Identifying limitations for invasion: the effect of phosphorus availability on the growth of the non-native tree, Tipuana tipu
Melinda S. Trudgen



A School of Biological Sciences, The University of Western Australia, 35 Stirling Highway, Crawley, WA 6009, Australia.
B CSIRO Health and Biosecurity, 147 Underwood Avenue, Floreat, WA 6016, Australia.
C The Western Australian Biodiversity Science Institute, 133 St Georges Terrace, Perth, WA 6000, Australia.
Australian Journal of Botany 71(6) 275-285 https://doi.org/10.1071/BT22061
Submitted: 3 June 2022 Accepted: 5 April 2023 Published: 9 May 2023
© 2023 The Author(s) (or their employer(s)). Published by CSIRO Publishing. This is an open access article distributed under the Creative Commons Attribution-NonCommercial 4.0 International License (CC BY-NC)
Abstract
Context: Despite being a crucial factor in plant growth and fitness, the nutritional requirements of non-native invasive plants are poorly understood and rarely considered when assessing invasion risk; yet, they are particularly relevant in many parts of the world with nutrient-poor soils.
Aims: We investigated the growth response of a native South American tree species (Tipuana tipu), to soil concentrations of phosphorus (P). T. tipu is widely introduced in some regions of western Australia and South Africa, and we aimed to determine whether soil P availability constrains establishment, naturalisation or invasion of the species.
Methods: We grew T. tipu (Benth.) Kuntze (Fabaceae), a species that is invasive in some regions, from seed in a glasshouse. All treatments were supplied baseline nutrients, and P from 0 to 640 μg P g−1 dry substrate. Plant height and the number of mature leaves were recorded regularly. Plant biomass, P, and nitrogen (N) concentrations were analysed following destructive harvest.
Key results: Phosphorus availability had a significant effect on all measured aspects of plant growth. Seed P resources were sufficient to support growth for about 7 weeks, with plants at very low soil P availability (≤5 μg P g−1 dry soil) unable to sustain growth beyond that time. P-toxicity symptoms were observed when substrate P exceeded optimum concentrations (40–160 μg P g−1 dry soil).
Conclusions: Growth of young T. tipu seedlings was very slow at very low soil P concentrations. Under these conditions, seeds may germinate, and seedlings may survive for a short time, but self-sustaining populations are unlikely to be established. Our study adds to a growing body of research that shows that nutrient requirements of introduced plants and soil nutrient availability influence invasion risk and should be considered in risk assessments for managing species invasions at the landscape level.
Keywords: non-native plants, nutrient use, plant growth, plant nutrient requirements, seedlings, soil phosphorus, species invasions, Tipuana tipu.
Introduction
Non-native invasive plants are a serious conservation issue, and have a range of negative impacts on native species, communities and ecosystems, as well as on agricultural systems and urban environments (Didham et al. 2005; Vilà et al. 2011; Castro-Díez et al. 2019). In agricultural systems, invasive plants may be a direct threat to crop growth and animal production, or an indirect threat as a vector for pests and diseases (Groves et al. 2003). Non-native plants form a significant component of urban flora in many cities, providing a pool of species with the potential to naturalise or become invasive, and the horticultural industry provides a pathway for plants to move far beyond their natural range (Reichard and White 2001; Kowarik 2011; Pergl et al. 2016). The diversity of introduced plant communities varies widely among cities, with temperature being a strong physical driver of plant distribution because watering can compensate for the natural precipitation requirements of plants. Human modification of the landscape, both temporary and enduring, may relax the limitations that biotic and abiotic factors place on plant distributions (Kendal et al. 2012). The progression of introduced species along the invasion pathway (sensu Blackburn et al. 2011) is defined by the interaction of the following three primary components: biotic interactions, abiotic suitability and the capacity of the species for movement (Soberón and Nakamura 2009). Once a species is established in a region, it may be further transported, intentionally or unintentionally, by humans, with unintentional transport occurring via a variety of vectors, including on vehicles, in canals, or on footwear and clothing (von der Lippe and Kowarik 2007; Säumel and Kowarik 2010; Ansong and Pickering 2014).
The majority of research on the constraints of species distributions and biotic invasions has focused on the climatic suitability of a region (e.g. Thuiller et al. 2005; Webber et al. 2011; Ghosh et al. 2016), with little attention paid to other factors that influence the distribution of non-native invasive species. The focus on climate, and the use of climatic modelling for national-level management programs, has been justified by the predictive capacity of well calibrated climate-based species-distribution models (Peterson et al. 2011). Other range-defining factors such as biotic interactions and nutrient availability can vary considerably across time and within a given landscape, and thus are rarely informative for identifying range limitations that can be manipulated via management. The nutrient requirements of non-native invasive species are an important, but rarely studied, aspect of the invasion pathway for plants (Pyšek and Richardson 2007). However, nutrient availability, and nutrient-use efficiency are factors that can significantly change the risk of biotic invasions and any subsequent impacts. It is, therefore, important to understand how nutrient availability and nutrient-use efficiency influence plant invasion success, if we are to generate robust plans for managing non-native species.
Although relatively little work has directly investigated nutrient use by invasive species, there is a broad body of research that has attempted to define traits that are associated with invasiveness (sensu Blackburn et al. 2011), with Pyšek and Richardson (2007) providing an excellent overview of the main methodologies and outcomes. They summarise a variety of plant traits associated with invasiveness such as growth form (invasive species tend to be perennial), plant height (invasive species tend to be taller) and clonality or vegetative reproduction. Plasticity to nutrient availability appears to influence invasiveness; however, there are insufficient studies to draw robust conclusions, and invasive traits are likely habitat and stage specific (Pyšek and Richardson 2007). Some studies have associated invasiveness with phosphorus (P) or nitrogen (N) enrichment (Riley and Banks 1996; Weiss 1999; Pasari et al. 2014), and showed that invasive species have faster relative growth rates and/or competitive ability with greater nutrient availability (Huenneke et al. 1990; Witkowski 1991; Burns 2004; Eskelinen and Harrison 2014). Funk and Vitousek (2007) found that non-native invasive species have a high resource-use efficiency (carbon assimilation per unit of resource) across a range of soil nutrient concentrations, and warned that maintenance of low soil nutrient concentrations is insufficient to prevent plant invasion. It is clear that the nutrient requirements of invasive plants are likely to be one of several factors (including climatic conditions) that interact to affect the competitive ability of invasive plants (Witkowski 1994; Seabloom et al. 2003; Suding et al. 2004).
Regions of the world with low soil nutrient availability provide an opportunity to examine the role of soil nutrient limitation on the successful establishment and invasion of introduced species. The Western Cape of South Africa and south-western Australia have among the lowest concentrations of soil P and N in the world (Lambers et al. 2010). In these regions, soil nutrient concentrations may play a major role in impeding the progression of non-native species along the invasion pathway (Witkowski 1994; Stock and Verboom 2012). Two of the dominant non-native invasive species in the south-western Cape of South Africa, Acacia saligna (Labill.) H.L.Wendl. and Acacia cyclops A.Cunn. ex G.Don (Fabaceae) (Witkowski 1994), are leguminous species with the capacity to fix atmospheric N2 via a symbiotic relationship with nodule-forming rhizobia (Craig et al. 1991). Nitrogen-fixing non-native species may be at a relative competitive advantage because their growth is unlikely to be limited by low soil N concentrations and are especially interesting for understanding how nutrients influence the invasion dynamics of introduced plants.
For the present study, we chose the invasive legume tree species Tipuana tipu (Benth.) Kuntze (Fabaceae). T. tipu is native to an area east of the Andes in southern Bolivia and northern Argentina (Brown et al. 2007; Atahuachi et al. 2012), and is invasive in some regions of Australia and South Africa (Department of the Environment and Heritage and CRC for Australian Weed Management 2003; Macdonald et al. 2003). T. tipu is widely planted as a street and garden tree (Jochner et al. 2013), with numerous cultivated locations in south-western Australia and the Western Cape in South Africa (Department of the Environment and Heritage and CRC for Australian Weed Management 2003; Macdonald et al. 2003). Despite being widely planted, T. tipu has not been documented as being invasive in regions with low concentrations of soil P (e.g. in south-western Australia, where the soil typically has ≤20–120 μg P g−1 dry soil; Lambers et al. 2010; Kooyman et al. 2017; Trudgen 2020). In contrast, the regions of south-eastern Queensland and north-eastern New South Wales, where T. tipu is invasive, have relatively high concentrations of soil P (≥100 μg P g−1 dry soil, with most of the region having ≥250 μg P g−1 dry soil; Kooyman et al. 2017). T. tipu produces root nodules, and is likely to fix atmospheric N2 symbiotically, making N deficiency unlikely (de Faria et al. 1989; Pueppke and Broughton 1999). It is therefore possible that P deficiency in T. tipu due to insufficient soil P concentration in some regions is preventing T. tipu from becoming locally invasive, but there is currently no evidence as to how the growth of T. tipu is affected by soil P concentrations.
We used T. tipu to investigate whether naturalisation and establishment of a non-native invasive tree species may be constrained by low soil P availability. To assess the impact of P soil concentrations on T. tipu, we established a glasshouse experiment to assess growth response to P supply across a wide range of P soil concentrations. We explored possible mechanisms by which soil P concentration may affect growth and infer how these responses may affect the establishment of self-supporting populations of the species.
Materials and methods
Study species
Tipuana tipu (Benth.) Kuntze (Fabaceae) is a medium–large tree, with deciduous bi-pinnate leaves, yellow flowers and one to five seeds held within a woody samara, or winged achene (Martins and Oliveira 2001; Cruz et al. 2002). Common names include Bolivian rosewood, pride of Bolivia, tipa or racehorse tree (the latter on account of its rapid growth rate). The climate in the native range varies from semi-arid to subtropical (Antezana and Navarro 2002; Brown et al. 2007). In its native range, it is a common or dominant component of several vegetation types (Navarro and Ferreira 2004).
There are multiple records of naturalisation, predominantly in countries with a Mediterranean climate (Trudgen 2020). Young seedlings have been observed at numerous locations near cultivated trees in south-western Australia; however, the species is not known to have naturalised outside urban environments in this region (Trudgen 2020). The invasion-pathway framework of Blackburn et al. (2011) was used to define invasive, and the framework of Webber and Scott (2012) to define non-native for this study.
Growing conditions
A stratified randomised-block design pot experiment was established in a glasshouse, with the intention of simulating growing conditions in south-western Australia. The experiment was conducted from October 2012 to March 2013 in a glasshouse at The University of Western Australia (31°59′S, 115°49′E), and the plants were harvested 15 weeks after sowing. In the glasshouse, the mean monthly minimum temperature was between 14.3°C and 19.7°C, and the mean monthly maximum temperature was between 29.1°C and 32.0°C. Coarse quartz river sand, sieved to 2 mm, was used as the growth medium because (a) naturally occurring soils in south-western Australia tend to be dominated by sand (Lambers et al. 2010), and (b) the low nutrient concentrations applied in this study are not possible to control in soils with high organic matter. The soil was washed with tap water and oven dried prior to use, and had <2 μg P g−1 dry soil prior to nutrients being added to the soil. Nutrients (Table 1; consistent with Pearse et al. 2006; Pang et al. 2010; Suriyagoda et al. 2010) were mixed by a cement mixer prior to about 3 kg of the mixed sand being added to each of 72 pots. Pots were 125 mm × 210 mm white PVC pipe, with 1 mm flyscreen mesh taped across the bottom. To assist aeration, the pots had a v-shaped plastic frame positioned between the pot and the drip tray. Phosphorus was added to the sand in each pot (as dissolved KH2PO4, with KCl at an appropriate rate to balance K across the treatments) at one of the eight treatment levels of 0, 5, 10, 40, 80, 160, 320, 640 μg P g−1 dry soil and the pots were watered every 1–3 days to maintain 70% field capacity. The dried fruit (samaras) were X-rayed (Faxitron MX-20 Specimen Radiography System, Tucson, AZ, USA) prior to sowing, to determine the number of viable seeds per samara, with viability assessed visually on the basis of the size, shape and fill of the seed(s) within the samara. The samaras used for the experiment were randomly chosen from among the samaras with two viable seeds, and the wings of the samara were removed with scissors prior to planting. Two samaras (and therefore a total of four seeds, visually similar by X-ray) were sown per pot. Germination was recorded daily, and plants were thinned to one plant per pot 3 weeks after sowing (as per Poorter and Garnier 1996; Poorter et al. 2012).
Nutrient | Concentration (μg g−1 dry soil) |
---|---|
B | 0.12 |
Ca | 57.3 |
Cl | 3.0 |
Co | 0.084 |
Cu | 0.50 |
Fe | 5.50 |
Mg | 10.0 |
Mn | 4.0 |
Mo | 0.44 |
N | 40 |
Na | 0.20 |
S | 60.9 |
Zn | 2.0 |
Plant measurements
Non-destructive measurements (stem height and leaf number, and observing if the leaf at each leaf node was immature, mature or senesced) were recorded on the day of seedling thinning, and remeasured three times (at 4-weekly intervals) thereafter, including on the day of the final destructive harvest. Senesced leaves (if any) from each plant were collected and oven dried immediately, with all senesced leaves from each plant combined at the end of the experiment to allow resorption of nutrients to be measured. In the final destructive harvest, each plant was severed at ground level. Leaves were removed from the stem and roots were gently washed free of the sand by using a light stream of water and a 2 mm sieve plate to prevent fine roots from being washed away. Nodules were counted, removed from the roots and stored for oven drying. The stem was dried immediately, and the leaves stored at 2°C until they were scanned. Fresh leaves were scanned (RGB TIFF; 300 dpi) on an Epson 750 flatbed scanner and leaf area was calculated using the LEAF GUI (Price 2012). All plant material was dried at 68°C for a minimum of 7 days to obtain dry weight.
Chemical analyses
Plant materials (leaves, stems, roots, senesced leaves and thinned germinants) were individually ground using an electric coffee grinder before subsampling for chemical analysis. Nodules were excluded from analyses because of a lack of material across treatment groups. Thinned germinants were used as a proxy for seed reserves, because it was not possible to safely extract individual seeds from the woody samaras. Phosphorus was analysed (with a precision of 0.01 mg g−1) following the protocol for small samples in Fourqurean et al. (1992). Nitrogen was analysed using the Leco Total Nitrogen method (with a precision of 0.1% by weight) for the Leco Combustion analyser (TruSpec CN Carbon Nitrogen Determinator Instruction Manual Software, ver. 1.6 x., Part No. 200-288, March 2006). Phosphorus-resorption efficiency (PRE) and nitrogen-resorption efficiency (NRE) were calculated as the change in nutrient concentration between mature green and fully senesced leaves (nutrient concentration in green leaves minus the nutrient concentration in senesced leaves, all divided by the nutrient concentration in green leaves and multiplied by 100, as per Killingbeck 1996). Values for N and P in the fully senesced leaves are equivalent to values of nutrient-resorption proficiency (as per Killingbeck 1996).
Data analysis
Non-destructive measurements were analysed using repeated-measures ANOVA and Tukey’s HSD test (P < 0.05). Harvest results and chemical analysis results were analysed using two-way ANOVA and Tukey’s HSD test (P < 0.05). Five plants had atypical growth patterns (branching owing to thrip damage, or unusually early flower production) and were excluded from analyses. All analyses were undertaken in R ver. 3.5.1 (R Core Team 2018) and the broom, Cairo, car, emmeans, facetscales, lattice, modelr, multcompView, nlme, rcompanion and tidyverse packages (Sarkar 2008; Graves et al. 2015; Wickham 2017, 2019; Fox and Wesiberg 2019; Lenth 2019; Mangiafico 2019; Oller Moreno 2019; Pinheiro et al. 2019; Robinson and Hayes 2019; Urbanek and Horner 2019).
Results
Vegetative growth over the experimental period showed that the P treatments had a significant (P < 0.0001) effect on total plant dry mass and leaf area (Fig. 1a, b). At very low soil P concentrations (≤5 μg P g−1 dry soil), the plants had very low dry mass and small leaf areas. The majority of the dry mass of these plants was root dry mass (≥60%). As P in the soil was increased (≥10 μg P g−1 dry soil), the dry mass and leaf area of the plants increased concomitantly, and then decreased again at very high concentrations of soil P (≥160 μg P g−1 dry soil). Maximum dry mass occurred at 40–80 μg P g−1 dry soil and was 7–18 times greater than the dry mass recorded at very low soil P concentrations (≤5 μg P g−1 dry soil). Plants in treatments with soil P from 10 to 160 μg P g−1 dry soil had roughly equal aboveground and belowground biomass (42–54% belowground biomass), whereas plants at very high soil P of ≥320 μg P g−1 dry soil had relatively low root biomass (<40%). Nodule formation occurred at all soil P treatment levels (Fig. 1c), but the nodule mass was less than 2% of dry mass in all treatments, except at 80 μg P g−1 dry soil. Plants in this treatment group had significantly greater nodule biomass per unit total plant biomass than in any other treatment group (P = 0.0140), and nodules accounted for 6.5% of the biomass of these plants.
(a) Dry mass, (b) leaf area and (c) nodule dry mass per unit total dry mass for Tipuana tipu grown for 15 weeks in washed river sand supplied with eight concentrations of phosphorus (P) in the form of KH2PO4, ranging from 0 to 640 μg P g−1 dry soil. Data are presented as means ± s.e.m. Different letters above the bars indicate significant differences among the P treatments, according to two-way ANOVA and Tukey’s HSD test (P < 0.05).
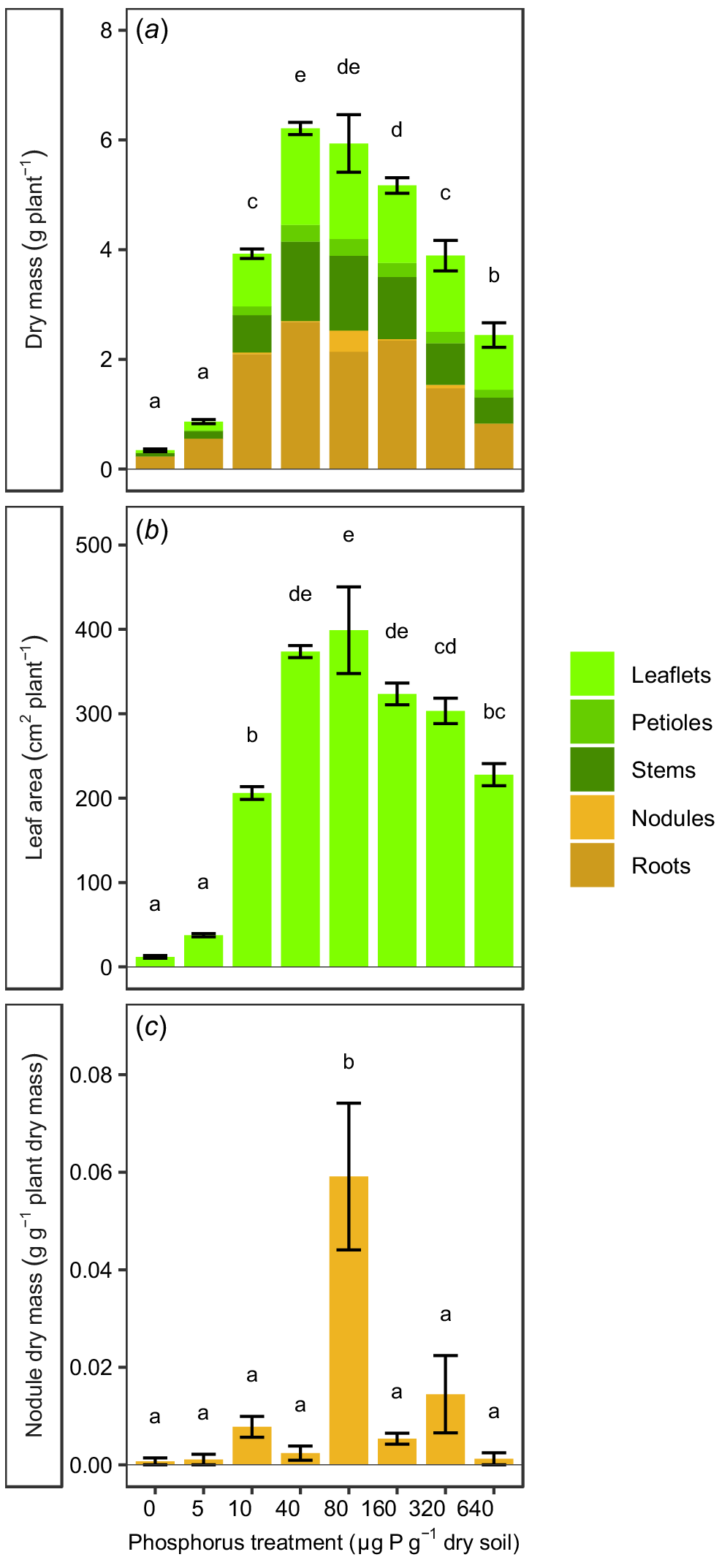
Non-destructive measurements also showed that soil P concentration had a significant (P < 0.0001) effect on the height and number of mature leaves of each plant over time (Fig. 2). At very low soil P concentrations (≤5 μg P g−1 dry soil; Fig. 2a, b, i, j), the overall height of plants (≤20 cm) was significantly shorter than in other treatments, and the number of mature leaves increased at 7 weeks before subsequently decreasing by 11 and 15 weeks. These plants were in very poor health, and the experiment was terminated before they senesced. Plants at 10 μg P g−1 dry soil were taller, although still significantly shorter than were plants at moderate to high soil P concentration (40–160 μg P g−1 dry soil), and whereas the number of mature leaves had increased after 7 and 11 weeks, the increase in the number of leaves from 11 to 15 weeks was small (Fig. 2c, k). Plants at moderate to high soil P concentrations (40–160 μg P g−1 dry soil; Fig. 2d–f, l–n) had the fastest growth in height and were significantly taller (50–60 cm) than those in other treatments, and also showed a rapid increase in the number of mature leaves. Growth decreased at P concentrations of ≥320 μg P g−1 dry soil, and the final height was significantly less than that of the plants at more moderate soil P concentrations (Fig. 2g). The number of mature leaves at 320 μg P g−1 dry soil was not significantly different from the number at 160 μg P g−1 dry soil (Fig. 2n, o); however, at the highest P treatment (640 μg P g−1 dry soil), the number of mature leaves was significantly less than at more moderate P concentrations and was not significantly different from the number of leaves at 10 μg P g−1 dry soil (Fig. 2k, p). Like the plants at 10 μg P g−1 dry soil, the number of mature leaves on the plants at 640 μg P g−1 dry soil increased by 7 and 11 weeks, but the increase in the number of leaves from 11 to 15 weeks was small.
(a–h) Height and (i–p) number of mature leaves per plant of Tipuana tipu grown for 15 weeks in washed river sand supplied with eight levels of phosphorus (P) in the form of KH2PO4, ranging from 0 to 640 μg P g−1 dry soil. Data are presented as means ± s.e.m. Different letters indicate significant differences among the P treatments, for each parameter, according to repeated measures ANOVA and Tukey’s HSD test (P < 0.05).
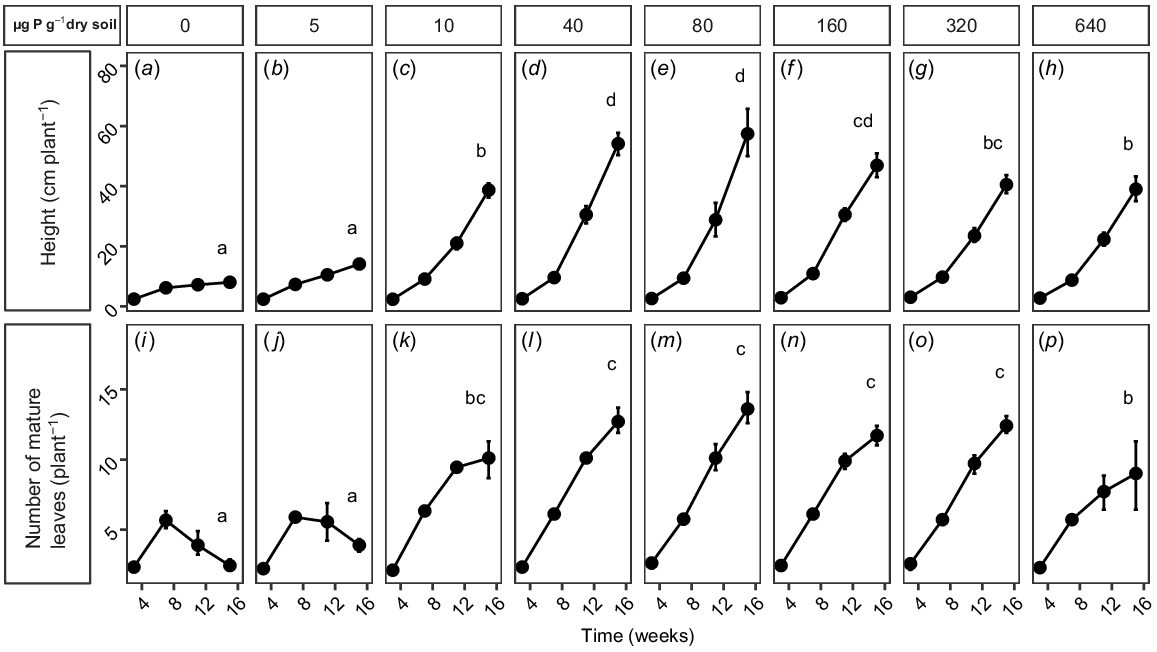
Nutrient analysis after 15 weeks of plant growth showed significant differences in leaf P concentrations among treatments, with a positive relationship between P concentration in the plant material and soil P concentration (P < 0.0001; Fig. 3g). The concentration of P in the leaves was not significantly different from 0 to 80 μg P g−1 dry soil, and then increased with an increasing soil P concentration, with leaves from the highest soil P treatment (640 μg P g−1 dry soil) having significantly higher P concentrations than those in any other treatment (Fig. 3g). The concentration of P in the senesced leaves showed the same pattern of being initially stable and then increasing with an increasing soil P concentration (Fig. 3i). The PRE was between 34% and 67% for plants grown at 0–10 μg P g−1 dry soil and 49% for plants grown at 80 μg P g−1 dry soil; for plants grown at all other soil P concentrations, the PRE was below 0% (Fig. 3i). The stems showed a positive relationship between soil and plant P concentrations, with the material from the highest soil P treatment (640 μg P g−1 dry soil) having the highest P concentrations (Fig. 3j). All plant materials apart from the senesced leaves from the highest soil P treatment (640 μg P g−1 dry soil) had lower concentrations of P than was found in the germinants (16.6 ± 0.3 mg g−1; Fig. 3f–j).
(a–e) Nitrogen (N) and (f–j) phosphorus (P) concentration of Tipuana tipu germinants (as a surrogate for seed concentrations), and of seedlings grown for 15 weeks in washed river sand supplied with eight concentrations of P in the form of KH2PO4, ranging from 0 to 640 μg P g−1 dry soil. Data are presented as means ± s.e.m. DM, dry mass. Different letters above the bars indicate significant differences among the P treatments, for each parameter, according to two-way ANOVA and Tukey’s HSD test (P < 0.05). Numbers above the bars in the senesced leaves panels indicate nitrogen-resorption efficiency and phosphorus-resorption efficiency (the change in nutrient concentration from green leaves to senesced leaves) respectively.
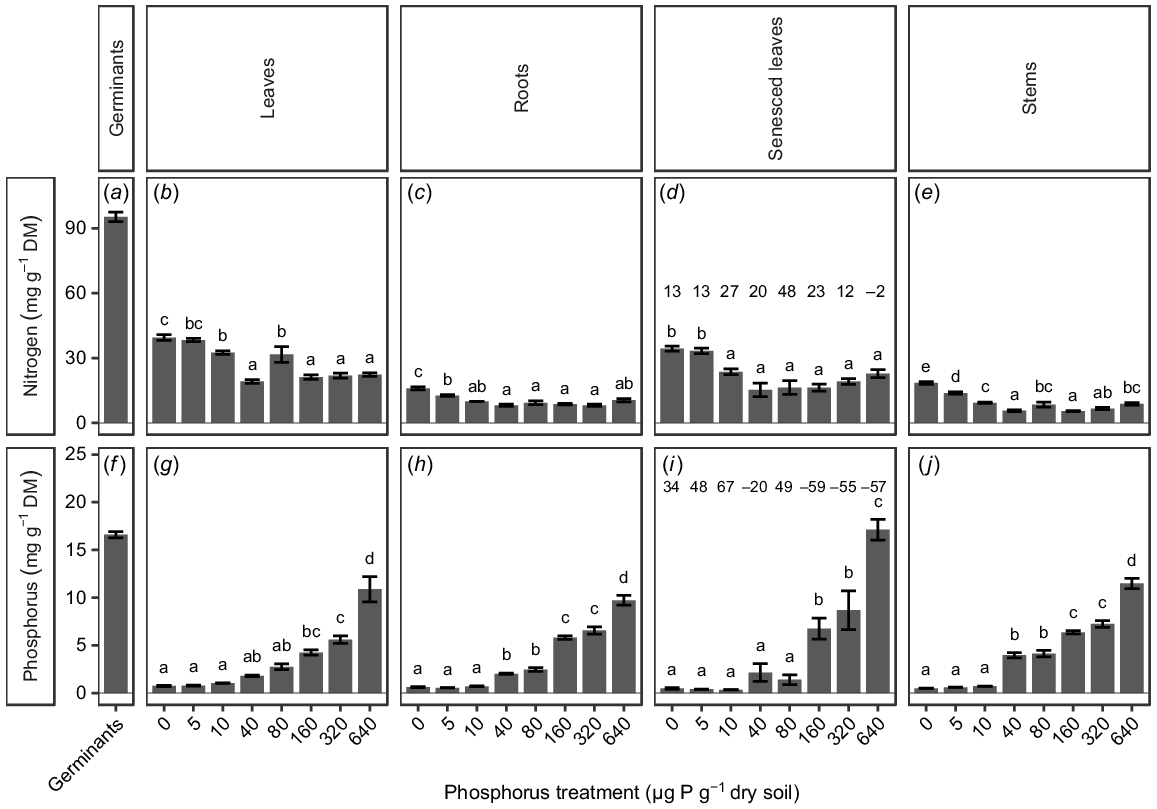
Nitrogen concentrations in plant material showed less distinct trends than those observed for P; however, there were significant differences among treatment groups, with a general negative relationship between N and soil P concentration (P < 0.0001; Fig. 3). The N concentrations in leaf and stem material from plants at 80 μg P g−1 dry soil were significantly greater than those from plants grown in the soil P treatments immediately above or below this treatment (Fig. 3b, e). The NRE varied from 12% to 27%, except for a higher value of 48% for plants grown at 80 μg P g−1 dry soil and −2% for plants grown at 640 μg P g−1 dry soil (Fig. 3d). All seedling material had lower concentrations of N than was found in the germinants (Fig. 3a–e).
Discussion
Plant mineral nutrition is broadly recognised for its importance to plant growth; however, it is relatively poorly understood with respect to invasive plant species. The results of this study have provided strong evidence that seedling growth of Tipuana tipu is significantly associated with soil P concentration, and suggest that progress down the invasion pathway may be constrained in regions with insufficient soil P availability.
Plant response to soil P availability
Seed P reserves are an important resource for seedling establishment, and are considered a strategy of increasing seedling survival in nutrient-poor soils (Kuo et al. 1982; Denton et al. 2007). Growth of T. tipu seedlings up to 7 weeks after sowing appeared to be driven by nutrient reserves from the seed endosperm, because plant height and the number of mature leaves per plant were consistent across treatments up to this time. Germinant P concentration of T. tipu (measured in lieu of seeds) was greater than the average concentration recorded for seeds from species adapted to the nutrient-poor soils of south-western Australia (13.2 ± 0.8; Kuo et al. 1982; Groom and Lamont 2010; Hayes et al. 2021); however, the total P content of T. tipu seeds was much lower than recorded for those seeds, owing to lower total germinant weight. This indicates that T. tipu is not adapted to relying on its seed P reserves to support a long period of establishment or survival as a seedling during periods of enduring resource limitation.
Soil nutrient availability has a strong influence on plant growth, and the T. tipu seedlings in our study showed a strong response to soil P concentration. Plants grown at very low soil P concentrations (≤5 μg P g−1 dry soil) began to show signs of P deficiency, leaf senescence, very slow growth and showed declining vigour across all replicates after 7 weeks. When these plants were harvested, it became clear that they had invested heavily in root growth, which is commonly observed in plants grown in conditions of P deficiency (e.g. Wang et al. 2015) and corresponds with a growth strategy to locate soil nutrient reserves and increase root surface area for absorption of P (Vance et al. 2003; Lambers et al. 2006). Therefore, we conclude that plants grown at ≤5 μg P g−1 dry soil had insufficient P available to sustain vigorous growth beyond approximately 7 weeks.
Plants grown at slightly higher soil P concentrations (10 μg P g−1 dry soil) were able to sustain growth for longer; however, they still appeared to exhaust their limited P supply and had signs of P deficiency after 11 weeks. Although the P concentration in senesced leaves (a measure of nutrient-resorption proficiency) of plants grown at ≤10 μg P g−1 dry soil was somewhat higher that that of plants native to the nutrient-poor soils of south-western Australia (Hayes et al. 2021), the P-remobilisation efficiency (34–67%, PRE) was similar to the lower end of P-remobilisation efficiency for those plant species (27–82%, Denton et al. 2007; approximately 20–80%, He et al. 2011). Although it is possible that some leaching occurred from the senesced leaves prior to collection, they were collected as soon as possible to minimise this possibility. Although the plants in our study grown at ≤10 μg P g−1 dry soil resorbed significant P from the leaves prior to leaf abscission, they still had significantly less P in their plant organs than did healthy T. tipu plants grown at higher soil P concentrations. Although P deficiency is uncommon in young P-rich soils, plants in our study were well supplied with N and other baseline nutrients, and P was the limiting nutrient in the river sand used in our experiment (Lambers et al. 2010).
The optimal soil P concentration for growth of T. tipu plants in our study was between 40 and 80 μg P g−1 dry soil, with plants in these treatments having the greatest dry mass, number of mature leaves and plant height. This optimal soil P concentration is much higher than that for many species adapted to soils with low P concentrations (e.g. Williams et al. 2019), and is most likely to reflect the soil conditions in the native range of southern Bolivia and northern Argentina (Brown et al. 2007; Atahuachi et al. 2012). Plants from one of the treatment groups with optimal soil P concentration (80 μg P g−1 dry soil) had a significantly higher proportion of nodules (g g−1 total biomass) than did those from the other treatment groups, and had significantly greater biomass and height than did plants from other treatments. This finding indicated that these plants were N limited rather than P limited and invested in nodule growth to access additional N supplies (Sprent and Thomas 1984). Plants in this treatment resorbed P and N from the leaves prior to senescence, and it appears likely that the greatly increased nodulation on these plants increased the plants overall demand for P, because increased N availability enhances the demand for other nutrients (Eller and Oliveira 2017; Valliere et al. 2017).
Across the treatments, foliar, stem and root P concentrations increased and PRE decreased as soil P concentration was increased, being consistent with previous studies (e.g. He et al. 2011). Foliar P concentrations were relatively high for T. tipu when compared with those for plants adapted to the nutrient-poor soils of south-western Australia (0.14–0.27 mg g−1, Denton et al. (2007); <1 mg g−1, Hayes et al. 2021); however, they were low in comparison with global foliar P concentrations for deciduous angiosperms (0.75–10.9 mg g−1 compared with 15.5 mg g−1 globally; Vergutz et al. 2012).
Plant growth of T. tipu in our study was negatively affected by surplus P availability at high soil P concentrations (≥160 μg P g−1 dry soil). Above the optimal soil P concentration range (between 40 and 80 μg P g−1 dry soil), dry mass and leaf area decreased for each increase in soil P concentration, and plants at higher soil P concentration decreased their investment in belowground growth. This growth pattern was opposed to the pattern exhibited by plants when they are P limited (Lambers et al. 2006), and suggests that the plants obtained the nutrients required for growth from a small volume of soil. We found no evidence of the plants in our study down-regulating P-uptake capacity at the higher soil P concentrations, and the P concentrations in senesced leaves were within the range of P concentrations at which P toxicity typically develops (Aiello and Graves 1996; Shane et al. 2004; Lambers 2022). The negative PRE values for plants grown at very high soil P concentrations (≥160 μg P g−1 dry soil) demonstrated that the plants were depositing P in these leaves prior to leaf senescence. Taken together, these findings provided strong evidence that the relative decrease in dry mass and leaf area of plants grown at high soil P concentrations was a result of P toxicity, rather than nutrient deficiency.
Nutrient use and invasive-species establishment
The nutritional requirements of non-native invasive species are an aspect of invasion ecology that has not been studied enough to draw out general patterns on the associated traits that lead to invasion success (Pyšek and Richardson 2007), despite the recognised importance of adequate nutrition for plant growth and survival. The establishment of seedlings is generally considered the most vulnerable developmental stage for a plant (Wilson and Witkowski 1998). Our study has demonstrated that the seedling growth of T. tipu is affected by soil P availability, and it is likely that soil P availability constrains the capacity of this introduced species to establish self-sustaining populations in regions with very low soil P concentrations (≤5 μg P g−1 dry soil). These findings add to an increasing body of evidence (e.g. Witkowski 1994; Riley and Banks 1996; Burns 2004; Eskelinen and Harrison 2014; Hu et al. 2019) that suggests that a plant’s response to nutrients should be considered when assessing the invasion risk posed by a species, and when developing suitable management plans.
In our results, T. tipu plants grown at very low soil P concentrations (≤5 μg P g−1 dry soil) were unable to sustain growth beyond 7 weeks, indicating that T. tipu will be very unlikely to survive to reach maturity, and even less likely to establish and form self-sustaining populations in regions with very low soil P concentrations, such as south-western Australia (Lambers et al. 2010). T. tipu plants grown at these very low soil P concentrations were small at the end of our study, with extremely low biomass in comparison with tree species native to the nutrient-poor soils of south-western Australia (Zhou et al. 2022). Increased height is associated with invasiveness (Pyšek and Richardson 2007) and the small stature of these plants is likely to make them vulnerable in the wild to mortality by a range of causes such as trampling, browsing, mowing, or targeted weed removal. Although we have not directly assessed competitive ability in this study, the low total biomass, short stature, and signs of P deficiency of T. tipu grown at very low soil P concentrations, in comparison with species adapted to nutrient-poor landscapes (e.g. Denton et al. 2007; He et al. 2011; Zhou et al. 2022), suggests that T. tipu has a low competitive ability against species that are adapted to low soil P concentrations. At optimal soil P concentrations (40–160 μg P g−1 dry soil), T. tipu was capable of rapid growth across a range of measures (height, biomass, number of mature leaves), and the species’ competitive ability is likely to be greater at these soil P concentrations. This contrasts with seedlings from species that are adapted to nutrient-poor soils, which often have greater biomass at very low soil P concentrations and lower biomass when grown in higher soil P concentrations (Standish et al. 2007; Williams et al. 2019). Although plants in natural conditions would be subject to a range of other factors affecting growth, we expect that, in suitable climatic conditions, soils with P concentrations in this range (40–160 μg P g−1 dry soil) may allow naturalised populations of T. tipu to establish. At quite high soil P concentration (640 μg P g−1 dry soil), plants in our study showed signs of P toxicity, which would likely prevent the establishment of naturalised populations where high soil P concentrations occur. This concentration of soil P is extremely high for nutrient-poor landscapes such as south-western Australia, although it is possible that localised nutrient pollution may occur, with riparian areas at a particular risk of accumulating excess P; however, this soil P concentration may occur in soils in other regions around the world (Stock and Verboom 2012). Areas with unnaturally high soil P concentrations as a result of nutrient enrichment are of particular interest with regard to plant invasions, because the growth of native species may be suppressed, whereas non-native species may respond to the higher soil nutrient concentrations with increased growth and, therefore, have a competitive edge in such situations (Williams et al. 2019).
The consideration of nutrient requirements in invasion management is hampered by two main limitations, namely, the lack of data on nutrient requirements for individual species, and the lack of suitably scaled and up to date soil nutrient maps (see Michael et al. 2012; Matos-Moreira et al. 2017). Even though we have been able to describe the growth response of T. tipu seedlings across a broad range of soil P availabilities, our findings remain limited by the lack of suitable soil maps (Viscarra Rossel and Bui 2016; Kooyman et al. 2017), and we have been unable to map regions where very low soil P concentrations may prevent the establishment of non-native T. tipu populations (see Michael et al. 2012). Moreover, nutrient pollution of soils can circumvent this limit to invasion risk and is perhaps most likely in urban and agricultural environments, which have a high proportion of non-native species with the potential to become invasive (Kowarik 2011). The issue of scale and currency of soil maps will, therefore, be particularly important in these environments. Until these two limitations are overcome, it will be difficult to accurately account for nutrient requirements in species risk assessments and management plans at a large scale (Viscarra Rossel and Bui 2016). Nonetheless, soil nutrients remain a critical microhabitat consideration to assess the risk of non-native plants moving down the invasion pathway, particularly for microhabitats around cities where riparian areas and nutrient pollution are more frequent in the landscape.
Conclusions
We found that the growth of young T. tipu seedlings was significantly affected by soil P concentrations, with implications for their potential capacity to become invasive, particularly in landscapes with very low soil P concentrations (≤5 μg P g−1 dry soil). The growth of young T. tipu seedlings was very slow at very low soil P concentrations, and the optimal soil P concentration range for growth was higher than that for plants adapted to low soil concentrations (e.g. He et al. 2011; Williams et al. 2019). The extremely poor vigour and growth of T. tipu plants at very low soil P concentrations, the low number of mature leaves on these plants, and the importance of height and rapid growth rates as traits of successful plant invasions, lead us to conclude that T. tipu will likely be constrained in establishing self-sustaining populations in locations with very low soil P concentrations. T. tipu plants exhibited signs of P toxicity beyond the optimal concentration of soil P, which may also constrain the invasive potential in areas with quite high soil P concentrations (≥640 μg P g−1 dry soil). Our study contributes to the growing body of evidence that soil nutrient availability and soil nutrient requirements are an important aspect to consider when contextualising plant invasions. Such insight represents an important inclusion into invasive species risk assessments and management plans, particularly at a fine scale where microhabitats are a significant aspect of the landscape.
Declaration of funding
MST was supported by an Australian Government Research Training Program (RTP) Scholarship (Australian Post-Graduate award) and the University of Western Australia top-up program. Funding for experimental costs was supported by a University of Western Australia Research Development Award to BLW.
Acknowledgements
Glasshouse assistance was provided by Rob Creasy and the University of Western Australia Plant Growth Facility staff, with additional assistance by Chris Chester, Rowena Putland, Sonja Jakob, Xing Wang, Veronica Wilson, Alice Watt and Caroline Delaisse. Charles A. Price provided assistance with calculating leaf area. Wolfgang Lewandrowski, David Merritt and Shane Turner facilitated the use of seed X-ray equipment at Kings Park and Botanic Garden.
References
Aiello AS, Graves WR (1996) Two leguminous tree species differ in growth and ion uptake in Hoagland solution: phosphorus toxicity in Amur Maackia. Journal of Plant Nutrition 19, 1061-1073.
| Crossref | Google Scholar |
Ansong M, Pickering C (2014) Weed seeds on clothing: a global review. Journal of Environmental Management 144, 203-211.
| Crossref | Google Scholar |
Antezana C, Navarro G (2002) Contribución al análisis biogeográfico y catálogo preliminar de la flora de los valles secos interandinos del centro de Bolivia. Revista Boliviana de Ecología y Conservación Ambiental 12, 3-38.
| Google Scholar |
Atahuachi M, Villalobos J, Catari JC, Serrano M (2012) Tipuana tipu (Benth.) Kuntze. In ‘Libro rojo de la flora amenazada de Bolivia. Vol. 1. Zona Andina’. (Eds G Navarro, S Arrázola, M Atahuachi, N De la Barra, M Mercado, W Ferreira, M Moraes). pp. 553–554. (Ministerio de Medio Ambiente y Agua: La Paz, Bolivia)
Blackburn TM, Pyšek P, Bacher S, Carlton JT, Duncan RP, Jarošík V, Wilson JRU, Richardson DM (2011) A proposed unified framework for biological invasions. Trends in Ecology & Evolution 26, 333-339.
| Crossref | Google Scholar |
Burns JH (2004) A comparison of invasive and non-invasive dayflowers (Commelinaceae) across experimental nutrient and water gradients. Diversity and Distributions 10, 387-397.
| Crossref | Google Scholar |
Castro-Díez P, Vaz AS, Silva JS, et al. (2019) Global effects of non-native tree species on multiple ecosystem services. Biological Reviews 94, 1477-1501.
| Crossref | Google Scholar |
Craig GF, Atkins CA, Bell DT (1991) Effect of salinity on growth of four strains of Rhizobium and their infectivity and effectiveness on two species of Acacia. Plant and Soil 133, 253-262.
| Crossref | Google Scholar |
de Faria SM, Lewis GP, Sprent JI, Sutherland JM (1989) Occurrence of nodulation in the Leguminosae. New Phytologist 111, 607-619.
| Crossref | Google Scholar |
Denton MD, Veneklaas EJ, Freimoser FM, Lambers H (2007) Banksia species (Proteaceae) from severely phosphorus-impoverished soils exhibit extreme efficiency in the use and re-mobilization of phosphorus. Plant, Cell & Environment 30, 1557-1565.
| Crossref | Google Scholar |
Didham RK, Tylianakis JM, Hutchison MA, Ewers RM, Gemmell NJ (2005) Are invasive species the drivers of ecological change? Trends in Ecology & Evolution 20, 470-474.
| Crossref | Google Scholar |
Eller CB, Oliveira RS (2017) Effects of nitrogen availability on the competitive interactions between an invasive and a native grass from Brazilian cerrado. Plant and Soil 410, 63-72.
| Crossref | Google Scholar |
Eskelinen A, Harrison S (2014) Exotic plant invasions under enhanced rainfall are constrained by soil nutrients and competition. Ecology 95, 682-692.
| Crossref | Google Scholar |
Fourqurean JW, Zieman JC, Powell GVN (1992) Phosphorus limitation of primary production in Florida Bay: evidence from C:N:P ratios of the dominant seagrass Thalassia testudinum. Limnology and Oceanography 37, 162-171.
| Crossref | Google Scholar |
Funk JL, Vitousek PM (2007) Resource-use efficiency and plant invasion in low-resource systems. Nature 446, 1079-1081.
| Crossref | Google Scholar |
Ghosh S, Zhu K, Gelfand AE, Clark JS (2016) Joint modeling of climate niches for adult and juvenile trees. Journal of Agricultural, Biological, and Environmental Statistics 21, 111-130.
| Crossref | Google Scholar |
Graves S, Piepho H-P, Selzer L, Dorai-Raj S (2015) multcompView: visualizations of paired comparisons. R package version 0.1-7. Available at https://cran.r-project.org/web/packages/multcompView/index.html
Groom PK, Lamont BB (2010) Phosphorus accumulation in Proteaceae seeds: a synthesis. Plant and Soil 334, 61-72.
| Crossref | Google Scholar |
Hayes PE, Nge FJ, Cramer MD, et al. (2021) Traits related to efficient acquisition and use of phosphorus promote diversification in Proteaceae in phosphorus-impoverished landscapes. Plant and Soil 462, 67-88.
| Crossref | Google Scholar |
He H, Bleby TM, Veneklaas EJ, Lambers H (2011) Dinitrogen-fixing Acacia species from phosphorus-impoverished soils resorb leaf phosphorus efficiently. Plant, Cell & Environment 34, 2060-2070.
| Crossref | Google Scholar |
Hu C-C, Lei Y-B, Tan Y-H, Sun X-C, Xu H, Liu C-Q, Liu X-Y (2019) Plant nitrogen and phosphorus utilization under invasive pressure in a montane ecosystem of tropical China. Journal of Ecology 107, 372-386.
| Crossref | Google Scholar |
Huenneke LF, Hamburg SP, Koide R, Mooney HA, Vitousek PM (1990) Effects of soil resources on plant invasion and community structure in Californian serpentine grassland. Ecology 71, 478-491.
| Crossref | Google Scholar |
Jochner S, Alves-Eigenheer M, Menzel A, Morellato LPC (2013) Using phenology to assess urban heat islands in tropical and temperate regions. International Journal of Climatology 33, 3141-3151.
| Crossref | Google Scholar |
Kendal D, Williams NSG, Williams KJH (2012) A cultivated environment: exploring the global distribution of plants in gardens, parks and streetscapes. Urban Ecosystems 15, 637-652.
| Crossref | Google Scholar |
Killingbeck KT (1996) Nutrients in senesced leaves: keys to the search for potential resorption and resorption proficiency. Ecology 77, 1716-1727.
| Crossref | Google Scholar |
Kooyman RM, Laffan SW, Westoby M (2017) The incidence of low phosphorus soils in Australia. Plant and Soil 412, 143-150.
| Crossref | Google Scholar |
Kowarik I (2011) Novel urban ecosystems, biodiversity, and conservation. Environmental Pollution 159, 1974-1983.
| Crossref | Google Scholar |
Kuo J, Hocking PJ, Pate JS (1982) Nutrient reserves in seeds of selected Proteaceous species from South-Western Australia. Australian Journal of Botany 30, 231-249.
| Crossref | Google Scholar |
Lambers H (2022) Phosphorus acquisition and utilization in plants. Annual Review of Plant Biology 73, 17-42.
| Crossref | Google Scholar |
Lambers H, Shane MW, Cramer MD, Pearse SJ, Veneklaas EJ (2006) Root structure and functioning for efficient acquisition of phosphorus: matching morphological and physiological traits. Annals of Botany 98, 693-713.
| Crossref | Google Scholar |
Lambers H, Brundrett MC, Raven JA, Hopper SD (2010) Plant mineral nutrition in ancient landscapes: high plant species diversity on infertile soils is linked to functional diversity for nutritional strategies. Plant and Soil 334, 11-31.
| Crossref | Google Scholar |
Lenth R (2019) emmeans: estimated marginal means, aka least-squares means. R package version 1.4.1. Available at https://cran.r-project.org/web/packages/emmeans/index.html
Mangiafico S (2019) rcompanion: functions to support extension education program evaluation. R package version 2.3.0. Available at https://cran.r-project.org/web/packages/rcompanion/index.html
Martins MAG, Oliveira DMT (2001) Morfo-anatomia e ontogênese do fruto e da semente de Tipuana tipu (Benth.) O. Kuntze (Fabaceae: Faboideae). Revista Brasileira de Botânica 24, 109-121.
| Crossref | Google Scholar |
Matos-Moreira M, Lemercier B, Dupas R, Michot D, Viaud V, Akkal-Corfini N, Louis B, Gascuel-Odoux C (2017) High-resolution mapping of soil phosphorus concentration in agricultural landscapes with readily available or detailed survey data. European Journal of Soil Science 68, 281-294.
| Crossref | Google Scholar |
Michael PJ, Yeoh PB, Scott JK (2012) Potential distribution of the Australian native Chloris truncata based on modelling both the successful and failed global introductions. PLoS ONE 7, e42140.
| Crossref | Google Scholar |
Navarro G, Ferreira W (2004) Zonas de vegetación potencial de Bolivia: una base para el análisis de vacíos de conservación. Revista Boliviana de Ecología y Conservación Ambiental 15, 1-40.
| Google Scholar |
Oller Moreno S (2019) facetscales: facet_grid with different scales per facet. R package version 0.1.0.9000. Available at http://github.com/zeehio/facetscales
Pang J, Tibbett M, Denton MD, Lambers H, Siddique KHM, Bolland MDA, Revell CK, Ryan MH (2010) Variation in seedling growth of 11 perennial legumes in response to phosphorus supply. Plant and Soil 328, 133-143.
| Crossref | Google Scholar |
Pasari JR, Hernández DL, Zavaleta ES (2014) Interactive effects of nitrogen deposition and grazing on plant species composition in a serpentine grassland. Rangeland Ecology & Management 67, 693-700.
| Crossref | Google Scholar |
Pearse SJ, Veneklaas EJ, Cawthray GR, Bolland MDA, Lambers H (2006) Carboxylate release of wheat, canola and 11 grain legume species as affected by phosphorus status. Plant and Soil 288, 127-139.
| Crossref | Google Scholar |
Pergl J, Sadlo J, Petřík P, et al. (2016) Dark side of the fence: ornamental plants as a source of wild-growing flora in the Czech Republic. Preslia 88, 163-184.
| Google Scholar |
Pinheiro J, Bates D, DebRoy S, Sarkar D, Team RC (2019) nlme: linear and nonlinear mixed effects models. R package version 3.1-141. Available at https://cran.r-project.org/web/packages/nlme/index.html
Poorter H, Garnier E (1996) Plant growth analysis: an evaluation of experimental design and computational methods. Journal of Experimental Botany 47, 1343-1351.
| Crossref | Google Scholar |
Poorter H, Fiorani F, Stitt M, et al. (2012) The art of growing plants for experimental purposes: a practical guide for the plant biologist. Functional Plant Biology 39, 821-838.
| Crossref | Google Scholar |
Pueppke SG, Broughton WJ (1999) Rhizobium sp. strain NGR234 and R. fredii USDA257 share exceptionally broad, nested host ranges. Molecular Plant-Microbe Interactions 12, 293-318.
| Crossref | Google Scholar |
Reichard SH, White P (2001) Horticulture as a pathway of invasive plant introductions in the United States: most invasive plants have been introduced for horticultural use by nurseries, botanical gardens, and individuals. BioScience 51, 103-113.
| Crossref | Google Scholar |
Riley SJ, Banks RG (1996) The role of phosphorus and heavy metals in the spread of weeds in urban bushlands: an example from the Lane Cove Valley, NSW, Australia. Science of The Total Environment 182, 39-52.
| Crossref | Google Scholar |
Robinson D, Hayes A (2019) broom: convert statistical analysis objects into tidy tibbles. R package version 0.5.2. Available at https://cran.r-project.org/web/packages/broom/index.html
Seabloom EW, Harpole WS, Reichman OJ, Tilman D (2003) Invasion, competitive dominance, and resource use by exotic and native California grassland species. Proceedings of the National Academy 100, 13384-13389.
| Crossref | Google Scholar |
Shane MW, McCully ME, Lambers H (2004) Tissue and cellular phosphorus storage during development of phosphorus toxicity in Hakea prostrata (Proteaceae). Journal of Experimental Botany 55, 1033-1044.
| Crossref | Google Scholar |
Soberón J, Nakamura M (2009) Niches and distributional areas: concepts, methods, and assumptions. Proceedings of the National Academy 106, 19644-19650.
| Crossref | Google Scholar |
Sprent JI, Thomas RJ (1984) Nitrogen nutrition of seedling grain legumes: some taxonomic, morphological and physiological constraints. Plant, Cell & Environment 7, 637-645.
| Crossref | Google Scholar |
Standish RJ, Stokes BA, Tibbett M, Hobbs RJ (2007) Seedling response to phosphate addition and inoculation with arbuscular mycorrhizas and the implications for old-field restoration in Western Australia. Environmental and Experimental Botany 61, 58-65.
| Crossref | Google Scholar |
Stock WD, Verboom GA (2012) Phylogenetic ecology of foliar N and P concentrations and N:P ratios across mediterranean-type ecosystems. Global Ecology and Biogeography 21, 1147-1156.
| Crossref | Google Scholar |
Suding KN, LeJeune KD, Seastedt TR (2004) Competitive impacts and responses of an invasive weed: dependencies on nitrogen and phosphorus availability. Oecologia 141, 526-535.
| Crossref | Google Scholar |
Suriyagoda LDB, Ryan MH, Renton M, Lambers H (2010) Multiple adaptive responses of Australian native perennial legumes with pasture potential to grow in phosphorus- and moisture-limited environments. Annals of Botany 105, 755-767.
| Crossref | Google Scholar |
Säumel I, Kowarik I (2010) Urban rivers as dispersal corridors for primarily wind-dispersed invasive tree species. Landscape and Urban Planning 94, 244-249.
| Crossref | Google Scholar |
Thuiller W, Richardson DM, Pyšek P, Midgley GF, Hughes GO, Rouget M (2005) Niche-based modelling as a tool for predicting the risk of alien plant invasions at a global scale. Global Change Biology 11, 2234-2250.
| Crossref | Google Scholar |
Urbanek S, Horner J (2019) Cairo: R graphics device using Cairo Graphics Library for creating high-quality bitmap (PNG, JPEG, TIFF), vector (PDF, SVG, PostScript) and display (X11 and Win32) output. R package version 1.5-10. Available at https://cran.r-project.org/web/packages/Cairo/index.html
Valliere JM, Irvine IC, Santiago L, Allen EB (2017) High N, dry: experimental nitrogen deposition exacerbates native shrub loss and nonnative plant invasion during extreme drought. Global Change Biology 23, 4333-4345.
| Crossref | Google Scholar |
Vance CP, Uhde-Stone C, Allan DL (2003) Phosphorus acquisition and use: critical adaptations by plants for securing a nonrenewable resource. New Phytologist 157, 423-447.
| Crossref | Google Scholar |
Vergutz L, Manzoni S, Porporato A, Novais RF, Jackson RB (2012) Global resorption efficiencies and concentrations of carbon and nutrients in leaves of terrestrial plants. Ecological Monographs 82, 205-220.
| Crossref | Google Scholar |
Vilà M, Espinar JL, Hejda M, et al. (2011) Ecological impacts of invasive alien plants: a meta-analysis of their effects on species, communities and ecosystems. Ecology Letters 14, 702-708.
| Crossref | Google Scholar |
Viscarra Rossel RA, Bui EN (2016) A new detailed map of total phosphorus stocks in Australian soil. Science of The Total Environment 542, 1040-1049.
| Crossref | Google Scholar |
von der Lippe M, Kowarik I (2007) Long-distance dispersal of plants by vehicles as a driver of plant invasions. Conservation Biology 21, 986-996.
| Crossref | Google Scholar |
Wang X, Veneklaas EJ, Pearse SJ, Lambers H (2015) Interactions among cluster-root investment, leaf phosphorus concentration, and relative growth rate in two Lupinus species. American Journal of Botany 102, 1529-1537.
| Crossref | Google Scholar |
Webber BL, Scott JK (2012) Rapid global change: implications for defining natives and aliens. Global Ecology and Biogeography 21, 305-311.
| Crossref | Google Scholar |
Webber BL, Yates CJ, Le Maitre DC, Scott JK, Kriticos DJ, Ota N, McNeill A, Le Roux JJ, Midgley GF (2011) Modelling horses for novel climate courses: insights from projecting potential distributions of native and alien Australian acacias with correlative and mechanistic models. Diversity and Distributions 17, 978-1000.
| Crossref | Google Scholar |
Weiss SB (1999) Cars, cows, and checkerspot butterflies: nitrogen deposition and management of nutrient-poor grasslands for a threatened species. Conservation Biology 13, 1476-1486.
| Crossref | Google Scholar |
Wickham H (2017) tidyverse: easily install and load ‘Tidyverse’ packages. R Package version 1.1.1. Available at https://cran.r-project.org/web/packages/tidyverse/index.html
Wickham H (2019) modelr: modelling functions that work with the pipe. R package version 0.1.5. Available at https://cran.r-project.org/web/packages/modelr/index.html
Williams A, George S, Birt HWG, Daws MI, Tibbett M (2019) Sensitivity of seedling growth to phosphorus supply in six tree species of the Australian Great Western Woodlands. Australian Journal of Botany 67, 390-396.
| Crossref | Google Scholar |
Wilson TB, Witkowski ETF (1998) Water requirements for germination and early seedling establishment in four African savanna woody plant species. Journal of Arid Environments 38, 541-550.
| Crossref | Google Scholar |
Witkowski ETF (1991) Growth and competition between seedlings of Protea repens (L.) L. and the alien invasive, Acacia saligna (Labill.) Wendl. in relation to nutrient availability. Functional Ecology 5, 101-110.
| Crossref | Google Scholar |
Witkowski ETF (1994) Growth of seedlings of the invasives, Acacia saligna and Acacia cyclops in relation to soil phosphorus. Australian Journal of Ecology 19, 290-296.
| Crossref | Google Scholar |
Zhou XM, Ranathunge K, Cambridge ML, Dixon KW, Hayes PE, Nikolic M, Shen Q, Zhong H, Lambers H (2022) A cool spot in a biodiversity hotspot: why do tall Eucalyptus forests in Southwest Australia exhibit low diversity? Plant and Soil 476, 669-688.
| Crossref | Google Scholar |