electrode to use statistical methods to detect multiple species on the same electrode.43 The other interfacial design aspect of this very simple interface is that the spacing between peptides can be precisely controlled by forming mixed layers where the number of coupling points to non-coupling points can be altered by altering the ratio of two SAM-forming molecules in solution and subsequently attaching the peptide. We demonstrated this not with alkanethiol chemistry but with organophosphonates on ITO for the immobilisation of the cell adhesive peptide arginine–glycine–aspartic acid to allow dual fluorescence microscopy and electrochemical impedance spectroscopy measurements for drug response studies and showing how the surface design influences the drug response.36
Notably, this research program was where we first started to suffer from the instability of the alkanethiol chemistry, in terms of operational stability when trying to detect Pb2+ with the peptide angiotensin I,44 which required a more negative potential than Cu2+. At this more negative potential, the SAM became unstable. With regards to storage stability where performing multiple fabrication steps, when performing on-surface peptide synthesis, the gold–thiol bond began to oxidise.45
Can we develop biosensors where the signal only comes from the biorecognition event?
As outlined above, how well the interface functions in a sensor is central to its performance. The receptor on the surface is designed to selectively bind to the target species, from which the concentration of analyte is determined. However, in the case of many sensors, any analyte that binds to the interface, regardless of whether it is specific or non-specifically adsorbed, will give a signal. As such, we explored concepts where only the binding event would give a signal. We explored this idea using DNA-modified electrodes and either exploited the change in flexibility44 of the DNA or the change in electron transfer efficiency.46,47 We even showed with the electron transfer studies that complete hybridisation was needed to give the signal46 and any perturbation in the DNA base pair stacking influenced the signal, as shown with DNA base-pair mismatches48 and the DNA binding drug cisplatin bending the DNA and decreasing the current49 (Fig. 3).
(a) Schematic depicting the long-range charge transport in DNA duplex monolayers on gold electrodes and the electron transport disturbance on cisplatin drug-induced DNA base pair breakage. (b, c) Square wave voltammograms of the duplex DNA-modified gold electrode obtained in a phosphate buffer solution (pH 7.0), containing 25 μM of AQMS intercalator, then exposing the interface to 1-μM cisplatin for different periods of time: (b) raw data, and (c) background subtracted. Parts b and c are adapted from Wong and Gooding,49 with permission from American Chemical Society.
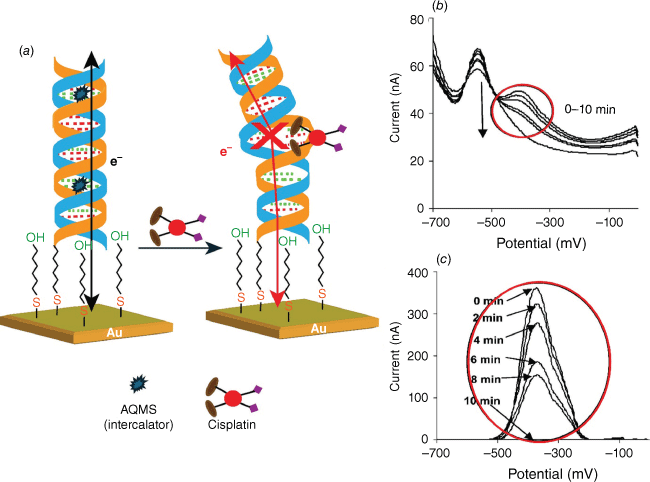
The sensing interface used in this work has become one of the most used sensing interfaces. First developed by Tarlov and co-workers,50,51 it uses DNA with an alkanethiol tail that attaches to the gold surface and, subsequently, a diluent layer of 6-mercaptohexanol is assembled onto the gold surface. Although it is a simple dual-component SAM, what the two components do is quite sophisticated. The DNA naturally provides the recognition element for the binding partners while the diluent lifts the probe DNA off the gold surface and spaces the DNA strands apart, and the hydroxyl moiety in the distal end of the 6-mercaptohexanol diluent is suggested to repel the target DNA off the surface so that hybridisation efficiency is very high.52 The power of this interface is shown in electrochemical aptamer-based (EAB) biosensors where the redox species methylene blue attached to the distal end of the DNA aptamer gives an electrochemical signal.53,54 As aptamers can be thought of as the DNA equivalent of an antibody, the selective recognition of a small molecule such as a drug causes the aptamer to change conformation with a concomitant change in electrochemical signal (Fig. 4). So, in this case, the DNA molecules are acting as molecular switches and so the interface, although still consisting of only two components, is also assisting to orient the DNA for signal generation. As clever as this idea is, what is even more amazing is that the concept was shown to be able to continuously monitor drugs in a live animal in real time, indicating that the layer is also conferring some resistance to non-specific protein adsorption.54
Schematic depicting the structure of electrochemical aptamer-based (EAB) sensors on gold electrodes (MB, methylene blue). The interaction between the aptamer DNA and the target (T) leads to bringing the methylene blue signal closer to the electrode surface, and this results in a faster electron transfer, with the electron transfer rate constant in absence of the target (ket1) is smaller than the electrocn transfer rate constant with the target (ket2), that is correlated to the amount of the target captured from the solution. Also, owing to the reversibility of the aptamer–target binding reaction, EAB sensors are deployed in continuous monitoring devices (note: in the right panel of continous monitoring, the red rectangles represent the signal in presence of the target and the black rectangles represent the signal in absence of the target).
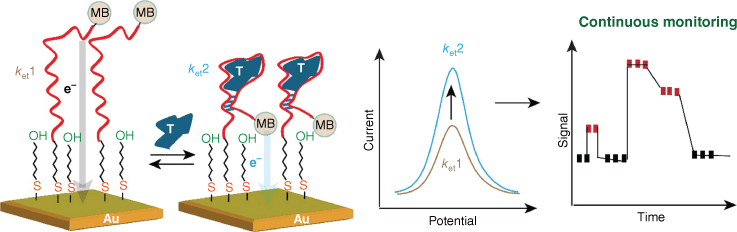
Can we make electrodes that can detect proteins in whole blood?
The first great success of the biosensing world was the glucose sensor that could detect glucose in whole blood. One of the key challenges with these electrochemical devices was preventing electrode fouling when operating in whole blood. As glucose is a small molecule, a very effective strategy is to use a semi-permeable membrane to keep out fouling proteins and cells while allowing small molecules such as glucose to pass through the membrane and react with the enzyme glucose oxidase. However, when the analyte is a protein or a nucleic acid, this strategy does not work because as the foulants and the analyte are similar sizes, the semi-permeable membrane that prevents access of foulants would also prevent access of the analyte. A self-assembled monolayer approach to mitigate electrode fouling was to use alkanethiol SAMs of thiolated-oligoethylene glycol molecules. These have been shown to be highly effective at resisting non-specific adsorption of proteins,55 but these monolayers passivate electrochemistry such that they cannot be used for amperometric sensing. As such, some researchers have looked at using these layers in electrochemical sensors based on impedance spectroscopy, a method that is seldom as robust as amperometry because impedance is so sensitive to any change at a sensing interface.
To employ oligo(ethylene glycol) (OEG) molecules with amperometric sensing, we sought to use SAMs of these molecules but with conducting channels incorporated within the OEG layer. We first did these aryl diazonium salt-derived layers where there was a small subset of molecular wires within the organic OEG layer. To the end of the molecular wire was attached a ferrocene molecule and to that the epitope for an antibody to bind to.56 Subsequently, after discovering that gold nanoparticles attached to long-chain SAMs that otherwise blocked electrochemistry facilitated amperometric reactions,57 we used a similar approach to form conducting channels through an OEG layer. This new strategy greatly simplified the formation of sensing layers that could operate in whole blood, as shown with our work on detecting glycosylated haemoglobin in whole blood in a competition assay (Fig. 5a, b).58 The calibration curve shown in Fig. 5b was obtained in biological fluids and the comparison of the sensor in a human blood sample and an independently measured value by a local pathology laboratory gave very similar values. More recently, the same principle of using nanomaterials to form conducting channels through an otherwise passivating antifouling layer was reported that is much more compatible with bulk manufacture.59,60 We have also come up with an alternative solution to developing immunosensors, also using aryl diazonium salt layers, using aryl phosphoryl choline, which is a zwitterionic antifouling layer that, being a short-chain molecule, allows significant amperometric response at the underlying electrode.61 We have used this approach to show the detection of cytokines in whole blood (Fig. 5c).28
(a) Schematic depicting fabrication of an amperometric immunosensors based on gold nanoparticles (AuNPs)–diazonium salt-modified interfaces for the detection of HbA1c. (b) A calibration curve for HbA1c measured using the competitive inhibition assay where both the IgG (immunoglobulin G) and the target HbA1c were present in the PBS buffer. Part b is adapted from Liu et al.,56 with permission from Wiley. (c) A schematic showing the use of aryldiazonium salts to fabricate an anti-fouling sensing interface composed of mixed layers of phenyl phosphorylcholine (PPC) and phenyl butyric acid (PBA) on an ITO electrode for the detection of tumour necrosis factor α (TNF-α). Part c is adapted from Jiang et al.,28 with permission from the American Chemical Society.
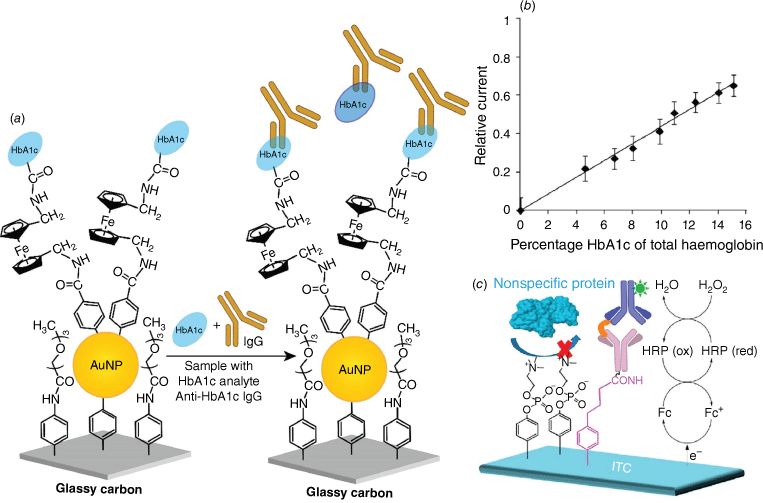
Can we develop electrochemical sensing arrays that do not require wires to each and every area of a surface?
A major challenge for forming electrode arrays is that for each element of an array to be independently addressable, wires and bonding pads are needed for each electrode. This is not such a large problem when one has only a few electrodes. However, these wires and bonding pads take up enormous amounts of space in a chip when the number of electrode elements reaches 100 or more. We asked the question: can we develop electrode arrays for sensing where we do not need each electrode to be connected to a separate set of wires and bonding pads by exploiting semiconducting electrodes? In a semi-conducting electrode that is in depletion, which means it has insufficient carriers to sustain charge flow, light of sufficient energy can be used to enhance the conductivity and allow electrochemistry to proceed. This is because light with energy greater than the band gap of the semi-conductor excites charge carriers such that the illuminated region becomes more conducting. As the enhancement of conductance is only at the site of illumination, one can then make electrode arrays with a single connecting wire simply by illuminating different spots in sequence.
We exploited this concept to make electrode arrays for detecting rare cells and releasing them. As we were detecting cells, we needed a semi-conductor with a band gap in the visible region and the obvious choice was silicon. However, silicon is prone to oxidation in air (Eqn 1) and water (Eqn 2), with the resultant silicon dioxide layer being an insulator.62
As such, we developed surface chemistry that protected the silicon from oxidation even under oxidising potentials. To achieve this, we explored the hydrosilylation of silicon but found that alkene-terminated SAMs, even if formed where there was no apparent silicon dioxide, would rapidly oxidise during electrochemistry in aqueous solution.63 We, however, were also using dialkyne layers to form SAMs with the intention of allowing subsequent click reactions to proceed to add further functionality.64 We serendipitously discovered that such layers also allowed faradaic electrochemistry to proceed without silicon oxidation.65 This enhanced protection of the silicon against oxidation was shown to be due to the fact that the distal end of the monolayer-forming molecules could interact to each other by π–π stacking before and after the click reaction. This attraction between the distal end of the molecules gave the silicon greater protection against oxidation. Note that attractive groups at the distal ends of monolayers are in contrast to most other SAM-forming molecules where the distal moieties repel each other.
The protection of the silicon allowed us to demonstrate the concept of forming electrode arrays with a single wire by connecting to different regions of a surface with light.66 We showed we could differentiate features with a resolution of less than 2 µm67 and that many different regions on a surface could be rapidly interrogated using a data projector lens rather than a moving light pointer.68 We then proceeded to employ this technology in the capture and release of rare cells using electrochemically cleavable surface chemistry.69 The surface chemistry that was employed is shown in Fig. 6, where cells can only be released when simultaneously viewed under a microscope and a potential applied to the silicon surface.33 The advantages of these light-addressable electrode arrays are that two stimuli are required to release each cell so a single cell can be released.
(a) Schematic demonstrating the rare cells (green) capture and release device fabricated from modified silicon interfaces. By controlling the position of the light illumination on the silicon surface and the electrochemical bias, a single cell can be released from the surface. (b) Fluorescence microscopy images for the cell-covered silicon surface before and after releasing a single cell from the surface, as tracked within the red circle. Parts a and b are adapted from Parker et al.,33 with permission from Springer Nature.
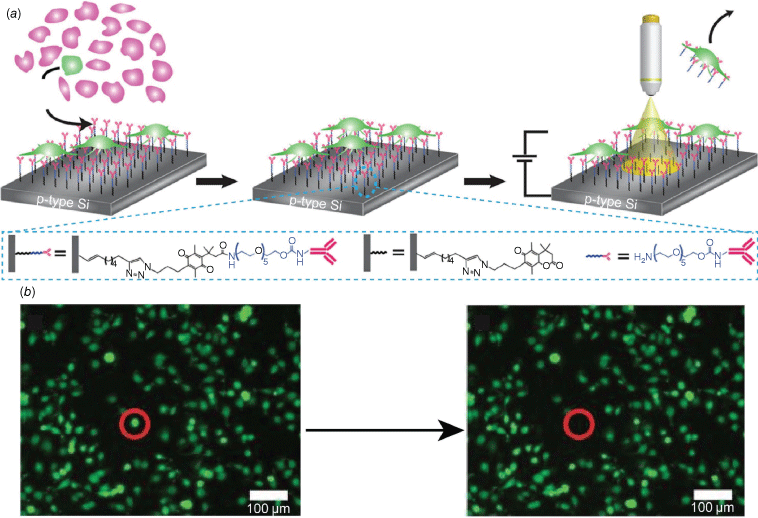
How do we develop biosensors that can detect ultralow amounts of analyte but still have a rapid response time?
The final question we sought to answer where SAMs were pivotal was how do we detect really tiny amounts of material? One often thinks the challenge of ultrasensitivity is an unmet need for more sensitive detectors but, in fact, we have several types of detectors and techniques that can detect single molecules70 including nanopore sensors, fluorescence methods, surface enhanced Raman spectroscopy and other localised surface plasmon resonance type devices. The issue is in fact one of mass transport. With ultralow amounts of analyte, the problem becomes one of the analytes finding the sensor. This is because of diffusion, where the time constants for diffusion have a square dependency on the distance something must diffuse. So, to solve this challenge of slow response times, we proposed to break the sensor up into nanoparticles that were dispersed throughout the sample to be analysed but then reassembled using a magnetic field for subsequent measurement. We referred to this concept as dispersible electrodes, where each nanoparticle was a gold-coated magnetic nanoparticle (Au@MNPs) and the gold was modified with alkanethiol SAMs to allow all the surface chemicals required for sensing to be attached. After each nanoparticle captures its target, all the nanoparticles are returned to a bulk electrode for detection to proceed71 (Fig. 7).
(a) Schematic depicting the use of DNA-functionalised magnetic gold nanoparticles to selectively capture target miRNA (microRNA) from complex biofluid samples. (b) Schematic illustrating the process of pulling down the magnetic nanoparticle with the target miRNA by an external magnetic field to the surface of a gold foil, followed by electrochemical detection of the signal difference between the target-bound and target-non-bound nanoparticles. The difference in the electrochemical signal is proportional to the target amount in the solution.
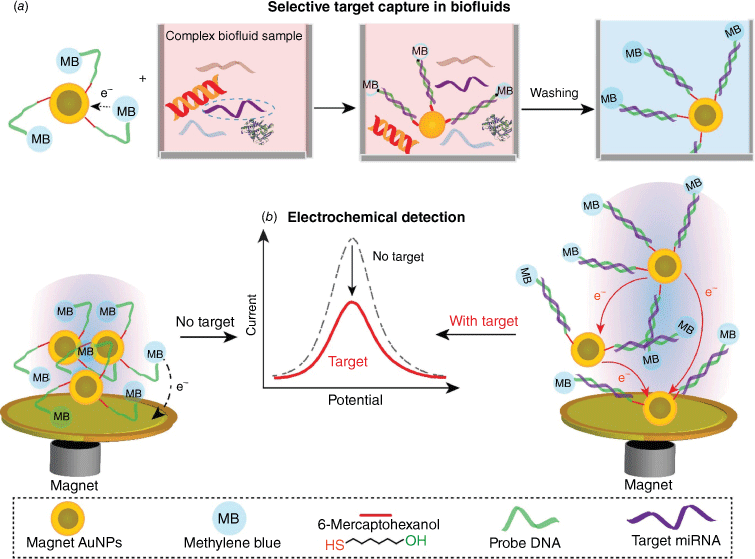
We first showed the utility of the concept for the detection of copper ions using the same peptide approach discussed above. We showed that not only were response times faster, but also we saw lower detection limits.72 The study showed that the lower detection limits were because the dispersible electrodes captured effectively all the analyte such that the sensor was determining the amount of an analyte rather than its concentration. A second important concept with regards to dispersible electrodes is that unlike other sensors where the number of analyte species is well in excess of the number of receptors, with the dispersible electrodes, the number of receptors is massively in excess of the amount of analyte.
The dispersible electrode concept has been applied to metal ions, small organic molecules, proteins and, perhaps most successfully, with nucleic acids73 (Fig. 7a). In the case of detecting nucleic acids, the Au@MNPs were modified with a DNA sequence with an alkanethiol linker on one end and a methylene blue redox-active tag on the other end. The DNA-modified Au@MNPs were dispersed into a biological sample where the DNA-Au@MNPs hybridised with complementary microRNA in the sample. On assembly of the DNA-Au@MNPs on a bulk gold electrode, the electrochemical response of the methylene blue was detected. If the DNA-Au@MNPs captured a complementary microRNA sequence, the electrochemical signal was diminished because the pathway for electrons to hop from one particle to the next was broken by the long rigid nucleic acid duplex formed on hybridisation to the target (Fig. 7b). Because all the nanoparticles above experience a break in the electron-hopping pathway, caused by the duplex formation, they can no longer be interrogated electrochemically, a single strand of microRNA can turn off the electrochemistry of up to 100,000 methylene blue molecules. This inbuilt amplification system gives the approach incredible sensitivity, with as few as 200 molecules in a sample causing an appreciable signal. This powerful technology is now being used to understand the liquid biopsy concept and how small changes in 3-D bioprinted cancer models74 influence their response to chemotherapeutics.
Some final thoughts
This account has covered our work on developing new sensing concepts that have been dependent on fundamental advances in self-assembled monolayers. We feel some of these advances have been quite significant from a surface chemistry perspective, such as our advances on making mixed layers with aryl diazonium salts or the use of dialkynes to stabilise silicon against oxidation. Other surface chemistry advances revolve more around the new capabilities that the surface chemistry allows. This is particularly pertinent in sensing as sensors are interfacial devices. However, we feel the central point here is that even when doing fundamental research, having a clear applied focus can greatly facilitate the advances made. It also assists in blurring the artificial distinction between fundamental and applied research.
It is worth, however, discussing how the surface chemistry advances described have progressed towards commercialisation and what the challenges are in the surface chemistry. Alkanethiol surface chemistry is already used in several commercial sensing technologies, most notably with the surface plasmon resonance instruments that often use the surface chemistry for specialised chips. We have also used knowledge from the surface chemistry of monolayers in relation to cell adhesion75,76 to develop the inks for commercialised 3-D bioprinters for producing 3-D cell cultures in high throughput.74,77 Equally notable is that a number of companies are attempting to commercialise electrochemical aptamer-based (EAB) sensors (Fig. 5), all of which use alkanethiol chemistry to develop the sensing interface. As the EAB sensors are targeted towards long-term monitoring of molecules in the body, the challenges with the stability of alkanethiol chemistry are again becoming pertinent.78
The EAB sensor concept, however, shows in many ways the incredible power of SAMs for developing sensors. In this rather simple interface, a change in the spatial position of a redox species on analyte binding at the molecular level is the key signal transduction while the diluent serves to space the DNA apart, influence its conformation, limit electrode fouling and limit the availability of oxygen at the electrode surface. That is, the interface is performing multiple functions at the same time, with molecular level control, to allow a sensor to operate in vivo based on a change in molecular conformation.79 This is quite incredible and highlights the power and possibility SAMs provide. It is clear, however, that for some applications, further developments are needed to understand these interfaces, make them more stable and make them better at resisting non-specific protein adsorption, all while ensuring their formation is simple enough to be compatible with bulk manufacture.
Declaration of funding
The authors acknowledge funding from the Australian Research Council and the National Health and Medical Research Council for their generous support throughout the authors’ careers. Particular grants to be acknowledged are the NHMRC Investigator grant (GNT1196648 to J. J. Gooding) and an ARC Industry Laureate Fellowship (IL240100091 to J. J. Gooding).
Acknowledgements
J. J. Gooding acknowledges all the bench researchers and collaborators throughout his career who have contributed to the work presented herein. He also acknowledges the career-long support from the University of New South Wales.
References
1 Ulman A. Formation and structure of self-assembled monolayers. Chem Rev 1996; 96(4): 1533-54.
| Crossref | Google Scholar | PubMed |
2 Sagiv J. Organized monolayers by adsorption. 1. Formation and structure of oleophobic mixed monolayers on solid surfaces. J Am Chem Soc 1980; 102(1): 92-8.
| Crossref | Google Scholar |
3 Nuzzo RG, Allara DL. Adsorption of bifunctional organic disulfides on gold surfaces. J Am Chem Soc 1983; 105(13): 4481-3.
| Crossref | Google Scholar |
4 Delamar M, Hitmi R, Pinson J, Saveant JM. Covalent modification of carbon surfaces by grafting of functionalized aryl radicals produced from electrochemical reduction of diazonium salts. J Am Chem Soc 1992; 114(14): 5883-4.
| Crossref | Google Scholar |
5 Linford MR, Chidsey CED. Alkyl monolayers covalently bonded to silicon surfaces. J Am Chem Soc 1993; 115(26): 12631-2.
| Crossref | Google Scholar |
6 Laibinis PE, Hickman JJ, Wrighton MS, Whitesides GM. Orthogonal self-assembled monolayers: alkanethiols on gold and alkane carboxylic acids on alumina. Science 1989; 245(4920): 845-7.
| Crossref | Google Scholar | PubMed |
7 Gardner TJ, Frisbie CD, Wrighton MS. Systems for orthogonal self-assembly of electroactive monolayers on Au and ITO: an approach to molecular electronics. J Am Chem Soc 1995; 117(26): 6927-33.
| Crossref | Google Scholar |
8 Allara DL, Nuzzo RG. Spontaneously organized molecular assemblies. 1. Formation, dynamics, and physical properties of n-alkanoic acids adsorbed from solution on an oxidized aluminum surface. Langmuir 1985; 1(1): 45-52.
| Crossref | Google Scholar |
10 Love JC, Estroff LA, Kriebel JK, Nuzzo RG, Whitesides GM. Self-assembled monolayers of thiolates on metals as a form of nanotechnology. Chem Rev 2005; 105(4): 1103-69.
| Crossref | Google Scholar | PubMed |
11 Smith RK, Lewis PA, Weiss PS. Patterning self-assembled monolayers. Prog Surf Sci 2004; 75(1): 1-68.
| Crossref | Google Scholar |
12 Wen K, Maoz R, Cohen H, Sagiv J, Gibaud A, Desert A, et al. Postassembly chemical modification of a highly ordered organosilane multilayer: new insights into the structure, bonding, and dynamics of self-assembling silane monolayers. ACS Nano 2008; 2(3): 579-99.
| Crossref | Google Scholar | PubMed |
13 Li M, Liu M, Qi F, Lin FR, Jen AK-Y. Self-assembled monolayers for interfacial engineering in solution-processed thin-film electronic devices: design, fabrication, and applications. Chem Rev 2024; 124(5): 2138-204.
| Crossref | Google Scholar | PubMed |
14 Casalini S, Bortolotti CA, Leonardi F, Biscarini F. Self-assembled monolayers in organic electronics. Chem Soc Rev 2017; 46(1): 40-71.
| Crossref | Google Scholar | PubMed |
15 Zeira A, Chowdhury D, Maoz R, Sagiv J. Contact electrochemical replication of hydrophilic−hydrophobic monolayer patterns. ACS Nano 2008; 2(12): 2554-68.
| Crossref | Google Scholar | PubMed |
16 Gooding JJ, Parker SG, Lu Y, Gaus K. Molecularly engineered surfaces for cell biology: from static to dynamic surfaces. Langmuir 2014; 30(12): 3290-302.
| Crossref | Google Scholar | PubMed |
17 Gooding JJ, Ciampi S. The molecular level modification of surfaces: from self-assembled monolayers to complex molecular assemblies. Chem Soc Rev 2011; 40(5): 2704-18.
| Crossref | Google Scholar | PubMed |
18 Cornell BA, Braach-Maksvytis VLB, King LG, Osman PDJ, Raguse B, Wieczorek L, et al. A biosensor that uses ion-channel switches. Nature 1997; 387(6633): 580-3.
| Crossref | Google Scholar | PubMed |
19 Finklea HO. Electrochemistry of organized monolayers of thiols and related molecules on electrodes. Electroanal Chem 1996; 19: 110-337.
| Google Scholar |
20 Darwish N, Paddon-Row MN, Gooding JJ. Surface-bound norbornylogous bridges as molecular rulers for investigating interfacial electrochemistry and as single molecule switches. Acc Chem Res 2014; 47(2): 385-95.
| Crossref | Google Scholar | PubMed |
21 Eggers PK, Darwish N, Paddon-Row MN, Gooding JJ. Surface-bound molecular rulers for probing the electrical double layer. J Am Chem Soc 2012; 134(17): 7539-44.
| Crossref | Google Scholar | PubMed |
22 Darwish N, Eggers PK, Ciampi S, Tong Y, Ye S, Paddon-Row MN, et al. Probing the effect of the solution environment around redox-active moieties using rigid anthraquinone-terminated molecular rulers. J Am Chem Soc 2012; 134(44): 18401-9.
| Crossref | Google Scholar | PubMed |
23 Vericat C, Vela ME, Salvarezza RC. Self-assembled monolayers of alkanethiols on Au(111): surface structures, defects and dynamics. Phys Chem Chem Phys 2005; 7(18): 3258-68.
| Crossref | Google Scholar | PubMed |
24 Siddiqui A-R, N’Diaye J, Santiago-Carboney A, Martin K, Bhargava R, Rodríguez-López J. Spectroelectrochemical determination of thiolate self-assembled monolayer adsorptive stability in aqueous and non-aqueous electrolytes. Analyst 2024; 149(10): 2842-54.
| Crossref | Google Scholar | PubMed |
25 Mearns FJ, Wong ELS, Short KT, Hibbert DB, Gooding JJ. DNA biosensor concepts based on a change in the DNA persistence length upon hybridization. Electroanalysis 2006; 18: 1971-81.
| Crossref | Google Scholar |
26 Gooding JJ. Advances in interfacial design for electrochemical biosensors and sensors: aryl diazonium salts for modifying carbon and metal electrodes. Electroanalysis 2008; 20(6): 573-82.
| Crossref | Google Scholar |
27 Liu G, Liu J, Böcking T, Eggers PK, Gooding JJ. The modification of glassy carbon and gold electrodes with aryl diazonium salt: the impact of the electrode materials on the rate of heterogeneous electron transfer. Chem Phys 2005; 319(1): 136-46.
| Crossref | Google Scholar |
28 Jiang C, Alam MT, Silva SM, Taufik S, Fan S, Gooding JJ. Unique sensing interface that allows the development of an electrochemical immunosensor for the detection of tumor necrosis factor α in whole blood. ACS Sens 2016; 1(12): 1432-8.
| Crossref | Google Scholar |
29 Yang W, Jaramillo D, Gooding JJ, Hibbert DB, Zhang R, Willett GD, et al. Sub-ppt detection limits for copper ions with Gly-Gly-His modified electrodes. Chem Commun 2001; 2001(19): 1982-1983.
| Crossref | Google Scholar | PubMed |
30 Collman JP, Devaraj NK, Eberspacher TPA, Chidsey CED. Mixed azide-terminated monolayers: a platform for modifying electrode surfaces. Langmuir 2006; 22(6): 2457-2464.
| Crossref | Google Scholar |
31 Ng CC, Magenau A, Ngalim SH, Ciampi S, Chockalingham M, Harper JB, et al. Using an electrical potential to reversibly switch surfaces between two states for dynamically controlling cell adhesion. Angew Chem Int Ed 2012; 51(31): 7706-10.
| Crossref | Google Scholar | PubMed |
32 Lian J, Tang W, Yang Y, Vaidyanathan R, Gonçales VR, Arman SY, et al. A transparent semiconducting surface for capturing and releasing single cells from a complex cell mixture. ACS Appl Mater Interfaces 2022; 14(16): 18079-86.
| Crossref | Google Scholar | PubMed |
33 Parker SG, Yang Y, Ciampi S, Gupta B, Kimpton K, Mansfeld FM, et al. A photoelectrochemical platform for the capture and release of rare single cells. Nat Commun 2018; 9(1): 2288.
| Crossref | Google Scholar | PubMed |
34 Hanson EL, Schwartz J, Nickel B, Koch N, Danisman MF. Bonding self-assembled, compact organophosphonate monolayers to the native oxide surface of silicon. J Am Chem Soc 2003; 125(51): 16074-80.
| Crossref | Google Scholar | PubMed |
35 Chockalingam M, Darwish N, Le Saux G, Gooding JJ. Importance of the indium tin oxide substrate on the quality of self-assembled monolayers formed from organophosphonic acids. Langmuir 2011; 27(6): 2545-52.
| Crossref | Google Scholar | PubMed |
36 Parviz M, Gaus K, Gooding JJ. Simultaneous impedance spectroscopy and fluorescence microscopy for the real-time monitoring of the response of cells to drugs. Chem Sci 2017; 8(3): 1831-40.
| Crossref | Google Scholar | PubMed |
37 Yang Y, Ma Y, Berengut JF, Lee LK, Tilley RD, Gaus K, et al. Electrochemically controlled blinking of fluorophores for quantitative STORM imaging. Nat Photon 2024; 18(7): 713-20.
| Crossref | Google Scholar |
38 Chen X, Luais E, Darwish N, Ciampi S, Thordarson P, Gooding JJ. Studies on the effect of solvents on self-assembled monolayers formed from organophosphonic acids on indium tin oxide. Langmuir 2012; 28(25): 9487-95.
| Crossref | Google Scholar | PubMed |
39 Senthilkumar M, Mathiyarasu J, Joseph J, Phani KLN, Yegnaraman V. Electrochemical instability of indium tin oxide (ITO) glass in acidic pH range during cathodic polarization. Mater Chem Phys 2008; 108(2): 403-7.
| Crossref | Google Scholar |
40 Crudden CM, Horton JH, Ebralidze II, Zenkina OV, McLean AB, Drevniok B, et al. Ultra stable self-assembled monolayers of N-heterocyclic carbenes on gold. Nat Chem 2014; 6(5): 409-14.
| Crossref | Google Scholar | PubMed |
41 Badgurjar D, Huynh M, Masters B, Wuttig A. Non-covalent interactions mimic the covalent: an electrode-orthogonal self-assembled layer. J Am Chem Soc 2023; 145(32): 17734-45.
| Crossref | Google Scholar | PubMed |
42 Gooding JJ, Darwish N. The rise of self-assembled monolayers for fabricating electrochemical biosensors—an interfacial perspective. Chem Rec 2012; 12(1): 92-105.
| Crossref | Google Scholar | PubMed |
43 Ebrahimi D, Chow E, Gooding JJ, Hibbert DB. Multi-analyte sensing: a chemometrics approach to understanding the merits of electrode arrays versus single electrodes. Analyst 2008; 133(8): 1090-6.
| Crossref | Google Scholar | PubMed |
44 Chow E, Hibbert DB, Gooding JJ. Electrochemical detection of lead ions via the covalent attachment of human angiotensin I to mercaptopropionic acid and thioctic acid self-assembled monolayers. Anal Chim Acta 2005; 543(1): 167-76.
| Crossref | Google Scholar |
45 Liu G, Nguyen QT, Chow E, Böcking T, Hibbert DB, Gooding JJ. Study of factors affecting the performance of voltammetric copper sensors based on Gly-Gly-His modified glassy carbon and gold electrodes. Electroanalysis 2006; 18(12): 1141-51.
| Crossref | Google Scholar |
46 Wong ELS, Chow E, Gooding JJ. DNA recognition interfaces: the influence of interfacial design on the efficiency and kinetics of hybridization. Langmuir 2005; 21(15): 6957-65.
| Crossref | Google Scholar | PubMed |
47 Wong ELS, Gooding JJ. Charge transfer through DNA: A selective electrochemical DNA biosensor. Anal Chem 2006; 78(7): 2138-44.
| Crossref | Google Scholar | PubMed |
48 Wong ELS, Gooding JJ. Electronic detection of target nucleic acids by a 2,6-disulfonic acid anthraquinone intercalator. Anal Chem 2003; 75(15): 3845-52.
| Crossref | Google Scholar | PubMed |
49 Wong ELS, Gooding JJ. The electrochemical monitoring of the perturbation of charge transfer through DNA by cisplatin. J Am Chem Soc 2007; 129(29): 8950-1.
| Crossref | Google Scholar | PubMed |
50 Peterlinz KA, Georgiadis RM, Herne TM, Tarlov MJ. Observation of hybridization and dehybridization of thiol-tethered DNA using two-color surface plasmon resonance spectroscopy. J Am Chem Soc 1997; 119(14): 3401-2.
| Crossref | Google Scholar |
51 Herne TM, Tarlov MJ. Characterization of DNA probes immobilized on gold surfaces. J Am Chem Soc 1997; 119(38): 8916-20.
| Crossref | Google Scholar |
52 Levicky R, Herne TM, Tarlov MJ, Satija SK. Using self-assembly to control the structure of DNA monolayers on gold: a neutron reflectivity study. J Am Chem Soc 1998; 120(38): 9787-92.
| Crossref | Google Scholar |
53 Ferguson BS, Hoggarth DA, Maliniak D, Ploense K, White RJ, Woodward N, et al. Real-time, aptamer-based tracking of circulating therapeutic agents in living animals. Sci Transl Med 2013; 5(213): 213ra165.
| Crossref | Google Scholar | PubMed |
54 Arroyo-Currás N, Somerson J, Vieira PA, Ploense KL, Kippin TE, Plaxco KW. Real-time measurement of small molecules directly in awake, ambulatory animals. Proc Natl Acad Sci USA 2017; 114(4): 645-50.
| Crossref | Google Scholar | PubMed |
55 Lopez GP, Albers MW, Schreiber SL, Carroll R, Peralta E, Whitesides GM. Convenient methods for patterning the adhesion of mammalian cells to surfaces using self-assembled monolayers of alkanethiolates on gold. J Am Chem Soc 1993; 115(13): 5877-8.
| Crossref | Google Scholar |
56 Liu G, Paddon-Row MN, Gooding JJ. Protein modulation of electrochemical signals: application to immunobiosensing. Chem Commun 2008; 2008(33): 3870-3872.
| Crossref | Google Scholar | PubMed |
57 Shein JB, Lai LMH, Eggers PK, Paddon-Row MN, Gooding JJ. Formation of efficient electron transfer pathways by adsorbing gold nanoparticles to self-assembled monolayer modified electrodes. Langmuir 2009; 25(18): 11121-8.
| Crossref | Google Scholar | PubMed |
58 Liu G, Iyengar SG, Gooding JJ. An amperometric immunosensor based on a gold nanoparticle-diazonium salt modified sensing interface for the detection of HbA1c in human blood. Electroanalysis 2013; 25(4): 881-7.
| Crossref | Google Scholar |
59 Sabaté del Río J, Henry OYF, Jolly P, Ingber DE. An antifouling coating that enables affinity-based electrochemical biosensing in complex biological fluids. Nat Nanotechnol 2019; 14(12): 1143-9.
| Crossref | Google Scholar | PubMed |
60 Lee J-C, Kim SY, Song J, Jang H, Kim M, Kim H, et al. Micrometer-thick and porous nanocomposite coating for electrochemical sensors with exceptional antifouling and electroconducting properties. Nat Commun 2024; 15(1): 711.
| Crossref | Google Scholar | PubMed |
61 Gui AL, Luais E, Peterson JR, Gooding JJ. Zwitterionic phenyl layers: finally, stable, anti-biofouling coatings that do not passivate electrodes. ACS Appl Mater Interfaces 2013; 5(11): 4827-35.
| Crossref | Google Scholar | PubMed |
62 Wayner DDM, Wolkow RA. Organic modification of hydrogen terminated silicon surfaces. J Chem Soc, Perkin Trans 2 2002; 2002(1): 23-34.
| Crossref | Google Scholar |
63 Yu J, Losic D, Marshall M, Böcking T, Gooding JJ, Shapter JG. Preparation and characterisation of an aligned carbon nanotube array on the silicon(100) surface. Soft Matter 2006; 2(12): 1081-8.
| Crossref | Google Scholar | PubMed |
64 Ciampi S, Böcking T, Kilian KA, James M, Harper JB, Gooding JJ. Functionalization of acetylene-terminated monolayers on Si(100) surfaces: a click chemistry approach. Langmuir 2007; 23(18): 9320-9.
| Crossref | Google Scholar | PubMed |
65 Ciampi S, Eggers PK, Le Saux G, James M, Harper JB, Gooding JJ. Silicon(100) electrodes resistant to oxidation in aqueous solutions: an unexpected benefit of surface acetylene moieties. Langmuir 2009; 25(4): 2530-9.
| Crossref | Google Scholar | PubMed |
66 Choudhury MH, Ciampi S, Yang Y, Tavallaie R, Zhu Y, Zarei L, et al. Connecting electrodes with light: one wire, many electrodes. Chem Sci 2015; 6(12): 6769-76.
| Crossref | Google Scholar | PubMed |
67 Gautam S, Gonçales VR, Colombo RNP, Tang W, Córdoba de Torresi SI, Reece PJ, et al. High-resolution light-activated electrochemistry on amorphous silicon-based photoelectrodes. Chem Commun 2020; 56(54): 7435-8.
| Crossref | Google Scholar | PubMed |
68 Vogel YB, Gonçales VR, Al-Obaidi L, Gooding JJ, Darwish N, Ciampi S. Nanocrystal inks: photoelectrochemical printing of Cu2O nanocrystals on silicon with 2D control on polyhedral shapes. Adv Funct Mater 2018; 28(51): 1804791.
| Crossref | Google Scholar |
69 Ciampi S, James M, Le Saux G, Gaus K, Justin Gooding J. Electrochemical ‘switching’ of Si(100) modular assemblies. J Am Chem Soc 2012; 134(2): 844-7.
| Crossref | Google Scholar | PubMed |
70 Gooding JJ, Gaus K. Single-molecule sensors: challenges and opportunities for quantitative analysis. Angew Chem Int Ed Engl 2016; 55(38): 11354-66.
| Crossref | Google Scholar | PubMed |
71 Moraes Silva S, Tavallaie R, Sandiford L, Tilley RD, Gooding JJ. Gold-coated magnetic nanoparticles: from preparation to surface modification for analytical and biomedical applications. Chem Commun 2016; 52(48): 7528-40.
| Crossref | Google Scholar | PubMed |
72 Goon IY, Lai LM, Lim M, Amal R, Gooding JJ. ‘Dispersible electrodes’: a solution to slow response times of sensitive sensors. Chem Commun 2010; 46(46): 8821-3.
| Crossref | Google Scholar | PubMed |
73 Tavallaie R, McCarroll J, Le Grand M, Ariotti N, Schuhmann W, Bakker E, et al. Nucleic acid hybridization on an electrically reconfigurable network of gold-coated magnetic nanoparticles enables microRNA detection in blood. Nat Nanotechnol 2018; 13(11): 1066-71.
| Crossref | Google Scholar | PubMed |
74 Utama RH, Atapattu L, O’Mahony AP, Fife CM, Baek J, Allard T, et al. A 3D bioprinter specifically designed for the high-throughput production of matrix-embedded multicellular spheroids. iScience 2020; 23(10): 101621.
| Crossref | Google Scholar | PubMed |
75 Le Saux G, Magenau A, Gunaratnam K, Kilian KA, Böcking T, Gooding JJ, et al. Spacing of integrin ligands influences signal transduction in endothelial cells. Biophys J 2011; 101(4): 764-73.
| Crossref | Google Scholar | PubMed |
76 Le Saux G, Magenau A, Böcking T, Gaus K, Gooding JJ. The relative importance of topography and RGD ligand density for endothelial cell adhesion. PLoS One 2011; 6(7): e21869.
| Crossref | Google Scholar | PubMed |
77 Utama RH, Tan VTG, Tjandra KC, Sexton A, Nguyen DHT, O’Mahony AP, et al. A covalently crosslinked ink for multimaterials drop-on-demand 3D bioprinting of 3D cell cultures. Macromol Biosci 2021; 21(9): e2100125.
| Crossref | Google Scholar | PubMed |
78 Arroyo-Currás N. Beyond the gold–thiol paradigm: exploring alternative interfaces for electrochemical nucleic acid-based sensing. ACS Sens 2024; 9(5): 2228-36.
| Crossref | Google Scholar | PubMed |
79 Downs AM, Plaxco KW. Real-time, in vivo molecular monitoring using electrochemical aptamer based sensors: opportunities and challenges. ACS Sens 2022; 7(10): 2823-32.
| Crossref | Google Scholar | PubMed |