Synthesis and stability studies of constrained peptide–antimony bicycles
Sven Ullrich

A
Handling Editor: Curt Wentrup
Abstract
Peptide therapeutics play an increasingly important role in modern drug discovery. Improving the pharmacokinetic profile of bioactive peptides has been effectively achieved with chemical modifications, especially macrocyclisation reactions. Consequently, there is a great demand for highly constrained compounds such as bicyclic peptides. In our previous research, we introduced peptide–bismuth bicycles and peptide–arsenic bicycles as new classes of constrained peptides. In this work, we extend our peptide bicyclisation strategy towards antimony. Similar to arsenic and bismuth, antimony(III) selectively binds to three cysteine residues in peptides, enabling the in situ formation of stable bicycles. The bicyclisation reaction occurs instantaneously under biocompatible conditions at physiological pH. Antimony–peptide bicycles remain largely intact in the presence of the common metal chelator ethylenediaminetetraacetic acid (EDTA) and the main endogenous thiol competitor glutathione (GSH). Furthermore, when challenged with bismuth(III) from water-soluble gastrodenol (bismuth tripotassium dicitrate), antimony–peptide bicycles convert into the corresponding bismuth–peptide bicycle, highlighting the superior thiophilicity of bismuth over other pnictogens. Our study further expands the toolbox of peptide multicyclisation with main group elements previously underexplored in chemical biology.
Keywords: antimony, bicycles, bismuth, cyclisation, cysteine, macrocycles, peptides, pnictogens.
Introduction
Peptide-based drugs occupy a growing niche in the therapeutic landscape.1–3 A wide range of chemical transformations to modify peptides contributes to their success.4 At the forefront of this are constrained peptides. Their conformational freedom is restricted into cyclic, stapled or multicyclic topologies by one or more key modifications.5–8 This generally improves the pharmaceutical properties of the peptide, such as prolonged metabolic stability, increased binding affinities due to pre-organisation and, in some instances, enhanced cell permeability.5,9 Although constrained peptides have the potential to match the high binding affinity and selectivity of antibodies, they remain accessible through chemical synthesis.5,10,11 Owing to these qualities, bioactive peptide macrocycles are considered highly promising next-generation drugs.12
The identification of targeted peptide macrocycles can be facilitated by genetically encoded libraries and display screenings.13,14 Resulting hit compounds can be further refined through conventional medicinal chemistry where certain properties such as binding affinity or cell membrane permeability are further improved.15–17 With increasing uptake of these drug discovery techniques, the next wave of therapeutic peptide macrocycles is expected to originate from such encoded libraries.18,19 It is therefore a priority in peptide research to innovate peptide modification and cyclisation with a focus on biocompatibility for display screenings.20 As multicyclic peptides with enhanced structural constraints emerge as promising drugs, an increasing number of bicyclic peptide syntheses are appearing in the literature.7,21–24
The pronounced reactivity of cysteine residues is frequently exploited for peptide (multi)cyclisation.25,26 For instance, alkylating agents such as 1,3,5-tris(bromomethyl)benzene (TBMB) are widely used to generate bicyclic peptide libraries on phage.27 Our group has described an alternative class of peptide bicycles scaffolded around a single thiophilic element.28 We pioneered the synthesis of bioactive and cell-permeable peptide–bismuth bicycles, where bismuth(III) is covalently bound to three cysteine residues of the peptide.28,29 Owing to the minimalistic ‘scaffold’ of a single atom, the constraint of the bicyclic peptide is enhanced compared to conventional scaffolding agents.28 This single-element strategy has recently been expanded to arsenic(III).30 Additionally, the compatibility of both bismuth(III) and arsenic(III) modification techniques with phage display screenings was successfully established.30,31 We hypothesised that antimony, located in group 15 between arsenic and bismuth, is similarly suitable for peptide bicyclisation. Hence, this study describes the first synthesis, characterisation and stability evaluation of SbIII-centred bicyclic peptides.
Results and discussion
Antimony trichloride (SbCl3) from a concentrated dimethyl sulfoxide (DMSO) stock served as the reagent to access SbIII-centred bicyclic peptides. The reagent was added to a peptide comprising three cysteine residues (1a) in aqueous buffer at physiological pH (Fig. 1a). Liquid chromatography–mass spectrometry (LCMS) revealed the presence of the resulting bicyclic peptide 1b with ≥95% conversion (Fig. 1b, Table 1). High-resolution mass spectrometry (HRMS) confirmed the identity of the peptide–antimony bicycle. Its distinctive metalloid core exhibits a characteristic isotope pattern (Fig. 1c). We further expanded this technique to various triple-cysteine peptides 2a–7a – covering hydrophobic, polar, acidic and basic amino acids – and converted them into their respective bicyclic peptide (Table 1). LCMS analysis confirmed ≥95% presence of the bicyclic peptides after exposure to SbCl3 (Table 1).
Synthesis of model peptide–antimony bicycle 1b. (a) Reaction scheme. (b) High-performance liquid chromatography (HPLC) trace. (c) Simulated isotope pattern (grey) and high-resolution positive electrospray ionisation (ESI+) mass spectrum (blue) of the [M + Na]+ adduct.
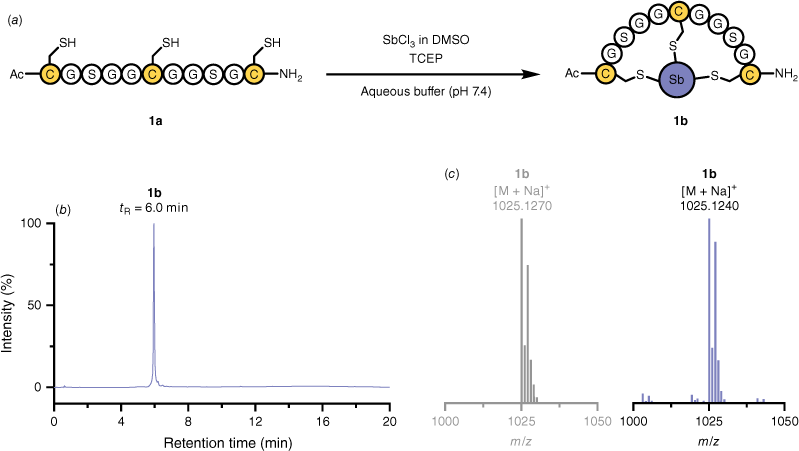
Linear peptide | Sequence | Antimony bicycle | Conversion (%) | |
---|---|---|---|---|
1a | Ac-CGSGGCGGSGC-NH2 | 1b | ≥95 | |
2a | H-ACSHPMCEGFPC-NH2 | 2b | ≥95 | |
3a | H-ACPHPQCEAAAC-NH2 | 3b | ≥95 | |
4a | H-ACHPQNCTEPAC-NH2 | 4b | ≥95 | |
5a | H-ACHPQVCSPTEC-NH2 | 5b | ≥95 | |
6a | H-ACTLRQCILQSC-NH2 | 6b | ≥95 | |
7a | Ac-CGSGGSGCGSGGSGC-NH2 | 7b | ≥95 |
The peptide sequences are shown using single-letter amino acid codes. N-terminal and C-terminal modifications are indicated. Antimony bicycles listed are the peptide–antimony bicycles 1b–7b of the respective linear sequences. Trivalent antimony is linked to the sulfur atoms of the three cysteines. Conversion yield of linear peptides 1a–7a into 1b–7b in aqueous buffer (20-mM tris(hydroxymethyl)aminomethane hydrochloride, 2-mM tris(2-carboxyethyl)phosphine, pH 7.4) using 3 equiv. of SbCl3 determined by LC-MS (254 nm). Peptide–bismuth bicycles of 1a and 2a are respectively referred to as 1c and 2c in the main text.
Next, we assessed the stability of the peptide–antimony bicycles in the presence of chelators and thiol reagents (Fig. 2, Supplementary Tables S3–S5). Specifically, we conducted competition experiments with ethylenediaminetetraacetic acid (EDTA) and glutathione (GSH). The evaluated bicyclic peptides 1b and 2b exhibited similar trends in the presence of the hexadentate metal chelator EDTA. At a five-fold excess of EDTA, ~90% of the peptide–antimony bicycles remained intact. Even at a 25-fold excess of EDTA, complete dissociation of SbIII from the peptides was not observed, as the fraction of peptide–antimony bicycles remained at 22 and 49% for 1b and 2b respectively (Fig. 2a), suggesting an equilibrium between the Sb(peptide) and Sb(EDTA) complexes with a stronger stability constant of the peptide–antimony complex. To assess the stability of our bicycles in a natural reducing environment, we determined their stability in the presence of GSH, the most abundant intracellular thiol.32 We anticipated that binding of three thiols from cysteine residues to SbIII may be affected by competition with other thiol-containing compounds such as GSH. For 1b, we observed increased decomposition of the bicycle with increasing equivalents of GSH, resulting in 40% intact peptide bicycle at a 25-fold excess of GSH. Bicycle 2b was found to be more resistant. Even at the highest analysed GSH concentration (25 equiv., 12.5 mM) only ~20% decomposition was observed (Fig. 2b). Considering that the GSH concentration in most cells is ~2 mM, this result suggests that peptide–antimony bicycles are sufficiently guarded.33
Stability of peptide–antimony bicycles 1b (orange square) and 2b (green circle) in competition with (a) ethylenediaminetetraacetic acid (EDTA) and (b) glutathione (GSH). Bicycles (0.5 mM) were incubated with increasing equivalents (0.5, 5, 10 and 25) of EDTA (a) or GSH (b) for 1 h at room temperature to assess stability.
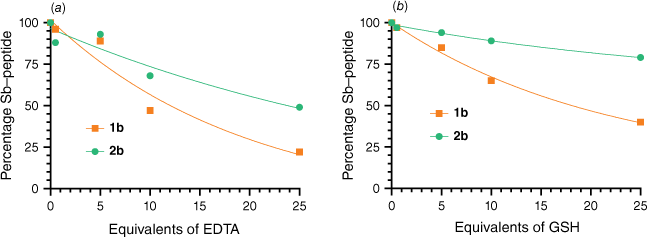
Like antimony(III), the higher pnictogen bismuth(III) has been studied for its medicinal properties.34 The high affinity of bismuth(III) towards thiolates has been previously demonstrated for small molecules as well as peptides.28–31,35,36 Therefore, we were keen to assess whether bicycles 1b and 2b would withstand increasing concentrations of bismuth(III) from gastrodenol (bismuth tripotassium dicitrate) (Fig. 3). Remarkably, we observed that only one equivalent of gastrodenol is sufficient to replace >80% of antimony(III) in 1b and 2b by bismuth(III) (Fig. 3a, c). A further increase in the gastrodenol concentration led to >90% conversion into the peptide–bismuth bicycles (1c and 2c respectively, Table 1, Fig. 3b, d). We attribute this observation to the higher thiophilicity of bismuth(III) in comparison to that of antimony(III),37 resulting in a larger stability constant of the Bi(SR)3 complex. As the bismuth–antimony competition experiments were conducted within minutes of incubation, we note that the kinetics of peptide ligand exchanges between both metals are rather fast.
Conversion of peptide–SbIII bicycles into peptide–BiIII bicycles using gastrodenol in aqueous buffer. Formation of peptide–BiIII bicycles 1c (b) and 2c (d) with increasing equivalents of gastrodenol. HPLC (254 nm) overlay and mass spectra of (a) 1b (orange) and 1c (maroon), and (c) 2b (green) and 2c (dark green).
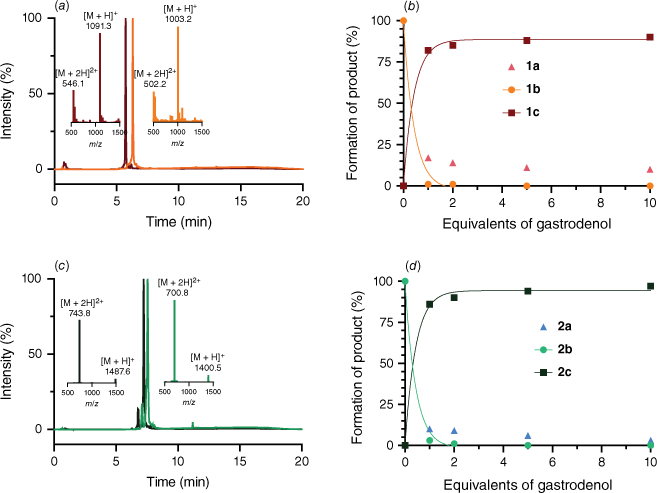
Conclusion
Our study introduces peptide–antimony bicycles as an additional class of single-element centred bicyclic peptides. This expands the repertoire of single atom ‘scaffolds’ for constrained peptide synthesis from arsenic(III) and bismuth(III) to antimony(III). These metal(loid)–peptide ‘scaffolds’ exhibit unique properties due to the combination of the stability and structural diversity of constrained peptides coupled with the unique characteristics of the metal(loid) in the centre. In situ generated SbIII-centred bicycles are considerably resistant to degradation in the presence of glutathione at physiological concentrations or EDTA. The substitution of antimony by bismuth in competition experiments supports the case of peptide–bismuth bicycles as the most promising class. As opposed to arsenic and antimony, bismuth is not generally known for its toxicity.38,39 In the future, it could be intriguing to investigate how these peptides affect target specificity and bioavailability. Overall, our study underscores the untapped potential of elements rarely used in chemical biology, which we use here to advance the synthesis of peptide macrocycles.
Experimental
Materials
Chemicals were purchased from Sigma–Aldrich (USA), AK Scientific (USA), Ambeed (USA) or ChemSupply (Australia). Peptides were purchased from Mimotopes (Australia) or produced in-house as previously described.30 Ultrapure water was obtained using a Milli-Q water purification system (Millipore, USA).
Peptide bicyclisation
The peptide (1 mM, 1a–7a) in aqueous buffer (20-mM tris(hydroxymethyl)aminomethane hydrochloride, Tris-HCl; 2-mM tris(2-carboxyethyl)phosphine, TCEP; pH 7.4) was exposed to 3 equiv. of antimony trichloride (SbCl3) from a concentrated DMSO stock (50 mM). The reaction mixture was vortexed briefly and centrifuged (3000g, 2 min) to give the corresponding peptide–antimony bicycle 1b–7b in the supernatant.
Bicyclic peptide analysis
The identity of the peptide–antimony bicycles was confirmed by high-resolution electrospray ionisation mass spectrometry (Orbitrap Elite, Thermo Fisher Scientific, USA) and liquid chromatography–mass spectrometry (LCMS System 1260/6120, Agilent, USA) at 254 nm using a reversed-phase column (Eclipse XDB-C18, 2.1 × 50 mm, 1.8 μm, Agilent, USA) held at 30°C. All chromatograms, gradients and mass spectra for bicyclic peptides 1b–7b are supplied in Supplementary Fig. S1 and S2 and Tables S1 and S2. Expected protein masses and isotope patterns were calculated with enviPat Web (Eawag, Switzerland).40
Competition assays
The antimony-uptake reaction was performed with peptides 1a and 2a as per the previously described protocol to yield ≥95% of antimony bicycles 1b and 2b. EDTA (50 mM), GSH (50 mM) and gastrodenol (10 mM) stocks were prepared in aqueous buffer (20-mM Tris-HCl, 2-mM TCEP, pH 7.4). The concentration of 1b and 2b for all three competition assays was 0.5 mM, diluted from 1-mM stocks. EDTA and GSH competitions were performed at 0.5, 5, 10 and 25 equiv. of the peptide concentration (0.5 mM). Antimony bicycles 1b and 2b were incubated in the same buffer in the presence of increasing equivalents of EDTA and GSH for 1 h at 25°C. For the BiIII competition, 1, 2, 5 and 10 equiv. of gastrodenol were added to the peptide and vortexed briefly at 25°C. Samples were analysed using high-resolution electrospray ionisation mass spectrometry (Orbitrap Elite, ThermoFisher Scientific, USA). Graphs were plotted in GraphPad Prism 10 (ver. 10.2.3, Dotmatics, USA) using a one-phase decay model under non-linear regression.
Data availability
The article and Supplementary material contain all data supporting the findings of this study.
Declaration of funding
Christoph Nitsche received an Australian Research Council grant (DP230100079) and Future Fellowship (FT220100010).
Acknowledgements
Christoph Nitsche thanks the Australian Research Council for a Future Fellowship and a Discovery Project. Lani J. Davies thanks Medical Advances Without Animals for a PhD Scholarship. Abhishek P. Patel thanks the Australian National University (ANU) for a Future Research Talent Award India. The authors thank Prof. Anthony Hill (ANU) for providing a sample of SbCl3. The authors are thankful for the ANU–CSIRO Publishing Read and Publish agreement that facilitated Open Access publication of this paper. Assoc. Prof. Christoph Nitsche was awarded the 2023 Rennie Memorial Medal of the RACI.
References
1 Sharma K, Sharma KK, Sharma A, Jain R. Peptide-based drug discovery: current status and recent advances. Drug Discov Today 2023; 28: 103464.
| Crossref | Google Scholar | PubMed |
2 Lubell WD. Peptide-based drug development. Biomedicines 2022; 10: 2037.
| Crossref | Google Scholar | PubMed |
3 Muttenthaler M, King GF, Adams DJ, Alewood PF. Trends in peptide drug discovery. Nat Rev Drug Discov 2021; 20: 309-325.
| Crossref | Google Scholar | PubMed |
4 Erak M, Bellmann-Sickert K, Els-Heindl S, Beck-Sickinger AG. Peptide chemistry toolbox – transforming natural peptides into peptide therapeutics. Bioorg Med Chem 2018; 26: 2759-2765.
| Crossref | Google Scholar | PubMed |
5 Ji X, Nielsen AL, Heinis C. Cyclic peptides for drug development. Angew Chem Int Ed 2024; 63: e202308251.
| Crossref | Google Scholar | PubMed |
6 Lau YH, de Andrade P, Wu Y, Spring DR. Peptide stapling techniques based on different macrocyclisation chemistries. Chem Soc Rev 2015; 44: 91-102.
| Crossref | Google Scholar | PubMed |
7 Ullrich S, Nitsche C. Bicyclic peptides: paving the road for therapeutics of the future. Pept Sci 2024; 116: e24326.
| Crossref | Google Scholar |
8 Baeriswyl V, Heinis C. Polycyclic peptide therapeutics. ChemMedChem 2013; 8: 377-384.
| Crossref | Google Scholar | PubMed |
9 Vinogradov AA, Yin Y, Suga H. Macrocyclic peptides as drug candidates: recent progress and remaining challenges. J Am Chem Soc 2019; 141: 4167-4181.
| Crossref | Google Scholar | PubMed |
10 Jing X, Jin K. A gold mine for drug discovery: strategies to develop cyclic peptides into therapies. Med Res Rev 2020; 40: 753-810.
| Crossref | Google Scholar | PubMed |
11 Zhang H, Chen S. Cyclic peptide drugs approved in the last two decades (2001–2021). RSC Chem Biol 2022; 3: 18-31.
| Crossref | Google Scholar | PubMed |
12 Morrison C. Constrained peptides’ time to shine? Nat Rev Drug Discov 2018; 17: 531-533.
| Crossref | Google Scholar | PubMed |
13 Passioura T, Katoh T, Goto Y, Suga H. Selection-based discovery of druglike macrocyclic peptides. Annu Rev Biochem 2014; 83: 727-752.
| Crossref | Google Scholar | PubMed |
14 Sohrabi C, Foster A, Tavassoli A. Methods for generating and screening libraries of genetically encoded cyclic peptides in drug discovery. Nat Rev Chem 2020; 4: 90-101.
| Crossref | Google Scholar | PubMed |
15 Alleyne C, Amin RP, Bhatt B, Bianchi E, Blain JC, Boyer N, et al. Series of novel and highly potent cyclic peptide PCSK9 inhibitors derived from an mRNA display screen and optimized via structure-based design. J Med Chem 2020; 63: 13796-13824.
| Crossref | Google Scholar | PubMed |
16 Tucker TJ, Embrey MW, Alleyne C, Amin RP, Bass A, Bhatt B, et al. A series of novel, highly potent, and orally bioavailable next-generation tricyclic peptide PCSK9 inhibitors. J Med Chem 2021; 64: 16770-16800.
| Crossref | Google Scholar | PubMed |
17 Kusumoto Y, Hayashi K, Sato S, Yamada T, Kozono I, Nakata Z, et al. Highly potent and oral macrocyclic peptides as a HIV-1 protease inhibitor: mRNA display-derived hit-to-lead optimization. ACS Med Chem Lett 2022; 13: 1634-1641.
| Crossref | Google Scholar | PubMed |
18 Sawyer TK, Biswas K. Peptide drug discovery raison d’etre: engineering mindset, design rules and screening tools. In: SV Ghodge, K Biswas, AA Golosov, editors. Approaching the Next Inflection in Peptide Therapeutics: Attaining Cell Permeability and Oral Bioavailability; ACS Publications; 2022. pp. 1–25. 10.1021/bk-2022-1417.ch001
19 You S, McIntyre G, Passioura T. The coming of age of cyclic peptide drugs: an update on discovery technologies. Expert Opin Drug Discov 2024; 19: 961-973.
| Crossref | Google Scholar | PubMed |
20 He J, Ghosh P, Nitsche C. Biocompatible strategies for peptide macrocyclisation. Chem Sci 2024; 15: 2300-2322.
| Crossref | Google Scholar | PubMed |
21 Rhodes CA, Pei D. Bicyclic peptides as next‐generation therapeutics. Chem Eur J 2017; 23: 12690-12703.
| Crossref | Google Scholar | PubMed |
22 Thombare VJ, Hutton CA. Bridged bicyclic peptides: structure and function. Pept Sci 2018; 110: e24057.
| Crossref | Google Scholar |
23 Ahangarzadeh S, Kanafi MM, Hosseinzadeh S, Mokhtarzadeh A, Barati M, Ranjbari J, et al. Bicyclic peptides: types, synthesis and applications. Drug Discov Today 2019; 24: 1311-1319.
| Crossref | Google Scholar | PubMed |
24 Feng D, Liu L, Shi Y, Du P, Xu S, Zhu Z, et al. Current development of bicyclic peptides. Chin Chem Lett 2023; 34: 108026.
| Crossref | Google Scholar |
25 Heinis C, Winter G. Encoded libraries of chemically modified peptides. Curr Opin Chem Biol 2015; 26: 89-98.
| Crossref | Google Scholar | PubMed |
26 Bechtler C, Lamers C. Macrocyclization strategies for cyclic peptides and peptidomimetics. RSC Med Chem 2021; 12: 1325-1351.
| Crossref | Google Scholar | PubMed |
27 Deyle K, Kong X-D, Heinis C. Phage selection of cyclic peptides for application in research and drug development. Acc Chem Res 2017; 50: 1866-1874.
| Crossref | Google Scholar | PubMed |
28 Voss S, Rademann J, Nitsche C. Peptide–bismuth bicycles: in situ access to stable constrained peptides with superior bioactivity. Angew Chem Int Ed 2022; 61: e202113857.
| Crossref | Google Scholar | PubMed |
29 Voss S, Adair LD, Achazi K, Kim H, Bergemann S, Bartenschlager R, et al. Cell‐penetrating peptide‐bismuth bicycles. Angew Chem Int Ed 2024; 63: e202318615.
| Crossref | Google Scholar | PubMed |
30 Ullrich S, Somathilake U, Shang M, Nitsche C. Phage-encoded bismuth bicycles enable instant access to targeted bioactive peptides. Commun Chem 2024; 7: 143.
| Crossref | Google Scholar | PubMed |
31 He R-N, Zhang M-J, Dai B, Kong X-D. Selection of peptide–bismuth bicycles using phage display. ACS Chem Biol 2024; 19: 1040-1044.
| Crossref | Google Scholar | PubMed |
32 Baba SP, Bhatnagar A. Role of thiols in oxidative stress. Curr Opin Toxicol 2018; 7: 133-139.
| Crossref | Google Scholar | PubMed |
33 Forman HJ, Zhang H, Rinna A. Glutathione: overview of its protective roles, measurement, and biosynthesis. Mol Asp Med 2009; 30: 1-12.
| Crossref | Google Scholar | PubMed |
34 Ge R, Sun H. Bioinorganic chemistry of bismuth and antimony: target sites of metallodrugs. Acc Chem Res 2007; 40: 267-274.
| Crossref | Google Scholar | PubMed |
35 Adeyemi JO, Onwudiwe DC. Chemistry and some biological potential of bismuth and antimony dithiocarbamate complexes. Molecules 2020; 25: 305.
| Crossref | Google Scholar | PubMed |
36 Sadler PJ, Sun H, Li H. Bismuth(III) complexes of the tripeptide glutathione. Chem Eur J 2006; 2: 701-708.
| Crossref | Google Scholar |
37 Kepp KP. A quantitative scale of oxophilicity and thiophilicity. Inorg Chem 2016; 55: 9461-9470.
| Crossref | Google Scholar | PubMed |
38 Gonçalves Â, Matias M, Salvador JAR, Silvestre S. Bioactive bismuth compounds: Is their toxicity a barrier to therapeutic use? Int J Mol Sci 2024; 25: 1600.
| Crossref | Google Scholar | PubMed |
39 Tillman LA, Drake FM, Dixon JS, Wood JR. Safety of bismuth in the treatment of gastrointestinal diseases. Aliment Pharmacol Ther 1996; 10: 459-467.
| Crossref | Google Scholar | PubMed |
40 Loos M, Gerber C, Corona F, Hollender J, Singer H. Accelerated isotope fine structure calculation using pruned transition trees. Anal Chem 2015; 87: 5738-5744.
| Crossref | Google Scholar | PubMed |