Mobilisation and replenishment of phosphorus reserves in Bos indicus cows. 1. Mid-pregnant mature cows post-weaning
R. M. Dixon
A
B
C
D
E
F
G Present address:
Abstract
Lactating beef cows grazing phosphorus (P)-deficient pastures often mobilise body P to alleviate a P deficiency. Knowledge of the circumstances when body P is mobilised, and later replenished, is necessary for optimal management of the P nutrition of breeder herds.
To investigate mobilisation and replenishment of body P in mature Bos indicus-cross beef cows post-weaning.
Cows (n = 40) in their second trimester of pregnancy were individually fed ad libitum low-P (LP) or high-P (HP) diets containing moderate or high metabolisable energy (ModE-LP, HighE-LP, ModE-HP and HighE-HP) for 14 weeks.
Plasma inorganic P concentrations (Pi) in LP- or HP-diet cows (0.58 and 2.15 mmol/L respectively) indicated that diet P was deficient or adequate. Intakes of DM and metabolisable energy, liveweight gain, and P retention were higher (P < 0.05 to P < 0.001) in cows fed the HP diets, and were also increased in the high metabolisable-energy diets. Efficiency in use of metabolisable energy for slow growth was lower in the HighE-LP than the ModE-HP cows. The cows fed the LP diets mobilised 4–5 g body P/day. Cows fed the Mod-HP and HighE-HP diets retained 1.1 and 8.8 g body P/day, and those ModE-HP cows with low bone P reserves retained ~2.3 g P/day. Rib cortical bone P did not change in HP cows but decreased (P < 0.05) in LP cows. The HP diets increased the bone volume, mineralised bone, and the thickness of the struts in trabecular bone in the tuber coxae. Osteoid tissue decreased (P < 0.05) in HighE-HP cows. Changes in plasma concentrations of 1,25-dihydroxy vitamin D3, carboxy-terminal telopeptides of type I collagen (CTX-1) and bone alkaline phosphatase (BAP) were in accord with substantial bone mobilisation in cows fed LP diets, and bone replenishment with HP diets.
Cows that had ingested P-deficient diets during lactation were able to further mobilise body P when fed LP diets post-weaning, but cows replenished body P when fed HP diets.
Mature cows with low body P reserves can replenish these reserves more rapidly when consuming diets high in both P and metabolisable energy.
Keywords: bone mineral replenishment, bone phosphorus, diet quality, energetic efficiency, liveweight change, P deficiency, plasma markers, plasma minerals.
Introduction
Many rangelands, particularly in northern Australia, South America, and Africa, have low soil phosphorus (P) concentrations that lead to low P in the pastures. Grazing beef cattle that are entirely dependent on such pastures are often P deficient, particularly during the rainy season when metabolisable energy and protein in pastures are highest and therefore when the demands of the animal for P are also highest (Winter 1988; Kerridge et al. 1990; Winks 1990). In herds grazing rangelands, signs of P deficiency are most often observed in lactating breeder cows because of their high demands for P during late pregnancy and lactation (Hart and Mitchell 1965; McCosker and Winks 1994). The most common symptom of P deficiency is reduced animal productivity, although acute and prolonged P deficiency in breeder cows causes osteophagea, poor body condition, low fertility, low bone mineralisation, bone breakages, high mortalities and osteomalacia (Dixon et al. 2020a; Jackson et al. 2023).
During dietary P deficiency in late pregnancy and lactation most ruminants, including beef-genotype cows, can mobilise a substantial proportion (e.g. often >30% and in at least some circumstances up to 45%) of their bone minerals (Benzie et al. 1959; Little 1983; Dixon et al. 2017, 2020a). Cows that mobilise a high proportion of bone minerals during late pregnancy and lactation need to replenish these reserves in preparation for the next reproductive cycle. When beef cows grazing P-deficient pastures are not able to replenish their body P, there are often severe adverse effects on liveweight, lactation, reconception, welfare, and mortality (Read et al. 1986a, 1986b, 1986c; Spangenberg 1997; Dixon et al. 2017; Schatz et al. 2023). Similar adverse outcomes also occur in severely P-deficient dairy cows (Valk and Sebek 1999; Knowlton and Herbein 2002). In seasonally dry rangeland environments where cows typically lactate during the rainy season, it will often be desirable to replenish cow body P reserves by feeding P supplements during the late rainy season and the early dry season when cows are in late lactation or after weaning. However, the effectiveness of P supplementation to replenish cow body P during these seasons is not known.
An experiment investigated the hypothesis that mature pregnant Bos indicus-cross breeder cows that had grazed P-deficient pastures during lactation, and were therefore expected to have low bone P reserves at weaning, could (i) continue to mobilise body P to maintain voluntary feed intake and plasma inorganic P (Pi) when fed P-deficient diets, and (ii) replenish body P reserves when consuming diets with either moderate or high metabolisable-energy (ME) content together with additional P surplus to immediate requirements. The experiment examined a high-ME diet (~9.5 MJ ME/kg DM) comparable with high-quality grass or grass–legume pastures during the mid- to late rainy season, versus a moderate-ME diet (~8 MJ ME/kg DM) comparable with grass pastures during the late rainy season and the transition into the dry season.
Materials and methods
Selection and housing of the cows
Mature Bos indicus × Bos taurus-cross cows (~5/8–3/4 Bos indicus, Fn, Droughtmaster type, 6–11 years of age) were selected from a mixed-age herd on the Queensland Department of Primary Industries Spyglass Cattle Research Station (100 km west of Townsville, Queensland, Australia) in the seasonally dry tropics. The herd had grazed tropical northern speargrass native pastures in an open Eucalyptus woodland paddock without any supplementation during the 6 months before selection of cows on the 19 June 2014. The paddock primarily comprised soils containing <4 and 4–6 mg Colwell PB/kg DM (Bryant and Harms 2016) that are classed as ‘acutely deficient’, and ‘deficient’ in P respectively, for grazing cattle (Kerridge et al. 1990; McCosker and Winks 1994; Jackson et al. 2023). The cows had been mated annually from ~2 years of age, and most recently from 15 January to 5 May 2014. The cows were blood-sampled from the coccygeal vein on the 5 May 2014 for measurement of Pi. On the 18 June 2014, the herd was mustered, calves weaned and pregnancy evaluated by rectal palpation. Forty cows were selected as (i) previously lactating through the 2013–2014 rainy season, (ii) currently 2.0–3.5 months pregnant, (iii) in body condition score (BCS) 2.0–3.3 on a 5-point scale (CSIRO 2007), and (iv) temperament suitable for an intensive pen experiment. About one-third of the cows were in each of the Age groups of 5–6, 7–8 and 9–11 years. The experiment was approved by the Queensland Department of Primary Industries Animal Ethics Committee (SA2014/04/464 and SA2014/04/470).
The cows were transported by truck to the Brian Pastures Research Station, Gayndah, in the subtropics of Queensland. After arrival (20 June 2014), the cows were allowed to recover from transport, while held in yards and fed barley straw ad libitum, and were blood-sampled on the 23 June 2014. The cows were then fed a low-P diet (the ModE-LP diet described below). On the 26 June 2014, liveweight of the cows was measured, BCS evaluated, the cows (n = 40) were randomly allocated to 10 blocks, and the four cows within each block were randomly allocated to the four treatments. The cows were allocated to 40 individual pens in the animal shed, with the four cows in each block in adjoining pens. The cows were (mean ± s.d. (range)) 430 ± 45 (307–502) kg liveweight, 2.7 ± 0.35 (2.0–3.3) BCS, and fetal age was 12 ± 1.2 (9–14) weeks. Thus, the cows were generally in their second trimester of pregnancy during the experiment. Water was available in each pen. Half of each pen (~33 m2) with the feed bunk was roofed and with a concrete floor, whereas the remainder was soil. Additional shade was provided with canvas sails.
Diets, total collections of faeces and measurements of liveweight and BCS
The four diet treatments in a 2 × 2 factorial design were as follows: (i) moderate ME, low P (ModE-LP), (ii) moderate ME, high P (ModE-HP), (iii) high ME, low P (HighE-LP), and (iv) high ME, high P (HighE-HP). The main effects of P concentration are referred to as LP or HP diets, whereas the main effects of ME content are referred to as ModE or HighE diets. The diets were prepared as total mixed rations based on coarsely chopped wheat straw, wheat flour and sugar. The HighE diets contained (g/kg as fed) 512 wheat straw, 282 wheat flour and 141 sugar, whereas the ModE diets contained 667 wheat straw, 186 wheat flour and 94 sugar (Table 1). The HP diets contained mono dicalcium phosphate (Kynophos, KK Animal Nutrition Pty Ltd, Umbogintwini, South Africa). The treatment diets were fed daily from the 26 June 2014 (Day 1) for 101 days, and cows were individually offered ~10% in excess of their previous intakes to achieve ad libitum intakes. Feed refusals were collected weekly. Cows were introduced to the treatment diets over 4 days, during which additional straw was added to the diet to minimise the risk of acidosis. Water was freely available from troughs in each pen.
Feed and composition | Treatment diet | ||||
---|---|---|---|---|---|
ModE-LP | ModE-HP | HighE-LP | HighE-HP | ||
Ingredients (g/kg as fed) | |||||
Wheat straw | 669 | 664 | 515 | 509 | |
Wheat flour | 186 | 185 | 283 | 280 | |
Sugar | 94 | 93 | 142 | 140 | |
Canola oil | 29 | 29 | 22.4 | 22.1 | |
Urea | 11.9 | 11.8 | 22.4 | 22.1 | |
Calcium phosphate A | 0 | 6.2 | 0 | 9.4 | |
Limestone | 3.1 | 3.1 | 4.8 | 4.7 | |
Ammonium sulfate | 3.1 | 3.1 | 4.8 | 4.7 | |
Sodium chloride | 3.1 | 3.1 | 4.8 | 4.7 | |
Rumigro premix B | 0.50 | 0.50 | 0.77 | 0.76 | |
Elanco rumensin 100 C | 0.25 | 0.25 | 0.27 | 0.27 | |
Composition | |||||
Dry matter (g/kg as fed) | 948 | 946 | 947 | 944 | |
Organic matter (g/kg DM) | 912 (5) | 911 (3) | 928 (4) | 927 (6) | |
Crude protein | 67.2 (5.0) | 72.4 (2.3) | 111 (7.1) | 115 (5.8) | |
Neutral detergent fibre | 593 (12) | 577 (29) | 432 (28) | 427 (26) | |
Acid detergent fibre | 374 (30) | 372 (8) | 284 (19) | 279 (15) | |
Lignin | 51 (10) | 44 (11) | 34 (5) | 37 (6) | |
Starch | 86 (9) | 95 (6) | 189 (13) | 193 (8) | |
Crude fat | 29 (1.9) | 28 (2.2) | 33 (2.9) | 31 (2.7) | |
Calcium | 3.02 (0.335) | 4.30 (0.868) | 2.96 (0.332) | 4.74 (0.530) | |
Phosphorus | 0.68 (0.071) | 1.58 (0.356) | 0.76 (0.057) | 2.11 (0.282) | |
Magnesium | 0.91 (0.05) | 0.95 (0.06) | 0.72 (0.06) | 0.83 (0.01) | |
Sulfur | 2.0 (0.36) | 1.8 (0.32) | 2.3 (0.33) | 2.0 (0.16) | |
Calcium:phosphorus | 4.5 (0.50) | 2.9 (0.20) | 3.9 (0.23) | 2.2 (0.13) |
Cow liveweight was measured, and cow BCS evaluated by the same experienced person, weekly. Voluntary intake of feed was measured weekly from the amounts offered and refused. The feed offered was sampled when each batch of diet was mixed, and feed refusals were sampled weekly from each pen. During 5-day total collections the faeces were collected frequently from the concrete floors during Weeks 3, 8 and 14, while the animals were constrained to the section of the pen with concrete flooring. The feed offered and refused during the total collection intervals were bulked and sampled for each cow. A 10% subsample of the faeces excreted each day was stored at −20°C, and at the end of the collection interval, these samples were mixed and subsampled. Feed offered and refused during other intervals of the experiment were bulked monthly. Feed and faeces samples were dried at 60°C, ground through a 1 mm screen (Christie and Norris Mill, Chelmsford, UK) and retained for analysis. Hip height of the cows was measured at the start and at the end of the experiment. The depth of fat at the P8 and rib sites, and the eye-muscle area were measured at Weeks 1 and 14 by ultrasound (Esaote Pie Medical Aquila with a 3.5 MHz linear array transducer; Pie Medical Imaging, Maastricht, The Netherlands).
Sampling of blood, urine and bone
Blood samples were obtained on the 23 June 2014 (Day –3), on the 30 June 2014 (Day 5) and then at fortnightly intervals, by jugular venipuncture using vacutainers containing lithium–heparin (BD, Diagnostics, Plymouth, UK). Samples were chilled in iced water, plasma was separated by centrifugation (3000g, 15 min at ambient temperature), and stored at −20°C. Urine samples (~30–60 mL) collected by stimulation of the vulva on days immediately preceding and following the total collection at Week 14 were acidified (pH < 3) with 4 M sulfuric acid, and stored at −20°C for subsequent analysis of creatinine, purine derivatives and P. Biopsy samples of outer cortical bone from the 12th rib, and from the tuber coxae bone, were obtained at commencement (Days 2–4) and from the opposite side of the cow near the end of the experiment (Days 93–95) (Little 1972; Kidd et al. 2014; Silva et al. 2021). The procedures used to obtain the bone biopsies are described in Supplementary Appendix S1.
Laboratory procedures
Organic matter was determined as the DM minus the ash measured by incineration (550°C for 8 h). Concentrations of P, calcium (Ca), magnesium (Mg) and sulfur (S) in the feed offered, feed refusals and faeces from the total collection intervals, were analysed using an inductively coupled plasma spectrometer (Optima 7300 DV, Perkin Elmer, Waltham, MA, USA) after nitric-perchloric acid digestion. Samples of feed offered were analysed for total nitrogen, neutral detergent fibre, acid detergent fibre, lignin and crude fat by near-infrared spectroscopy (NIRS) and using in-house calibrations (Dairy One, Ithaca, New York, USA). Purine derivatives (allantoin and uric acid) and creatinine in urine were measured by high-performance liquid chromatography (George et al. 2006).
Samples of rib cortical bone were scraped to remove any trabecular bone. Cortical bone thickness was measured using vernier callipers and specific gravity was measured gravimetrically. The concentration of P (mg P/mL) in rib cortical bone was calculated as follows (Dixon et al. 2019a):
The P in cortical rib bone per unit surface area of cortical bone (PSACB, μg P/mm2) index was calculated as the product of P concentration and the thickness of the cortical bone (Dixon et al. 2019a). Samples of cortical rib bone and tuber coxae trabecular bone for histology were fixed in 10% neutral buffered formalin for at least 8 weeks, decalcified in 14% EDTA (pH 7.0) for at least 12 weeks (Callis and Sterchi 1998), and then embedded in paraffin. Sections (5 μm) were stained with Masson’s trichrome, photographed on a microscope at ×2, ×10 and ×20 magnification. Measured attributes and changes were described following the nomenclature of Dempster et al. (2013). An image-analysis program (ImageJ; Doube et al. 2010; Schneider et al. 2012) was used to measure porosity (void volume:tissue volume; Po) in the rib cortical bone. The latter was expressed as (100-Po) so that increasing values corresponded with increasing amounts of bone in parallel with the measurements of P concentration in cortical rib bone. In tuba coxae bone, the measurements were the proportions of bone volume to total volume, osteoid to total bone volume, mineralised bone to total bone volume, mean trabecular strut thickness and maximum trabecular strut thickness. Mineralised bone and oestoid together represented the total bone volume. Changes were calculated from the measurements at the beginning and the end of the experiment.
Plasma samples were analysed calorimetrically for inorganic P (Pi), total Ca, and Mg by using a Beckman Coulter AU680 analyser (OSR6122, OSR 6189 and OSR6189 assay kits respectively), whereas the concentration of P in urine was analysed as described by Goodwin (1970). Plasma concentrations of a number of hormones and bone metabolism markers were determined using commercial assay kits as follows: parathyroid hormone (PTH; A11930, Beckman Coutler), 25-dihydroxy vitamin D3 and 1,25-dihydroxy vitamin D3 (AA-35F1 and AA-54F2, Immunodiagnostic Systems), insulin-like growth factor 1 (IGF-1; A15729, Beckman Coulter), carboxy-terminal telopeptides of type I collagen (CTX-1; Crosslaps AC-02F1, Immunodiagnostic Systems), bone alkaline phosphatase (BAP; MicroVue 8012, Quidel), and osteocalcin (OCN; MicroVue 8002, Quidel). Total alkaline phosphatase (ALP) and aspartate aminotransferase (AST) were analysed with kits (Beckman Coulter) on the basis of recommendations of the International Federation for Clinical Chemistry. Further details of these assays conducted in the same laboratory are given by Silva et al. (2021).
Calculations and statistical analyses
The age of the fetus at each measurement during the experiment was calculated from the actual date of parturition and assuming a 284 day gestation. The conceptus (gravid uterus) weight was calculated as described by O’Rourke et al. (1991) and the conceptus-free cow liveweight (CF-LW) was calculated by difference between the measured LW of the cow minus the estimated weight of the gravid uterus. The LW change of the cows was calculated as the difference between the initial and final LW.
The measured intakes of total P in each diet treatment were compared with the calculated P requirements of the cows (CSIRO 2007) (i) for the measured DM intakes, LWs and LW gains of the cows in the treatment, and also (ii) for the LP treatments, the P intakes were compared with the P requirement for the higher DM intakes and higher LW gains of the cows in the corresponding HP diet. The latter calculation allowed for the reduction in voluntary intakes during P deficiency, lower LW, and lower LW gain of cows fed the LP treatment diets. The amounts of absorbed P ingested in each of the diets were calculated as described by CSIRO (2007), (i) adopting their recommended true absorption coefficient of 0.70, and also (ii) when adopting a true absorption coefficient of 0.90 for the P as mono dicalcium phosphate included in the HP diets (Dixon et al. 2020a). Apparent digestibilities of DM and organic matter were calculated by conventional procedures. The ME contents of the diets (MJ ME/kg DM) were calculated from the organic-matter digestibility (OMD) measured during the total collections as follows (CSIRO 2007, p. 8):
The volume of urine excreted at the Week 14 total collection was calculated from the creatinine concentration in urine and assuming a daily creatinine excretion of 0.91 mmol/kg W0.75.day (Chen et al. 1995). Microbial crude protein synthesis was calculated from the excretion of purine derivatives (Chen and Gomes 1992), assuming an endogenous purine derivative excretion of 0.190 mmol/kg W075.day (Bowen et al. 2006). The P retention was calculated as the difference between intake and faecal excretion of P during each of the total collection intervals.
Statistical analysis of the results was conducted by ANOVA using Genstat (release 16.1, VSN International, Hemel Hemstead, UK). Measurements of the animals at the commencement of the experiment (cow LW, BCS and age, fetal age, rib cortical bone thickness, P concentration and PSACB) were examined as potential covariates and were included in the statistical model when significant (P < 0.05). Repeated-measures ANOVA in a 2 × 2 factorial design with main effects of diet energy and diet P, time, and the interactions, was used for analyses of intakes, digestibilities, P retentions, blood minerals, hormones and bone metabolism markers. Measurements of the metabolites PTH, 25-dihydroxy vitamin D3, 1,25-dihydroxy vitamin D3, ALP, AST and IGF-1 were transformed (log(X + 1)10) to address heterogeneity of variance. The effects of the diet treatments at individual time points were compared post hoc using Fishers protected least significant difference (l.s.d.) tests. In addition, a one-tailed t-test was used to examine whether changes in P retention, and in attributes of rib and tuba coxae bone, were greater than, or less than, zero in the HP and LP diets respectively.
Results
Initial status of the cows and the intakes of P provided by the treatment diets
The Pi measured on 5 May 2014 in the cows selected for the experiment when they were grazing late rainy–early dry transition-season native pastures was (mean ± s.d. (range)) 1.22 ± 0.48 (0.4–2.6) mmol/L. After arrival at Brian Pastures Research Station and having been fed barley straw for 3 days, the Pi was 1.78 ± 0.41 (1.02–2.73) mmol/L, plasma Ca 2.23 ± 0.41 mmol/L, and the ratio plasma Ca:Pi 0.81 ± 0.41. The diet treatments were commenced 3 days later. The initial cortical bone thickness was 3.73 ± 0.70 (2.70–4.99) mm, the P concentration was 149 ± 9.4 (130–162) mg P/mL, and the PSACB was 556 ± 116 (352–783) μg P/mm2. In tuber coxae trabecular bone, the initial bone volume/total volume averaged 8.68 ± 2.60 (4.72–15.91)%, mineralised volume/total volume 7.08 ± 2.28 (4.16–14.60)%, osteoid volume/total volume 1.80 ± 1.27 (0.20–5.03)%, and trabecular strut thickness 107 ± 12.8 (91–156) μm.
The total P concentrations in the ModE-LP and HighE-LP diets were 0.68 and 0.76 g P/kg DM, and in the ModE-HP and HighE-HP diets 1.58 and 2.11 g P/kg DM respectively (Table 1). The crude protein (CP) to ME ratio, calculated from values in Tables 1 and 2, ranged from 7.9 to 12.2 g CP/MJ ME. Calculations of the P requirements based on an adopted true absorption coefficient of 0.70 indicated that the ModE-HP and HighE-HP diets provided 0.99 and 1.10 of P requirements respectively (Table 2). When a true absorption coefficient of 0.90 was adopted for mono dicalcium phosphate, the ModE-HP and HighE-HP diets provided 1.15 and 1.30 of the absorbed P requirements respectively. The ModE-LP and HighE-LP diets both provided 0.50 of both the total and absorbed P requirements for the measured LW gains, but provided only 0.36 and 0.27 of the P intakes required to achieve the higher intakes and LW gains measured in the respective HP diets, as limited by animal factors and diet other than P. Therefore, the two LP diets were acutely P deficient and the two HP diets provided in excess of the estimated requirements. Intakes of Ca ranged among the diets from 1.3 to 1.8 of the requirements.
Measurement | Diet treatment | s.e.m. (df 19) | Cov | Probability | |||||||
---|---|---|---|---|---|---|---|---|---|---|---|
ModE-LP | ModE-HP | HighE-LP | HighE-HP | Energy (ME) | Phos (P) | ME × P | Time (T) | ||||
P intake and requirements | |||||||||||
Total P intake (g P/day) | 3.7 | 10.3 | 5.2 | 21.4 | – | – | – | – | – | – | |
Total P requirement (g P/day) | 7.5 | 10.4 | 10.4 | 19.5 | – | – | – | – | – | – | |
Total P intake/requirement-A | 0.50 | 0.99 | 0.50 | 1.10 | – | – | – | – | – | – | |
Total P intake/requirement-B | 0.36 | – | 0.27 | – | – | – | – | – | – | – | |
Absorbed P intake (g P/day) | 2.6 | 8.4 | 3.6 | 17.7 | – | – | – | – | – | – | |
Absorbed P requirement (g P/day) | 5.3 | 7.3 | 7.3 | 13.6 | – | – | – | – | – | – | |
Absorbed P intake/requirement-A | 0.50 | 1.15 | 0.50 | 1.30 | – | – | – | – | – | – | |
Absorbed P intake/requirement-B | 0.36 | – | 0.27 | – | – | – | – | – | – | – | |
Voluntary intakes | |||||||||||
DM intake (kg/day) | 5.47c | 6.54b | 6.83b | 10.14a | 0.15 | 1 | <0.001 | <0.001 | <0.001 | <0.001 | |
DM intake (g/kg LW.day) | 13.4c | 14.9b | 15.8b | 21.5a | 0.30 | 1 | <0.001 | <0.001 | <0.001 | <0.001 | |
OMD Week 3 (g/kg) | 618 | 516 | 676 | 622 | 13 | – | <0.001 | <0.001 | n.s. | – | |
OMD Week 8 (g/kg) | 629 | 622 | 695 | 725 | 9 | – | <0.001 | n.s. | n.s. | – | |
OMD Week 14 (g/kg) | 610c | 579d | 645b | 690a | 7 | – | <0.001 | n.s. | <0.001 | – | |
OMD Mean (g/kg) | 619b | 572c | 672a | 680a | 7 | – | <0.001 | 0.06 | <0.01 | <0.001 | |
ME content (MJ/kg) | 8.47b | 7.68c | 9.37a | 9.46a | 0.12 | – | <0.001 | n.s. | <0.05 | – | |
ME intake (MJ/day) | 45.8c | 50.7c | 64.2b | 96.0a | 1.42 | 1 | <0.001 | <0.001 | <0.001 | – | |
ME intake (kJME/kg LW.day) | 112c | 115c | 148b | 204a | 2.7 | 2 | <0.001 | <0.001 | <0.001 | – | |
Microbial CP (g/day) | 354b | 358b | 387b | 573a | 17.4 | – | <0.001 | <0.001 | <0.001 | – | |
Microbial CP/ME (g/MJ ME.day) | 7.77 | 8.30 | 6.78 | 6.34 | 0.40 | 1 | <0.05 | n.s. | n.s. | – | |
Liveweight and BCS | – | ||||||||||
Initial LW (kg) | 434 | 409 | 414 | 421 | 8.9 | – | n.s | n.s. | n.s. | – | |
LW change (kg) | +6c | +29b | +26b | +97a | 3.4 | – | <0.001 | <0.001 | <0.001 | – | |
LW change (kg/day) | 0.07c | 0.32b | 0.29b | 1.05a | 0.037 | – | <0.001 | <0.001 | <0.001 | – | |
Initial BCS | 2.6 | 2.7 | 2.7 | 2.7 | 0.07 | – | n.s. | n.s. | n.s. | – | |
BCS change | −0.10c | 0.25b | 0.27b | 1.25a | 0.08 | – | <0.001 | <0.001 | <0.01 | – | |
Ultrasound measurements | – | ||||||||||
Initial rump fat (mm) | 1.5 | 2.3 | 2.0 | 1.7 | 0.25 | – | n.s. | n.s. | n.s. | – | |
Change in rump fat (mm) | 0.5c | 0.5c | 2.3b | 8.7a | 0.32 | 2 | <0.001 | <0.001 | <0.001 | – | |
Initial rib fat (mm) | 1.4 | 1.5 | 1.6 | 1.3 | 0.15 | – | n.s. | n.s. | n.s. | – | |
Change in rib fat (mm) | −0.2c | 0.2bc | 0.9b | 4.4a | 0.23 | – | <0.001 | <0.001 | <0.001 | – | |
Initial EMA (mm2) | 50.5 | 53.5 | 53.5 | 56.4 | 1.83 | – | n.s. | n.s. | n.s. | – | |
Change in EMA (mm2) | −3.2b | 0.9b | 0.7b | 14.3a | 1.01 | – | <0.001 | <0.001 | <0.01 | – |
Means in the same row with different lower-case letters are significantly different (at P ≤ 0.05), and the means in the same column, within the same measurement, with different upper-case letters are significantly different (at P ≤ 0.05).
LW, liveweight; BCS, body condition score; cov, covariates: 1, cow initial liveweight; 2, cow age. ME × P, The interaction between metabolisable energy and phosphorus in the diet.
Intake, diet digestibility, LW change and rumen microbial synthesis
The cows were all in good health and no signs of osteomalacia such as abnormal gait were observed through the experiment. The voluntary intakes of DM and organic matter by the cows were increased (P < 0.001) by both higher ME and higher P contents of the diets, and there was an ME × P interaction (P < 0.001) (Table 2). Voluntary intake of the moderate-ME diet was increased 11% by additional P in the diet (13.4 and 14.9 g DM/kg LW in the ModE-LP and ModE-HP diets respectively; P < 0.05), whereas voluntary intake of the high-ME diet was increased by 36% by additional P (15.8 and 21.5 g DM/kg LW.day in the HighE-LP and HighE-HP diets respectively; P < 0.001). The voluntary DM intakes of the ModE-HP and the HighE-LP diets were similar (P > 0.05). Voluntary DM intake decreased (P < 0.001) by, on average, 0.33 g DM/kg LW.week in each of the diets through the experiment.
Organic matter digestibility was consistently higher in the high-ME diets than in the moderate-ME diets, averaging 676 and 596 g/kg respectively (P < 0.001) (Table 2). There was an ME × P interaction effect on the OMD (P < 0.01); OMD of the ModE-HP diet was lower (P < 0.05) than of the ModE-LP diet (572 and 619 g/kg respectively, and the OMDs of both of the moderate-ME diets were lower than those of the high-ME diets (mean 676 g/kg). Also the OMD was lower at the total collection at Week 3 than at Weeks 8 and 14 (P < 0.001). Voluntary intakes of ME through the 14 weeks averaged 112 and 115 kJ ME/kg LW.day for the ModE-LP and ModE-HP treatments, and increased (P < 0.001) to 148 and to 204 kJ ME/kg LW.day for the HighE-LP and HighE-HP diets respectively (Table 2). Microbial CP (MCP) synthesis per day was similar in the ModE-LP, ModE-HP and HighE-LP diets (354–387 g MCP/day), and was higher in the HighE-HP diet (573 g MCP/day; P < 0.05), in association with the higher ME intake. However, the efficiency of microbial synthesis was higher for the moderate-ME diets than for the high-ME diets (means 8.04 and 6.56 g MCP/MJ ME; P < 0.05).
Phosphorus intakes, excretion and retention
The average intakes of P during the three total collection intervals were 2.9 and 3.7 g P/day (7.1 and 9.0 mg P/kg LW.day) for the LP diets, and 10.0 and 20.2 g P/day (22.8 and 43.5 mg P/kg LW.day) for the HP diets (Table 3). Mean faecal P excretion ranged from 6.8 to 12.0 g P/day. Urinary P excretion at the total collection in Week 14 was negligible (≤0.1 g P/day; data not shown) and, therefore, the differences between intake and faecal excretion were used as the measure of P retention. The P retention was not significantly affected by the total collection interval and averaged −4.1 and −4.7 g P/day (−9.3 and −11.2 mg P/kg LW.day) in the ModE-LP and HighE-LP diets respectively, indicating that these amounts of body P were being mobilised. In contrast, cows fed the ModE-HP diet tended to be in a small positive P retention (+1.1 g P/day; +2.5 mg P/kg LW.day), although this was not different (P > 0.05) from zero. The cows fed the HighE-HP diet retained 8.8 g P/day (18.4 mg P/kg LW.day).
Measurement | Diet treatment | cov | s.e.m. (d.f. 19) | Probability | |||||||
---|---|---|---|---|---|---|---|---|---|---|---|
ModE-LP | ModE-HP | HighE-LP | HighE-HP | Energy (ME) | Phos (P) | ME × P | Time (T) | ||||
P intake | |||||||||||
Week 3 (g/day) | 3.1a | 9.4bA | 4.0c | 17.3a | 1, 2 | 0.36 | <0.001 | <0.001 | <0.001 | – | |
Week 8 (g/day) | 3.4a | 9.8bAB | 3.0a | 21.2cB | 1, 2 | 0.51 | <0.001 | <0.001 | <0.001 | – | |
Weel 14 (g/day) | 2.3a | 11.0cB | 4.1b | 22.8d | 1 | 0.32 | <0.001 | <0.001 | <0.001 | – | |
Mean (g/day) | 2.9c | 10.0b | 3.7c | 20.2a | 1, 2 | 0.51 | <0.001 | <0.001 | <0.001 | <0.001 | |
Faecal P excretion | |||||||||||
Week 3 (g/day) | 5.7a | 7.3a | 6.8aA | 10.6bA | – | 0.60 | <0.05 | <0.01 | <0.05 | – | |
Week 8 (g/day) | 7.2a | 8.6ab | 10.3bcB | 11.7cAB | – | 1.25 | n.s. | n.s. | <0.05 | – | |
Week 14 (g/day) | 7.6a | 9.9a | 8.4aAB | 13.7bB | – | 1.30 | n.s. | 0.06 | <0.05 | – | |
Mean (g/day) | 6.8a | 8.6a | 8.5a | 12.0b | 3 | 1.05 | <0.05 | <0.05 | <0.05 | <0.05 | |
P retention | |||||||||||
Week 3 (g/day) | −2.8a | +1.7b | −2.8a | +7.2c | – | 0.60 | <0.01 | <0.001 | <0.01 | – | |
Week 8 (g/day) | −3.9ab | +0.8b | −7.2a | +9.9c | – | 1.32 | n.s. | <0.001 | <0.01 | – | |
Week 14 (g/day) | −5.5a | +0.7b | −4.2a | +9.5c | – | 1.69 | <0.01 | <0.001 | 0.05 | – | |
Mean (g/day) | −4.1a | +1.1b | −4.7a | +8.8c | 2 | 1.21 | <0.01 | <0.001 | <0.01 | n.s. | |
P intake, mean (mg/kg LW.day) | 7.1a | 22.8b | 9.0a | 43.5c | 2 | 1.07 | <0.001 | <0.001 | <0.001 | <0.001 | |
Faecal P, mean (mg/kg LW.day) | 16.1 | 20.6 | 20.3 | 25.1 | – | 1.87 | n.s. | 0.09 | n.s. | <0.001 | |
P retention, mean (mg/kg LW.day) | −9.3a | +2.5b | −11.2a | +18.4c | – | 2.67 | <0.01 | <0.001 | <0.01 | n.s. |
Means in the same row with different lower-case letters are significantly different (at P < 0.05), and the means in the same column, within the same measurement, with different upper-case letters are significantly different (at P < 0.05).
LW, liveweight; cov, covariates: 1, cow age; 2, cow initial liveweight; 3, cow initial BCS; ME × P, the interaction between metabolisable energy and phosphorus in the diet.
The rates of LW gain of cows in each of the treatments were consistent throughout the 14 weeks. The ModE-LP diet cows were at about LW maintenance (+0.07 kg LW/day) (Table 2). The inclusion of P (ModE-HP diet) significantly (P < 0.05) increased LW gain to 0.32 kg LW/day, similar to that of cows fed HighE-LP (0.29 kg LW/day). The HighE-HP diet resulted in a large increase (P < 0.05) in growth, to 1.05 kg LW/day. Thus, provision of adequate diet P increased the LW gain much more in cows fed the high-ME diet, as described above. The ME intake was 29% higher for the HighE-LP diet than for the ModE-HP (148 and 115 kJ ME/kg LW.day; P < 0.05), even though the LW gains were similar (Table 2). Therefore, ingested ME was used with higher efficiency in cows fed the P-adequate diet than in those fed the P-deficient diet. Because the conceptus was estimated to grow, on average, from 4 to 16 kg during the experiment, the gains in total LW of the cows were ~0.13 kg/day higher than were the gains in CF-LW.
There were few changes during the experiment, or differences between the ModE-HP and the two LP treatments in BCS, fat depth at the rump and rib sites, and eye-muscle area (EMA, Table 2), although each of these attributes increased (P < 0.05) in cows fed the HighE-HP diet. Hip height of the cows (initially 1380 ± 43 mm) did not change (P > 0.05).
Changes in rib and tuber coxae bone
The cortical thickness of rib bone was maintained in cows fed the HP diets, but decreased, on average, by 12% (−0.44 mm) in cows fed LP diets (P < 0.05) (Table 4). The P concentration in the cortical bone was not affected by diet P, but decreased in the cows fed the high-ME diets by 4% (10 mg P/cc; P < 0.01). The PSACB, which estimates the amount of P in cortical rib bone, did not change through the experiment in cows fed the high-ME diet (P > 0.05). However, the PSACB decreased by 14% (77 mg P/mm2) in LP-diet cows (P < 0.05), in association with changes in cortical bone thickness rather than in P concentration. Furthermore, across all the treatments, the change in PSACB was correlated with the initial PSACB (Fig. 1a), indicating that cows with initial higher PSACB mobilised more bone. Rib cortical bone volume expressed as (100-Po) indicated that the HP-diet cows gained bone, whereas the LP-diet cows lost bone (1.57% increase and 1.86% decrease respectively; P < 0.05) (Table 4), with these increases and decreases both different from zero (P < 0.05). Across all the treatments, the change in 100-Po was correlated with the initial (100-Po) (Fig. 1b), indicating that cows with initial higher (100-Po) mobilised more bone. The relationships for the PSACB (Y1 and X1) and for (100-Po) (Y2 and X2) were as follows: (a) Y1 = 132–0.321 (X1) (n = 36, r = 0.38, P < 0.05). (b) Y2 = 79.3 – 0.825 (X2) (n = 30, r = 0.49, P < 0.01). In addition, there was a negative correlation between the change in (100-Po) and cow age (r = −0.39, n = 32, P < 0.05), indicating that bone replenishment occurred more slowly in older cows.
Measurement | Diet treatment | s.e.m. (df 19) | Cov | Probability | ||||||
---|---|---|---|---|---|---|---|---|---|---|
ModE-LP | ModE-HP | HighE-LP | HighE-HP | Energy (ME) | Phos (P) | ME × P | ||||
n | 10 | 10 | 9 | 8 | – | – | – | – | – | |
Cortical bone thickness (mm) | ||||||||||
Initial | 3.76 | 3.98 | 3.54 | 3.66 | 0.146 | – | n.s. | n.s. | n.s. | |
Final | 3.33 | 3.61 | 3.28 | 3.83 | 0.135 | 1 | n.s. | <0.05 | n.s. | |
Change | −0.41 | −0.13 | −0.46 | +0.10 | 0.135 | 1 | n.s. | <0.05 | n.s. | |
P concentration (μg P/mL) | ||||||||||
Initial | 150 | 148 | 149 | 148 | 2.4 | – | n.s. | n.s. | n.s. | |
Final | 149 | 152 | 137 | 142 | 2.3 | 2 | <0.01 | n.s. | n.s. | |
Change | −1 | +4 | −12 | −7 | 2.3 | 2 | <0.01 | n.s. | n.s. | |
PSACB (μg P/mm2) | ||||||||||
Initial | 565 | 591 | 527 | 533 | – | – | – | – | – | |
Final | 502 | 572 | 436 | 549 | 22 | 3 | n.s. | <0.05 | n.s. | |
Change | −63 | −19 | −91 | +16 | 20 | 3 | n.s. | <0.05 | n.s. | |
Cortical bone volume (100-Po) (%) | ||||||||||
n | 9 | 8 | 8 | 6 | – | – | – | – | – | |
Initial | 97.0 | 96.3 | 97.0 | 95.4 | – | – | – | – | – | |
Final | 96.6 | 98.1 | 93.8 | 96.6 | 0.57 | 4 | <0.05 | <0.05 | n.s. | |
Change | −0.4 | +1.8 | −3.2 | +1.2 | 0.58 | 4 | 0.09 | <0.001 | n.s. |
Covariates (cov): 1, initial cow cortical bone thickness; 2, initial P concentration; 3, initial PSACB; 4, cow age. ME × P, the interaction between metabolisable energy and phosphorus in the diet.
(a) The relationship between the initial P per unit surface area of cortical bone (PSACB) and the change in PSACB in cortical rib bone, and (b) the relationship between the initial (100-Po) and the change in (100-Po). (c) Measurements in trabecular bone tuber coxae of the initial bone volume:total volume (BV:TV) and the change in BV:TV, (d) the initial mean thickness of trabecular struts and the change in this mean thickness, and (e) the initial maximum thickness of trabecular struts and the average change in this maximum thickness, during the experiment are shown. Cows were fed four diets comprising ModE-LP (), ModE-HP (
), HighE-LP (
), and HighE-HP (
).
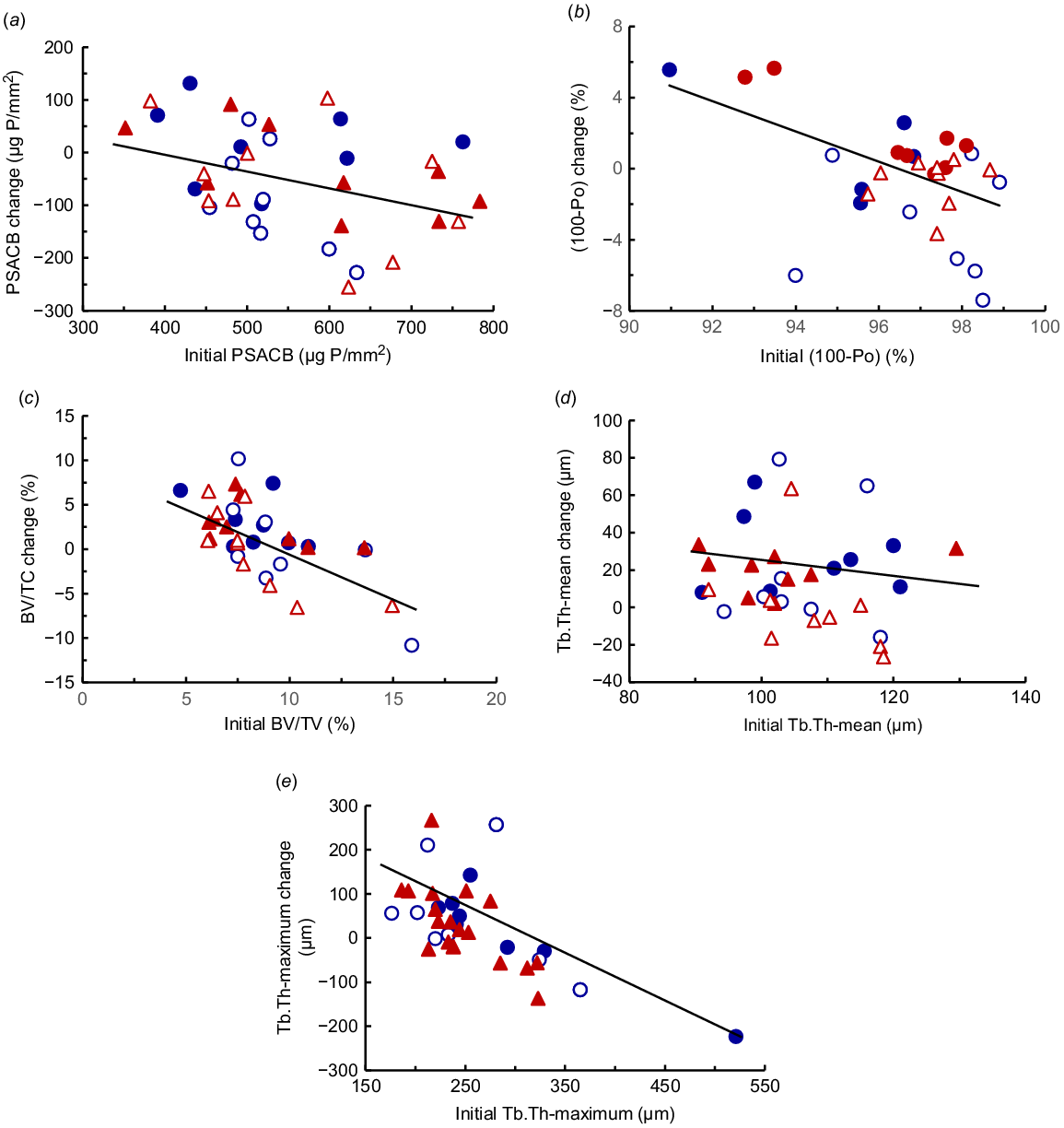
In tuber coxae trabecular bone, there was, as given above, high variation in the initial bone volume, mineralised bone volume and osteoid tissue in proportion to total bone volume. The variation in average trabecular strut thickness (107 ± 13 μm) was much lower than the variation in the maximum trabecular strut thickness (263 ± 65 μm). Examples of the histology of trabecular bone in representative cows from each diet treatment after 14 weeks are shown in Fig. 2. None of these attributes of trabecular bone was correlated with the PSACB of rib cortical bone. Changes in the attributes of trabecular bone were due to diet P rather than diet ME (Table 5). The bone volume:total volume increased by 36% in HP-diet cows (P < 0.05), but there was no change in LP-diet cows. Cows initially with low trabecular bone volume:total volume replenished more bone, whereas those initially with high bone volume:total volume mobilised more bone. Mineralised bone increased (P < 0.01) by 39% by the HP diets, but was not affected by diet ME. There were large changes in the amount of osteoid tissue and there was a diet ME × P interaction (P < 0.01). Osteoid volume increased by 51% in the HighE-LP diet cows and by 40–41% in the ModE-LP and ModE-HP diet cows, but was decreased by 55% in the HighE-HP diet cows. The cows fed the HighE-HP diet had thicker and more numerous trabecular struts than did those fed the ModE-LP diet (Fig. 2). The high proportion of osteoid and, therefore, poor bone mineralisation in the cows fed the LP diets (Table 5) occurred to a lesser extent in the cows fed the ModE-HP diet. Only in the cows fed the HighE-HP diet was there sufficient P substrate to allow mineralisation of the new bone formation. The average thickness of trabecular struts was increased by HP (P < 0.05) by 24%, and there was also a tendency (P = 0.07) for an increase in the maximum thickness of these struts. Furthermore, across all the treatments, the change in bone volume:total volume (Y3) was related to the initial bone volume:total volume (X3) (Fig. 1c). The initial mean thickness of trabecular struts (Y4) was related to the change in the mean thickness (X4) (Fig. 1d), and also the initial maximum thickness of trabecular struts was related to correlated the change in the maximum thickness (Fig. 1e) These regression relationships were as follows: Fig. 1c: (c) Y3 = 10.7 – 1.07 (X3) (n = 36, r = 0.64, P < 0.001); Fig. 1d: Y4 = 92 – 0.729 (X4) (n = 36, r = 0.34, P < 0.05); Fig. 1e: Y5 = 337 – 1.08 (X5) (n = 36, r = 0.65, P < 0.001).
The measurements showed that HP diets led to large increases in formation of trabecular bone, and that the gains or losses in individual cows were closely related to the amounts of bone at the commencement of the experiment.
Images of stained trabecular bone from the tuber coxae in the mature cows fed the diet treatments. The mineralised bone is shown in red, the unmineralised bone and osteoid are shown in blue. The cows were fed four diets either moderate or high in metabolisable energy and either with low or high P concentration in a 2 × 2 factorial design. (a) ModE-LP; (b) HighE-LP; (c) ModE-HP; and (d) HighE-HP.
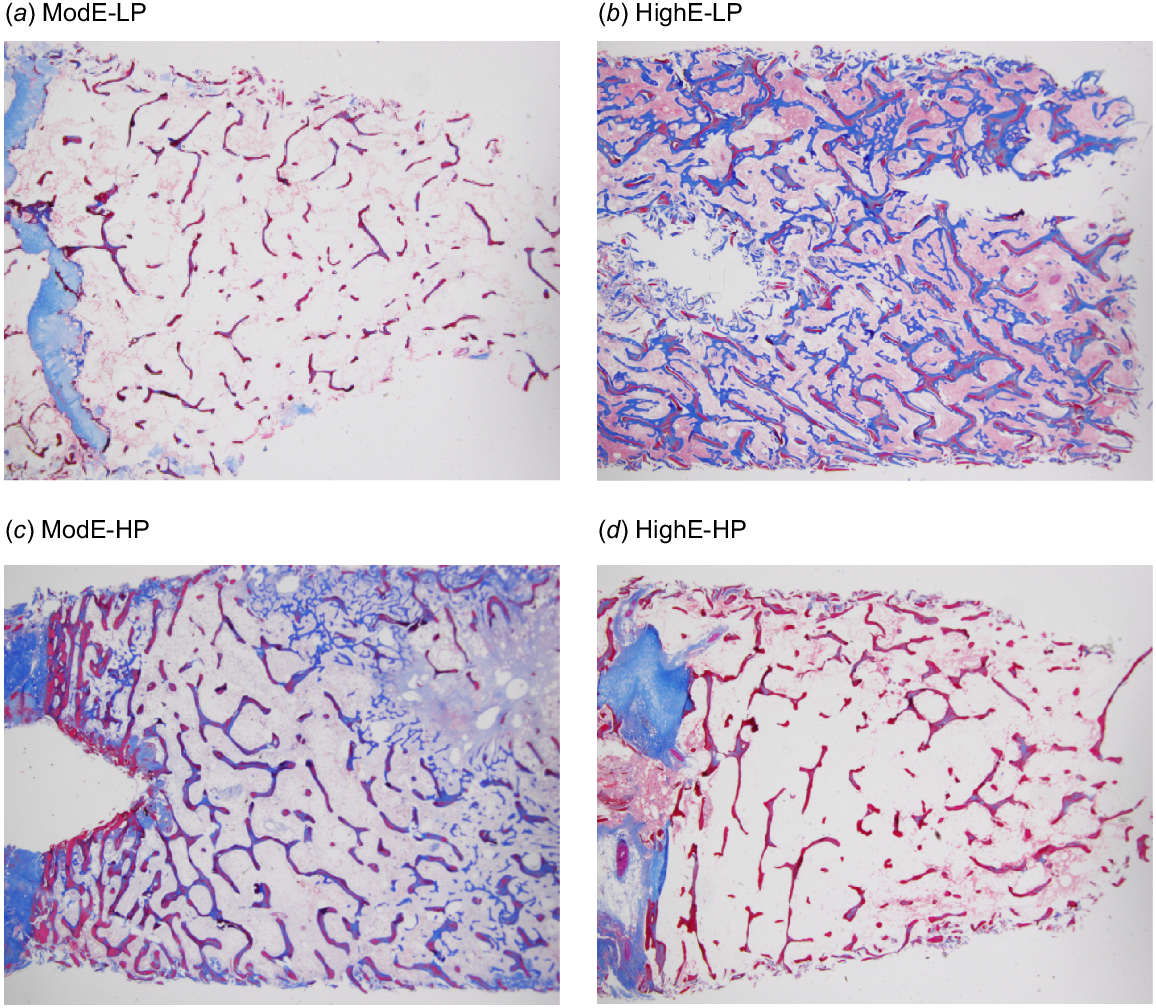
Measurement | Diet treatment | s.e.m. (df 19) | Probability | ||||||
---|---|---|---|---|---|---|---|---|---|
ModE-LP | ModE-HP | HighE-LP | HighE-HP | Energy (ME) | Phos (P) | ME × P | |||
n | 10 | 9 | 9 | 8 | – | – | – | ||
Bone volume to total volume (BV:TV) (%) | |||||||||
Initial | 8.36 | 8.34 | 9.79 | 8.30 | – | – | – | ||
Final | 8.40 | 11.36 | 10.17 | 11.23 | 0.73 | n.s. | 0.07 | n.s. | |
Change | +0.04 | +3.02 | +0.38 | +2.93 | 1.01 | n.s. | <0.05 | n.s. | |
Osteoid as a proportion of total volume (OV:TV) (%) | |||||||||
Initial | 1.64 | 1.35 | 2.12 | 2.02 | – | – | – | – | |
Final | 2.30b | 1.90b | 3.20c | 0.90a | 0.24 | n.s. | <0.001 | <0.01 | |
Change | +0.66 | +0.55 | +1.08 | −1.12 | 0.38 | n.s. | <0.05 | 0.07 | |
Mineralised bone as a proportion of total bone (Md.V:TV) (%) | |||||||||
Initial | 7.1 | 7.3 | 7.2 | 6.5 | – | – | – | – | |
Final | 6.4 | 9.1 | 6.8 | 10.3 | 0.54 | n.s. | <0.001 | n.s. | |
Change | −0.7 | +1.8 | −0.4 | +3.8 | 0.082 | n.s. | <0.01 | n.s. | |
Mean trabecular strut thickness (Tb.Th) (μm) | |||||||||
Initial | 113 | 103 | 107 | 107 | – | – | – | – | |
Final | 108 | 127 | 127 | 135 | 3.8 | 0.06 | 0.06 | n.s. | |
Change | −4 | +23 | +19 | +27 | 5.1 | n.s. | <0.05 | n.s. | |
Maximum trabecular strut thickness (Tb.Th-max) (μm) | |||||||||
Initial | 253 | 238 | 262 | 293 | – | – | – | – | |
Final | 254 | 316 | 306 | 303 | 13.8 | n.s. | n.s. | n.s. | |
Change | +4 | +35 | +26 | +12 | 14.2 | n.s. | n.s. | 0.07 |
Means in the same row with different lower-case letters are significantly different (at P < 0.05).
Plasma P, Ca and Mg concentrations
The Pi decreased rapidly when the LP diets were fed, and by Day 5, was lower (P < 0.05) in the LP than in the HP-diet cows (0.83 and 1.40 mmol/L respectively; P < 0.001) (Fig. 3a). There were effects of diet P over time on Pi (P < 0.001). The Pi in both LP diets continued to decrease (P < 0.05) and averaged 0.58 mmol/L during Weeks 10–14, whereas in the HP diets, Pi gradually increased and averaged 2.15 mmol/L during these weeks. The decrease in Pi at Week 8 in the HP diets coincided with a total faecal collection. There was no effect of the ME content of the diet on Pi (P > 0.05).
(a) The plasma P concentration (Pi), (b) plasma Ca concentration, and (c) plasma Ca:Pi ratio of cows fed four diets comprising ModE-LP (), ModE-HP (
), HighE-LP (
), and HighE-HP (
). In (a–c), the effects of diet P × time were significant (P < 0.001) and the least significant differences (l.s.d.) (5%) were 0.19, 0.11 and 0.86 for Pi, Ca and Ca:Pi respectively.
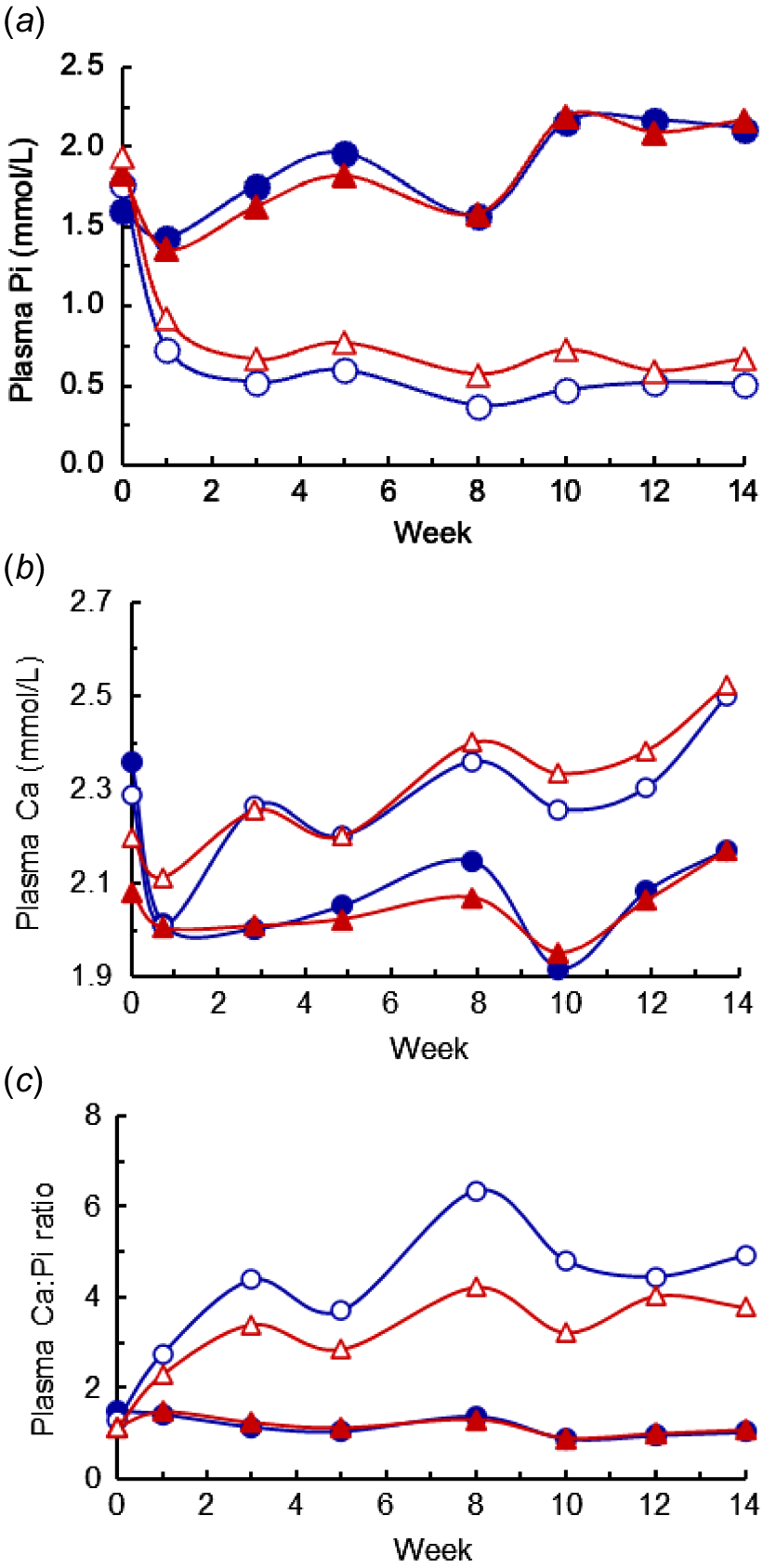
Mean plasma Ca concentrations were 2.23 ± 0.20 mmol/L before treatments commenced and the LP and HP diets generally showed opposite responses to the Pi, although through only a narrower range (Fig. 3b). The diet P × diet ME interaction approached significance (P = 0.06). By Week 3, the plasma Ca was higher in the LP- than in the HP-diet cows (means 2.26 and 2.00 mmol/L respectively; P < 0.001), and was higher at all sampling times thereafter. In cows fed the LP diets, the plasma Ca averaged 2.38 mmol/L during Weeks 10–14. There was no effect (P > 0.10) of diet ME on plasma Ca. Plasma Mg concentrations (data not shown) followed the same pattern as plasma Ca, with similar trends and differences between the LP and HP diets. The plasma Ca:Pi ratio averaged 0.81 ± 0.24 before treatments commenced and by Day 5 was higher (P < 0.001) in the LP than in the HP diets (Fig. 3c). There were diet P effects over time on the Ca:Pi ratio (P < 0.001). The plasma Ca:Pi ratio during Weeks 10–14 averaged 3.5 and 4.3 in cows fed the ModE-LP and HighE-LP diets respectively, compared with 1.1 in the two HP diets.
Endocrine and bone markers
Plasma parathyroid hormone (PTH) concentrations were highly variable within treatments, particularly before and shortly after the diet treatments commenced (Fig. 4). Three days before the treatments commenced, the mean PTH concentration averaged 147 pg/mL. From Week 3 to Week 14, the PTH concentrations were much higher (P < 0.05) in the HP- than the LP-diet cows (means 184 and 30 pg/mL respectively), but there was no effect (P > 0.05) of the ME content of the diet. At Week 14, the plasma PTH concentrations were negatively correlated with plasma Ca concentrations, as follows:
The plasma parathyroid hormone (PTH) concentrations of cows fed four diets comprising ModE-LP (), ModE-HP (
), HighE-LP (
), and HighE-HP (
). The effects of diet P × time were significant (P < 0.05) and the l.s.d. (5%) to compare the mean LP and HP diets was 0.31. The values presented have been back-transformed.
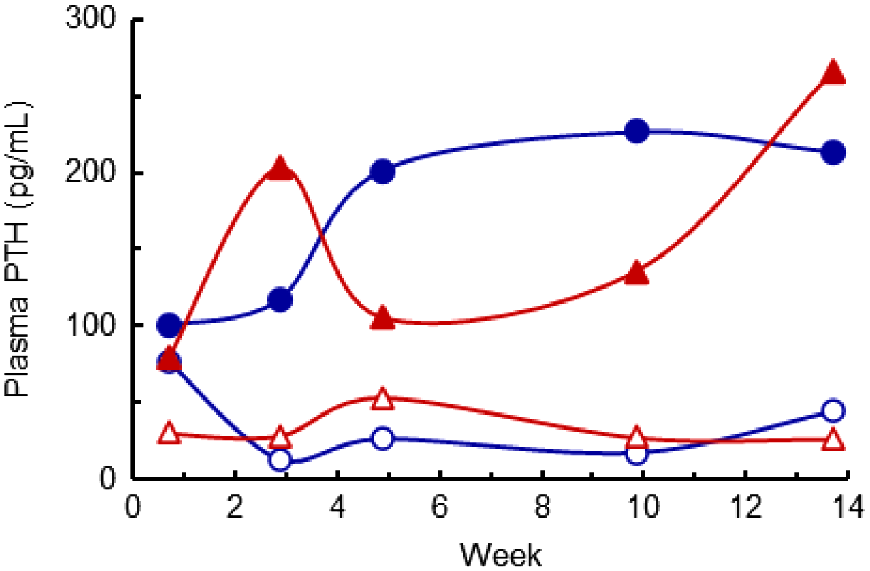
Plasma concentrations of the precursor 25-hydroxy vitamin D3 (data not shown) were not affected by the diets, averaging 27 ± 8 ng/mL at Day 5 and 33 ± 9 ng/mL at Week 14. The plasma concentrations of active 1,25-dihydroxy vitamin D3 (data also not shown) averaged 155 ± 82 ng/mL on Day 5. After 14 weeks, the 1,25-dihydroxy vitamin D3 concentrations in HP-diet cows (mean 118 pg/mL) did not differ from those at Day 5. However, in cows fed the ModE-LP and HighE-LP diets, the 1,25-dihydroxy vitamin D3 concentrations were increased (P < 0.001) to 252 pg/mL and 332 pg/mL respectively. The ratio of active to precursor vitamin D3 was higher (P < 0.05) in cows fed the HighE-LP diet (0.120) than in those fed the ModE-LP diet (0.084), and both were higher than that in the HP-diet cows (0.035 and 0.042; P < 0.05).
Plasma CTX-1 concentrations before treatments commenced averaged 0.57 ± 0.31 ng/mL, on Day 5 averaged 0.93 ng/mL, and did not differ among the diets (Fig. 5a). Thereafter, plasma CTX-1 concentrations were affected by diet P (P < 0.001) and diet ME (P < 0.05), and there was a diet P × time interaction (P < 0.001). From Week 3 to Week 14, the CTX-1 concentrations were higher (P < 0.001) in the LP- than in the HP-diet cows (2.27 and 0.64 ng/mL respectively). Also, during Weeks 10 and 14, the CTX-1 concentrations in the ModE-LP diet were higher than those in the HighE-LP diet (3.40 and 2.40 ng/mL respectively, P < 0.05). The CTX-1 (ng/mL) measured after 14 weeks was negatively correlated with Pi (mmol/L) and positively correlated with both the plasma Ca (mmol/L), and the plasma Ca:Pi ratio, as follows:
(a) The plasma CTX-1 concentration, (b) plasma OCN concentration, (c) plasma CTX-1:OCN ratio ands (d) plasma bone alkaline phosphatase (BAP) concentrations of cows fed four diets comprising ModE-LP (), ModE-HP (
), HighE-LP (
), and HighE-HP (
). In (a), the effects of diet P, diet metabolisable energy (ME), time, and the diet P × time interaction were significant (P < 0.05 or lower), and the l.s.d. (5%) of the diet P × time interaction = 0.63. In (b), the diet P × time interaction was significant (P < 0.001), and the effects of diet ME and the diet P × diet ME × time interaction both approached significance (P = 0.06, P = 0.09). The l.s.d. (5%) of the latter interaction = 12.6. In (c), the main effects and interactions, including the diet P × diet ME × time interaction, were significant (P = 0.01; l.s.d. (5%) = 0.020). In (d), the effects of diet P, diet ME, time, diet ME × time and the diet P × time interaction were significant (P < 0.05 or lower), and the diet P × diet ME × time interaction approached significance (P = 0.10, l.s.d. (5%) = 15.6).
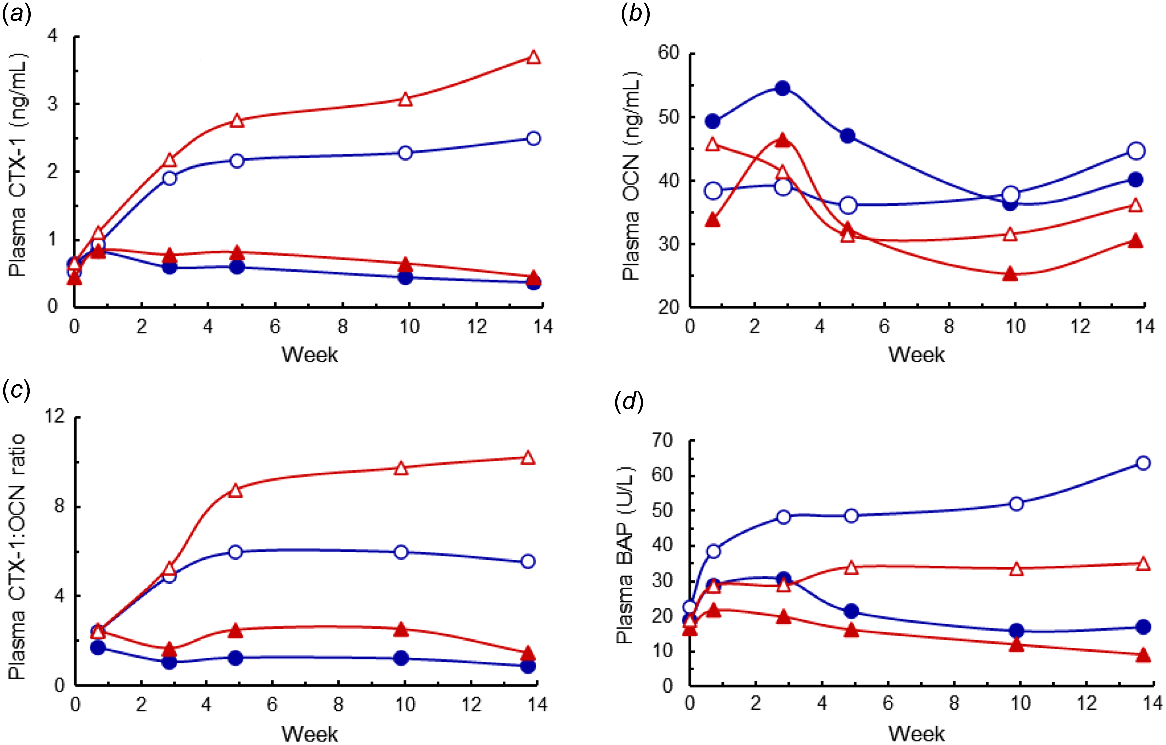
Plasma OCN concentrations were affected by time and the diet P × time interaction (both P < 0.001) (Fig. 5b). On Day 5 and Week 3, plasma OCN concentrations averaged 44 ng/mL, and then decreased (P < 0.05) to an average of 36 ng/mL from Week 5 to Week 14. After 3 weeks, the OCN concentrations were higher in the HP- than LP-diet cows (P < 0.05). In addition, higher diet ME tended (P = 0.06) to increase the OCN concentrations. The CTX-1:OCN ratios over time (Fig. 5c) showed a similar pattern to that of the CTX-1 concentrations. In addition, because the CTX-1 increased and the OCN tended to decrease owing to the treatment effects, the differences owing to P and to higher ME were greater than those for the CTX-1 concentrations. However, there appeared to be little advantage to using the CTX-1:OCN ratio rather than the CTX-1 concentration as an indicator of net bone P mobilisation.
Three days before the treatments commenced, the plasma BAP concentrations averaged 19 ± 7 U/L. The BAP concentrations were affected by the diet P × time and diet ME × time interaction (P < 0.001 and P < 0.05 respectively), and the diet P × diet ME × time interaction also approached significance (P = 0.10) (Fig. 5d). In each of the diets, the BAP concentration increased, or tended to increase, until Week 3. In the two HP diets, BAP then decreased progressively during the treatment period. In the HighE-LP diet, BAP increased markedly, whereas there was little change in the ModE-LP diet beyond Week 3. Average BAP concentrations from Week 10 to Week 14 were lowest in the two HP diets (mean 14 U/L), were increased (35 U/L) in the LowE-LP diet, and highest in the HighE-LP diet (58 U/L). ALP concentrations in plasma (data not shown) were generally consistent within each treatment from Week 5 to Week 14, and from Weeks 9 and 14 were higher (P < 0.05) in the LP-diet cows than in the HP-diet cows (means 145 and 76 U/L respectively). However, six cows had persistent high ALP concentrations (>200 U/L). Plasma concentrations of BAP were poorly correlated with those of ALP (r = +0.14, P = 0.41). Plasma AST concentrations were not affected by diet treatment (P > 0.05) and were, except for one sample in one animal, <170 U/L. This indicated that all the cows had normal liver function during the experiment and notably AST did not aid in diagnosis of the high ALP concentrations in the six cows.
Plasma IGF-1 concentrations were affected by the diet P × time and the diet ME × time interactions (P < 0.001 and P < 0.01 respectively) (Fig. 6). The IGF-1 concentrations were generally stable within treatments from Week 3 to Week 14, and during this interval, the higher diet P increased IGF-1 from 55 to 121 ng/mL, and higher diet ME increased IGF-1 from 63 to 126 ng/mL respectively. The mean IGF-1 concentrations at 10 and 14 weeks were correlated with the average ME intake (n = 40, r = +0.65, P < 0.01).
The plasma IGF-1 concentrations in cows fed four diets comprising ModE-LP (), ModE-HP (
), HighE-LP (
), and HighE-HP (
). The diet P × time and the diet metabolisable energy × time interactions were significant (P < 0.01 or lower), and the l.s.d. (5%) of the log(X + 1)10 transformed data was 0.14. The values presented have been back-transformed.
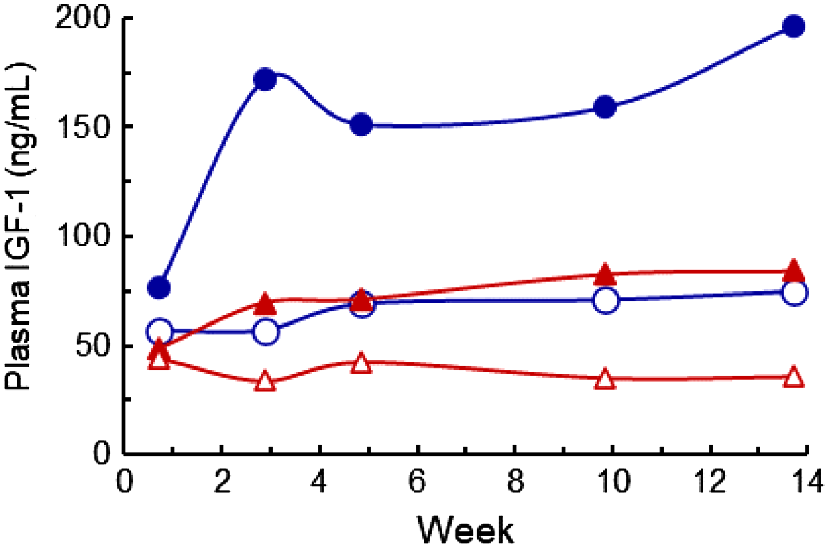
Prediction of P retention, plasma markers and bone PSACB from other measurements
There were linear relationships between the P retention and the measured P intake during each of the total collections at Weeks 3, 8 and 14 (R2 = 0.76, 0.71 and 0.59 respectively) and for the mean P retention and intake (R2 = 0.75) (Table 6). No other measured variable improved prediction of P retention in multiple-regression models, and the prediction of P retention was also not improved when expressed as mg P/kg LW.day (data not shown). Phosphorus retention was related to Pi as follows:
Dependent variable | Constant | Independent variable | R2 | s.e. | |||||
---|---|---|---|---|---|---|---|---|---|
P intake (g P/day) | PSACB (μg P/mm2) | MEI (kJ ME/kg LW.day) | Cow initial LW (kg) | Cow LW change (kg/day) | |||||
P retention (g P/day), Week 3 | |||||||||
Y1 | −5.196*** | 0.726*** | – | – | – | – | 75.7 | 2.41 | |
P retention (g P/day), mean | |||||||||
Y2 | −7.14*** | 0.829*** | – | – | – | – | 75.0 | 3.41 | |
Plasma Pi (mmol/L), mean | |||||||||
Y3 | 0.563*** | 0.0746*** | – | – | – | – | 58.7 | 0.44 | |
Y3 | −0.461n.s. | 0.128*** | 0.00159** | – | – | −0.874** | 69.7 | 0.381 | |
Y3 | 3.151*** | 0.129*** | – | −0.0131*** | −0.00286* | – | 85.7 | 0.261 | |
Plasma CTX-1 (ng/mL), mean | |||||||||
Y4 | 2.825*** | −0.128*** | – | – | – | – | 52.4 | 0.865 | |
Y4 | −2.930* | −0.194*** | – | 0.0153** | 0.00989** | – | 70.0 | 0.686 | |
Plasma Ca:Pi ratio, mean | |||||||||
Y5 | 4.673*** | −0.195*** | – | – | – | – | 42.3 | 1.60 | |
Y5 | 8.230*** | −0.208*** | −0.0062** | – | – | – | 52.7 | 1.45 | |
Y5 | 8.920*** | −0.418*** | −0.0066*** | – | – | 3.630** | 63.9 | 1.26 | |
Y5 | 3.000(−) | −0.338*** | −0.00337(-) | 0.0339*** | – | – | 69.5 | 1.16 |
LW, liveweight; PSACB, phosphorus per unit area of outer cortical bone; MEI, metabolisable energy intake.
n.s. not significant; (−), P < 0.10; *P < 0.05; **P < 0.01; ***P < 0.001.
Plasma Pi was related to P intake at each of the total collection intervals (R2 = 0.62, 0.45 and 0.58 respectively) and the mean of these intervals (R2 = 0.59) (Table 6). The prediction of mean Pi was improved in multiple-regression models that included both P intake and the initial PSACB as independent variables (R2 = 0.70). It was also improved by inclusion of both the measured initial cow LW, and the ME intake during the experiment, as independent variables (R2 = 0.86). The initial PSACB was correlated with ME intake (r = 0.33, P < 0.05), but not with the initial cow LW. There was no correlation between the initial cow LW and the ME intake (P > 0.05).
Plasma CTX-1 and the Ca:Pi ratio were both related to the P intake (R2 = 0.52 and 0.42, P < 0.001 for both) (Table 6). The prediction of CTX-1 was improved by inclusion of the initial cow LW and ME intake as additional variables (R2 = 0.70). Similarly, the prediction of the Ca:Pi ratio was improved by inclusion of PSACB (R2 = 0.70) and by inclusion of both PSACB and cow LW change, or of PSACB and ME intake, as additional variables (R2 = 0.70) (Table 6).
The high variability of the PSACB of the cows at the commencement of the experiment had significant effects on the responses of the cows as plasma Pi, plasma Ca:Pi and P retention. Inclusion of the initial PSACB as an independent variable in a multiple-regression model improved (P < 0.001) the prediction of Pi both at the Week 3 total collection and, on average, over the 14 weeks by 0.062 and 0.053 mmol/L for each 100 μg P/mm2 increase in initial PSACB respectively. Thus, the Pi at the Week 3 total collection, and the mean Pi over the entire experiment increased by 0.22 and 0.18 mmol/L respectively, as the initial PSACB of the cows increased from 350 to 750 μg P/mm2. In contrast, the P retention at the Week 3 total collection was increased 0.84 g P/day (1.75 mg P/kg LW.day) for each 100 μg P/mm2 decrease in the initial PSACB. It follows that at Week 3 the cows fed the ModE-HP diet with the lowest initial PSACB would have retained ~2.3 g P/day, whereas those with the highest initial PSACB would have retained ~1.1 g P/day; these are the highest and lowest P retentions respectively, compared with the mean of 1.7 g P/day during the Week 3 total collection (Table 3). There were similar effects of the initial PSACB (in Week 3; P < 0.001), in the Week 8 and Week 14 total collections, and for the average for the three total collection intervals (P < 0.10) on the plasma Ca:Pi, which increased as the initial PSACB decreased (Table 6).
Discussion
This study has clearly shown that mature cows in their second trimester of pregnancy fed acutely P-deficient diets mobilise substantial body P (~4–5 g P/day) to alleviate the effects of the P deficiency. Because mobilised P is presumably entirely available to the animal, this mobilisation would have corresponded to ~6–7 g of dietary P (CSIRO 2007). However, these LP cows were still P deficient because their voluntary intakes and their efficiency in utilisation of ingested ME were substantally lower than in the HP cows. The measured changes in rib and tuber coxae bone, and in plasma minerals and hormones (Pi, Pi:Ca ratio, 1,25-dihydroxy vitamin D3, CTX-1 and BAP) were informative of the changes in P metabolism in the cows.
Because the cows were previously lactating, and grazing native pastures growing on soils that were classed as deficient or acutely deficient through a rainy season (December 2013 – June 2014), it was expected that they would be P deficient and have low bone mineral reserves at the commencement of the experiment (McCosker and Winks 1994; Jackson et al. 2023). This was supported by the low Pi of the cows on 5 May 2014 (1.22 ± 0.48 mmol/L). However, at the commencement of the experiment, the bone measurements (PSACB in rib cortical bone and bone volume:total volume in tuber coxae rib bone) in the cows were highly variable. The coefficient of variation of the PSACB, bone volume:total volume and mineralised bone volume:total volume ranged from 21% to 32%. This was much higher than the coefficient of variation that we have observed in the PSACB of other groups of mature cows (10% and 15%) or in the bone volume:total volume of pregnant heifers (8%) (Dixon et al. 2020b). In addition, based on rib bone measurements in mature cattle fed P-adequate or P-deficient diets for extended periods, PSACB classes of <550, 550–650 and >650 μg P/mm2 are indicative of deficient, marginal and adequate bone P status respectively (Dixon et al. 2019a). By these criteria the PSACB measurements at the commencement of the present study indicated that only 57% of the cows were in deficient bone status, whereas 27% and 16% of the cows were in marginal and adequate bone P-status classes respectively. Furthermore, it is clear from the relationships shown in Fig. 1 that the initial bone mineral reserves, as measured by PSACB of rib bone and bone volume:total volume and thickness of the struts of trabecular bone, substantially affected the responses of the cows to the diet treatments in the present study.
Nutrient intakes and LW change of the cows
The MedE-HP and HighE-HP diets provided for slow and rapid LW gains (0.32 and 1.05 kg/day respectively) that were representative of low and high post-weaning performance of breeder cows grazing pasture ranging from moderate to high quality during the late-rainy into the early dry seasons in seasonally dry tropical rangelands (Holroyd et al. 1983, 1988; Dixon et al. 2011a, 2011b). The low Pi, voluntary intakes, P retentions, metabolites in plasma and LW gain in the LP-diet cows, and the responses to additional diet P, provided unequivocal evidence that the cows fed the LP diets were acutely P deficient (Wadsworth et al. 1990; Winks 1990; Dixon et al. 2020a). Furthermore, the diet P concentrations and P intakes of the LP-diet cows (0.68 and 0.76 g P/kg DM, 7–9 mg P/kg LW.day) fed in pens were comparable with P intakes measured in field experiments with growing cattle and breeder cows grazing native pastures classed as ‘acutely deficient’ or ‘deficient’ for northern Australia (McLean et al. 1990; Hendricksen et al. 1994; Ternouth and Coates 1997; Miller et al. 1998) and South Africa (Read et al. 1986a, 1986b, 1986c). In contrast, from the P intakes relative to the calculated requirements, and the high Pi, the supply of absorbed P was not likely to have limited either LW gain or body P replenishment in the HP-diet cows. Thus, the LP and HP diets did represent the P intakes of pregnant cows shortly after weaning during the late rainy season and the early dry season grazing P-deficient pastures of at least moderate quality, or such pasture supplemented with P, as classed in the P-management packages described by McCosker and Winks (1994) and Jackson et al. (2023). However, in some circumstances cows may be losing LW in the late rainy and early dry seasons because of poor seasonal conditions and/or when weaning is delayed until the mid- to late dry season (Holroyd et al. 1983, 1988; Dixon et al. 2007). When nutrient intake is insufficient for LW maintenance, the cow responses to supplementary P may well be lower than observed for the cows fed the moderate-ME diets in the present experiment.
The large responses to providing HP that we observed on voluntary intakes of DM and ME, and LW gain, are in general agreement with pen and grazing experiments reported for growing cattle ingesting P-deficient diets (Winks 1990; Bortolussi et al. 1996; Quigley et al. 2015). The observed responses were also consistent with reduced LW loss, and large increases in LW gain and reproductive performance, in field experiments with beef breeder cows where the benefits of additional diet P were associated with increases in voluntary intake and cow LW (Winks 1990; Winter et al. 1990; Ternouth and Coates 1997; Coates et al. 2019; Schatz et al. 2023). We are not aware of any previous research under pen conditions in non-lactating pregnant beef cows where the diet was closely controlled and the responses by P-deficient cows to additional diet P could be accurately measured.
The gradual decrease in voluntary intake of each of the diets by the cows during the present experiment was most likely to be a consequence of weaning shortly before the experiment commenced. In Bos indicus cows fed forage diets, the voluntary intake is usually increased by 20–30% during lactation (Penzhorn and Meintjies 1972; Hunter and Siebert 1986), and following weaning, gradually decreases over several months (Foote and Russel 1979). A carry-over effect of lactation on intake also likely contributed to the high voluntary intake (mean 21.5 g DM/kg LW.day) and high LW gain (mean 1.05 kg/day) of the cows fed the HighE-HP diets.
The observed higher efficiency in the utilisation of ingested ME in cows fed the ModE-HP diet than in those fed the ModE-LP diet was unexpected, but is the only explanation for the similar LW gains of the cows in these two treatments (0.32 and 0.29 kg/day), even though the HighE-LP diet cows consumed 29% more ME (Table 2). This result is in accord with slower growth over 40 weeks in P-deficient than in P-adequate heifers with similar ME intakes reported by Gartner et al. (1982). Severe P deficiency has been associated with a large decrease (~30%) in the P concentration of soft tissues in growing lambs (Ternouth and Sevilla 1990) and there is evidence that cellular metabolism, including of energy, is impaired by severe P deficiency (Henry et al. 1979; Wang et al. 1985; Ternouth 1989). Adverse effects on cellular metabolism may explain both the usual decreases in voluntary intake (Winks 1990), and a reduced efficiency in utilisation of ME, in severely P-deficient animals. A 30% increase in efficiency in utilisation of ME for maintenance and growth is clearly a very important production benefit if it does occur generally when P-deficient cattle are fed adequate diet P. Further information is needed to test this hypothesis.
Mobilisation of body P by the cows fed low-P diets
Even though some of the cows had partially depleted body P reserves at the start of the experiment, the LP-diet cows were still able to mobilise, on average, 4.1 and 4.7 g P/day (9.3 and 11.2 mg P/kg LW) of body P in the ModE-LP and HighE-LP diets respectively). This P corresponded to 55% and 45% of their calculated P requirements when the cows were ingesting <50% of their P requirements. Furthermore, over the 14-week experimental interval this corresponded to the mobilisation of ~12–14% of the body P reserves that was in addition to the mobilisation of body P that is likely to have occurred during the preceding lactation in these cows. The P mobilisation we observed was consistent with the decreases in PSACB, changes in the porosity of rib cortical bone, and changes in trabecular bone and osteoid in tuber coxae bone (Tables 4, 5) in the LP-diet cows. The much larger proportional changes in trabecular than cortical bone were consistent with the principle that during bone mobilisation and accretion, the changes in bone are much greater in trabecular bone with a large surface area, than in cortical bone (Seeman 2013; Winter et al. 2020). However, because cortical bone and trabecular bone represent about 80% and 20% respectively, of the total bone, a small change in cortical bone may be more important than a large change in trabecular bone on the total bone in the animal. Furthermore, the cortical thickness is a linear measure whereas the change in total rib bone in the animal is a volume. Hence, in the present study the small percentage changes in cortical bone thickness or PSACB represented greater changes in the total bone of the cows than the same percentage changes in the trabecular bone. A further consideration is that, in the present study, the diet treatments were imposed for only 14 weeks, which is only a short interval during the annual cycle for substantial changes to occur in bone.
The relationships between the amounts of bone initially present in the rib cortical bone and in tuber coxae trabecular bone, and the changes in both cortical and trabecular bone during the experiment (Fig. 1a–e) provided unequivocal evidence that the extent bone is gained or lost during P deficiency or P adequacy is highly dependent on the bone mineral status of the animals. The multiple regression modelling (Table 6) showed that the bone mineral status, measured as PSACB, contributed significantly to the prediction of plasma Pi and plasma Ca:Pi and is in accord with the important role of the bone minerals status. The hypothesis was also supported by the observation that inclusion of the initial PSACB as a covariate significantly (P < 0.001) increased the estimated Pi both at the total collection at Week 3, and, on average, over the 14 weeks, by 0.062 and 0.053 mmol/L for each 100 μg P/mm2 increase in initial PSACB respectively. Thus, the Pi at the Week 3 total collection, and the mean Pi over the entire experiment, increased by 0.22 and 0.18 mmol/L respectively, as the initial PSACB of the cows increased from 350 to 750 μg P/mm2. However, clearly, there must be limits to the rates and extent of bone mineral mobilisation as the bone reserves decline.
The cows were expected to have been in P-replete status in December 2013. A 450 kg P-replete cow is expected to have body reserves of ~8.9 g P/kg empty bodyweight (ARC 1980) (~3.4 kg P with 2.7 kg in bone and 0.7 kg in soft tissues). Since P-replete mature cows are expected to have a PSACB of 700–750 μg P/mm2 (Dixon et al. 2019a), the average 546 μg P/mm2 PSACB in June 2014 suggested that these cows had lost ~20–25% of their bone P reserves from December 2013 to June 2014, corresponding to mobilising ~4–5 g body P/day. This estimate is remarkedly similar to the mobilisation of P in the LP-diet cows measured as P retention during the experiment. Moreover, these calculations are consistent with the conclusion that cattle lose up to ~30% of their bone P reserves during part of their annual cycle, and in some circumstances over 40% of their bone P reserves, before becoming overtly P deficient and showing clinical signs of osteomalacia (Little 1983; Read et al. 1986a, 1986b, 1986c; Dixon et al. 2017, 2020a). The P-mobilisation rates in the LP-diet cows in the present experiment compare with 7–9 mg P/kg LW.day of P mobilisation in steers fed acutely P-deficient diets (Tuen et al. 1984; Bortolussi et al. 1996; Ternouth et al. 1996). More generally, it appears that in mature non-lactating beef cows, the maximum body P mobilisation is ~5 g P/day (11 mg P/kg LW), which will correspond to ~7 g diet P.
Replenishment of body P by the cows fed P-adequate diets
The histological changes and the changes in tuber coxae bone as increased bone volume (~36%), mineralised bone volume (~42%) and thickening of trabecular struts (~24%) (Table 5) clearly showed that there was increased trabecular bone formation when the cows were fed HP diets. The importance of availability of P as a metabolic substrate for this bone formation was shown by the response to the HighE-LP diet where trabecular thickness increased but was poorly mineralised. The large (55%) decrease in osteoid tissue in the HighE-HP diet cows was presumably because only this diet provided sufficient P to allow a large increase in bone mineralisation. The increase in mineralisation when ModE-HP was fed (25%) was much lower than the 58% increase with the HighE-HP diet. These measurements indicated that trabecular bone growth and mineralisation were proceeding in the cows fed the higher-ME or high-P diets, but occurred much more rapidly in the HighE-HP diet cows. This was in accord with the much lower P retention in the ModE-HP than the HighE-HP diet cows.
The changes in the rib cortical bone owing to the diet treatments were, as expected, smaller than those observed in trabecular bone in the tuber coxae. High diet P increased the cortical bone thickness, whereas high diet ME led to a small decrease in the P concentration of the cortical bone. As in tuber coxae bone, the larger effects were due to providing adequate diet P rather than to increasing the ME content of the diet. Also, the changes in PSACB were a consequence of the changes in thickness rather than in P concentration of cortical bone. This is consistent with the paradigm (Shupe et al. 1988; Breves and Prokop 1990; Coates et al. 2018) that loss of cortical rib bone in mature cows during extended P deficiency is associated with thinning of cortical bone rather than decrease in its mineral concentration. The increase in PSACB in cows fed the HighE-HP diet (16 μg P/mm2) was small compared with the decrease (mean 77 μg P/mm2) in the LP-diet cows (Table 4). The benefit of the HP diets on rib cortical bone was to maintain rather than to increase this bone. The small response may have been associated with a time lag for cows to change from net mobilisation during P deficiency to net replenishment in rib cortical bone when the HP diets are fed; 14 weeks is a short interval in the annual cycle. Nevertheless, the substantial increase in (100-Po) (i.e. the decrease in porosity) of the rib cortical bone in the HP diets, and the changes in the tuber coxae bone, established that there was increased formation as ‘infilling’ of porotic spaces in cortical bone concurrently with the increased growth and mineralisation of trabecular bone in the tuber coxae.
The high P retention (+8.8 g P/day) in cows fed the HighE-HP diet (Table 2) was greater than the amount of absorbed P (6.4 g P/day) calculated to be required for their 1.05 kg/day LW gain (CSIRO 2007). Hence, ~2.4 g absorbed P/day should have been available for replenishment of P concentration in soft body tissues and bones. The small positive average P retention in cows fed the ModE-HP diet (+1.1 g P/day) showed that there was a slow increase in body P reserves, even when ME intake limited the cows to only slow LW gain. As the difference between two large flows as P intake and faecal P excretion, the measurement of P retention is inevitably subject to substantial error. However, the error associated with measurements of P retention in the present experiment (s.e.m. 2.7 mg P/kg LW.day) was lower than that often reported previously, ranging from 1 to 8 mg P/kg LW.day (Tuen et al. 1984; Bortolussi et al. 1992, 1996; Knowlton and Herbein 2002).
The modest average replenishment of the P reserves in the cows fed the ModE-HP diet in the present experiment was likely to be associated with only about half (57%) of the cows being deficient in bone minerals at the commencement of the experiment as discussed above, and also the mature ages of the cows (Table 6). In addition, there may also have been limitations to bone mineral replenishment associated with the principle that the structural strength of bones and bone minerals change as needed to support the increasing or decreasing weight of the animal (Siemon and Moodie 1973). Because the cows fed the ModE-HP diet were in slow growth, whereas the HighE-HP cows gained LW rapidly during the experiment, there was likely to be lesser endocrine signalling in the former cows to increase bone mass (Iwaniec and Turner 2016). Large decreases in LW in growing steers during extended dry seasons have been associated with decreased rib bone minerals (Winter 1988). Furthermore, as would be expected, and observed in the present experiment, the rate and extent of body P replenishment will be affected by the magnitude of a depletion in body P reserves as well as intakes of P and other nutrients. This is supported by the study of Schild et al. (2023) where beef breeder cows with clinical osteomalacia from grazing acutely P-deficient pastures largely recovered from osteomalacia over 3 months when fed 8 g supplementary P/day. During recovery, the P-supplemented pasture was able to support 0.3 kg LW gain/day, the P supplementation increased Pi from 1.0 to 1.9 mmol/L, rib cortical bone P concentration increased from 85 to 131 mg/mL, and serum Ca:Pi decreased from 2.8 to 1.1. The bone mineral status of the cows was much lower than that of the cows in the present experiment, but, nevertheless, 90% of the cows recovered from clinical signs of osteomalacia. More generally, replenishment of 1.1–2.3 g/day body P (as measured in the present experiment) for 8 months from the mid-rainy season and through the dry season would contribute 260–550 g P to substantially replenish body P reserves. Even if insufficient to completely replenish the body P reserves of a 450 kg cow following mobilisation of 30% of body P reserves (~1 kg P) during pregnancy and lactation, this would make a substantial contribution to P status of cows.
Markers of the bone mineral mobilisation and replenishment and endocrine control
The plasma Pi in the HP-diet cows were high and within the range reported for growing cattle and non-lactating cows consuming P-adequate diets (Wadsworth et al. 1990; Anderson et al. 2017; Dixon et al. 2020a). The large decreases in Pi to 0.5–0.8 mmol/L when the LP diets were fed to the cows were expected and were comparable to those reported in severely P-deficient ruminants and following large abrupt decreases in diet P (Wadsworth et al. 1990; Rodehutscord et al. 1994; Dixon et al. 2020b). The rapid decreases in plasma Pi in the cows in the present experiment contrast with delays for many weeks or months for Pi to decrease in growing cattle fed P-deficient diets in some experiments (Gartner et al. 1982; Quigley et al. 2015; Dixon et al. 2019b), presumably owing to sufficient mobilisation of body P reserves to maintain Pi for extended intervals. In the present experiment, the concentrations of Ca and Mg in plasma were within the normal ranges for these minerals, and increases were consistent with their release into circulation with mobilisation of bone during P deficiency. The gradual increases in these minerals in the LP-diet cows as the experiment progressed were consistent with time lags and requirements for adaptation to the LP diets. Observed changes in plasma CTX-1, CTX-1:OCN ratio, and BAP concentrations support a hypothesis that mobilisation of bone increased gradually to provide P during severe P deficiency (Anderson et al. 2017). Although there was a rapid decrease within a week in plasma Pi, following the introduction of the LP diets, there were much more gradual increases in Ca and the other bone biomarkers during Weeks 2–5. It is likely that this delay reflected the time lag needed to recruit osteoclasts to enable bone mobilisation. This provides further evidence that the plasma Ca, CTX-1 and BAP provide reliable markers of bone mobilisation.
Interestingly, in the LP-diet cows, there was a large increase in active 1,25-dihydroxy vitamin D3 in plasma at Week 14 that was likely to be a direct effect of low Pi rather than a response to PTH; the concentrations of PTH were reduced in LP-diet cows. Furthermore, low plasma PTH concentrations in LP-diet cows were associated with high plasma Ca in accord with tight regulatory control between Ca and PTH concentrations. Increased plasma Ca in LP-diet cows may have been associated with increased bone mineral mobilisation and increased absorption from the gut in association with the adequate Ca content of the LP diets. Nevertheless, unlike during diet deficiencies of Ca, PTH appears not to have had a central role in the control of bone mobilisation in P-deficient cows. Rather, the evidence supports the hypothesis that increased active 1,25-dihydroxy vitamin D3 and/or other mediators are controlling P homeostasis and driving bone resorption in P deficiency.
It is well established that CTX-1, as a breakdown product of bone and cartilage during turnover, provides a marker of bone mobilisation (Anderson et al. 2017). The increases in CTX-1 in the LP-diet cows were in accord with these diets providing less than half of the calculated absorbed P requirements of the cows, with low Pi, with the substantial negative P retentions, and that these LP-diet cows were mobilising bone P. The CTX-1 concentrations measured in the current study, ranging from ~2.5 to 3.5 ng/mL after 10–14 weeks in the LP-diet cows, were much higher than those in the HP-diet cows, but were quite low compared with those in P-deficient beef and dairy cows in early to mid-lactation where CTX-1 concentrations are up to 6 ng/mL (Ekelund et al. 2006; Puggaard et al. 2014; Anderson et al. 2017; Dixon et al. 2020b). This supports a hypothesis that non-lactating cows have a lower potential than lactating cows to mobilise body P during P deficiency.
A potential simple alternative to CTX-1 as a marker of bone mineral mobilisation is the plasma Ca:Pi ratio. In mature lactating beef cows, there is a positive correlation between plasma CTX-1 concentrations and the plasma Ca:Pi ratio during P deficiency (Anderson et al. 2017). In the non-lactating cows in the present experiment, this relationship between plasma CTX-1 and the plasma Ca:Pi ratio was similar to that reported by Anderson et al. (2017), but through a smaller range and with a lower R2 (0.78 and 0.37 respectively). The present experiment supports the hypothesis that both plasma CTX-1 and Ca:Pi ratio can be used as markers of net bone mobilisation during P deficiency.
Both OCN and BAP are potential markers for bone growth, although the latter is indicative primarily of bone mineralisation (Naito et al. 1990; Anderson et al. 2017). In the present study, the concentrations of OCN were increased by the two HP diets, but they also tended to increase with higher diet ME content. Unfortunately, there was not a clear difference between the HighE-HP and the ModE-HP diets in OCN concentrations, even though P retention was much higher in the former diet. In contrast, there were large diet effects on BAP concentration. The increases in BAP as a bone formation marker during diet P deficiency appear contradictory. However, Anderson et al. (2017) argued that in the absence of sufficient P substrate in P-deficient animals, the formation of bone cannot proceed, and BAP (i.e. alkaline phosphatase isoform in bone) is upregulated to drive localised bone mineralisation. Therefore, high BAP concentrations appear to be primarily an indicator of defective mineralisation in P-deficient cows. In the present experiment, there were differences among diets in ‘total’ ALP concentration, but because this is a measure of alkaline phosphatases derived mainly from both bone and liver, there does not appear to be any advantage in use of ALP rather than BAP as a marker. The changes and differences among diets in OCN and in the CTX-1:OCN ratio were generally small and differences among treatment diets were often not clear. Therefore CTX-1 concentration alone appears to be the more useful biomarker for P deficiency. The increases in 1,25-dihydroxy vitamin D3 in plasma with P deficiency suggests that this also may provide a valuable hormonal marker. In summary, the concentrations of Pi, Ca, Ca:Pi, CTX-1, BAP and 1,25-dihydroxy vitamin D3 in plasma are all useful markers of P status, including in non-lactating beef breeder cows.
Conclusions
Mature Bos indicus-cross breeder cows fed acutely P-deficient diets mobilised substantial body P (~4–5 g P/day, equivalent to ~6–7 g diet P/day) to alleviate the effects of the deficiency, but both voluntary intake and the efficiency in utilisation of ingested ME were substantially reduced. Providing adequate diet P increased voluntary intake and LW gain, initiated growth of trabecular bone, and commenced replenishment of low body P reserves. The retention of P by cows fed a high-P diet but ingesting sufficient energy and protein for only slow LW gain was, on average, 1.1 g P/day, but was much higher in previously P-deficient cows with low bone mineral reserves. Responses by cows to adequate diet P were much greater when a higher ME-content diet allowed high voluntary intake and LW gain, increased P retention, replenishment of body P reserves, and increased bone growth. A number of metabolites (plasma Pi, Pi:Ca ratio, CTX-1, BAP and 1,25-dihydroxy vitamin D3) and measurements of rib and tuber coxae bone were informative of P metabolism in the cows, although testing over a wider range of circumstances is needed for their general application as diagnostics in P-deficient cows.
Data availability
The data that support this study may be shared upon reasonable request to the corresponding author.
Conflicts of interest
Dr Rob Dixon is an Associate Editor of Animal Production Science. To mitigate this potential conflict of interest he had no editor-level access to this manuscript during peer review. The authors declare that they have no further conflicts of interest.
Declaration of funding
Funding for this work was provided by Meat and Livestock Australia, by the Queensland Alliance for Agriculture and Food Innovation (QAAFI), the University of Queensland, and by the Queensland Department of Primary Industries.
Acknowledgements
We thank Tracy Longhurst, Don Cherry and the DAF team at the Brian Pastures Research Station for their assistance with the care, handling and sampling of the cattle in the experiment. We also thank Natasha Steiger, Jeremy Spiers and Nick Corbet (UQ), Michael Gravel and DPI technical staff for undertaking laboratory analyses.
References
Anderson ST, Kidd LJ, Benvenutti MA, Fletcher MT, Dixon RM (2017) New candidate markers of phosphorus status in beef breeder cows. Animal Production Science 57, 2291-2303.
| Crossref | Google Scholar |
Benzie D, Boyne AW, Dalgarno AC, Duckworth J, Hill R (1959) Studies of the skeleton of the sheep. III. The relationship between phosphorus intake and resorption and repair of skeleton in pregnancy and lactation. Journal of Agricultural Science 52, 1-12.
| Crossref | Google Scholar |
Bortolussi G, Ternouth JH, McMeniman NP (1992) The effects of dietary nitrogen and calcium supplementation on Bos indicus cross cattle offered low P diets. Proceedings of the Australian Society of Animal Production 19, 381-384.
| Google Scholar |
Bortolussi G, Ternouth JH, McMeniman NP (1996) Dietary nitrogen and phosphorus depletion in cattle and their effects on liveweight gain, blood metabolite concentrations and phosphorus kinetics. Journal of Agricultural Science 126, 493-501.
| Crossref | Google Scholar |
Bowen MK, Poppi DP, McLennan SR, Doogan VJ (2006) A comparison of the excretion rate of endogenous purine derivatives in the urine of Bos indicus and Bos taurus steers. Australian Journal of Experimental Agriculture 57, 173-177.
| Crossref | Google Scholar |
Breves G, Prokop M (1990) Dietary phosphorus depletion in sheep: long-term effects on bone structure. Journal of Animal Physiology and Nutrition 64, 74-79.
| Crossref | Google Scholar |
Callis G, Sterchi D (1998) Decalcification of bone: literature review and practical study of various decalcifying agents. Methods, and their effect on bone histology. Journal of Histotechnology 21, 49-58.
| Crossref | Google Scholar |
Chen XB, Meijia AT, Kyle DJ, Ørskov ER (1995) Evaluation of the use of the purine derivative: creatinine ratio in spot urine and plasma samples as an index of microbial protein supply in ruminants: studies in sheep. Journal of Agricultural Science 125, 137-143.
| Crossref | Google Scholar |
Coates DB, Dixon RM, Murray RM, Mayer RJ, Miller CP (2018) Bone mineral density in the tail-bones of cattle: effect of dietary phosphorus status, liveweight, age and physiological status. Animal Production Science 58, 801-810.
| Crossref | Google Scholar |
Coates DB, Dixon RM, Mayer RJ (2019) Between-year variation in the effects of phosphorus deficiency in breeder cows grazing tropical pastures in northern Australia. Tropical Grasslands- Forajes Tropicales 7, 223-233.
| Crossref | Google Scholar |
Dempster DW, Compston JE, Drezner MK, Glorieux FH, Kanis JA, Malluche H, Meunier PJ, Ott SM, Recker RR, Parfitt AM (2013) Standardized nomenclature, symbols, and units for bone histomorphometry: a 2012 update of the report of the ASBMR Histomorphometry Nomenclature Committee. Journal of Bone and Mineral Research 28, 2-17.
| Crossref | Google Scholar | PubMed |
Dixon RM, Smith DR, Coates DB (2007) Using faecal NIRS to improve nutritional management of breeders in the seasonally dry tropics. Recent Advances in Animal Nutrition in Australia 16, 135-145.
| Google Scholar |
Dixon RM, Playford C, Coates DB (2011a) Nutrition of beef breeder cows in the dry tropics. 1. Effects of nitrogen supplementation and weaning on breeder performance. Animal Production Science 51, 515-528.
| Crossref | Google Scholar |
Dixon RM, Playford C, Coates DB (2011b) Nutrition of beef breeder cows in the dry tropics. 2. Effects of time of weaning and diet quality on breeder performance. Animal Production Science 51, 529-540.
| Crossref | Google Scholar |
Dixon RM, Kidd LJ, Coates DB, Anderson ST, Benvenutti MA, Fletcher MT, McNeill DM (2017) Utilising mobilization of body reserves to improve the management of phosphorus nutrition of breeder cows. Animal Production Science 57, 2280-2290.
| Crossref | Google Scholar |
Dixon RM, Coates DB, Mayer RJ, Miller CP (2019a) Alternative rib bone biopsy measurements to estimate changes in skeletal mineral reserves in cattle. Animal 13, 119-126.
| Crossref | Google Scholar |
Dixon RM, Fletcher MT, Goodwin KL, Reid DJ, McNeill DM, Yong KWL, Petherick JC (2019b) Learned behaviours lead to bone ingestion by phosphorus deficient cattle. Animal Production Science 59, 921-932.
| Crossref | Google Scholar |
Dixon RM, Anderson ST, Kidd LJ, Fletcher MT (2020a) Management of phosphorus nutrition of beef cattle grazing seasonally dry rangelands: a review. Animal Production Science 60, 863-879.
| Crossref | Google Scholar |
Doube M, Kłosowski MM, Arganda-Carreras I, Cordelières FP, Dougherty RP, Jackson JS, Schmid B, Hutchinson JR, Shefelbine SJ (2010) BoneJ: free and extensible bone image analysis in ImageJ. Bone 47, 1076-1079.
| Crossref | Google Scholar | PubMed |
Ekelund A, Spörndly R, Holtenius K (2006) Influence of low phosphorus intake during early lactation on apparent digestibility of phosphorus and bone metabolism in dairy cows. Livestock Science 99, 227-236.
| Crossref | Google Scholar |
Foote JZ, Russel AJF (1979) The relationship in ewes between voluntary food intake during pregnancy and forage intake during lactation and after weaning. Animal Science 28, 25-39.
| Crossref | Google Scholar |
Gartner BJW, Murphy GM, Hoey WA (1982) Effects on induced, subclinical phosphorus deficiency on feed intake and growth of beef heifers. Journal of Agricultural Science, Cambridge 98, 23-29.
| Crossref | Google Scholar |
George SK, Dipu MT, Mehra UR, Singh P, Verma AK, Ramgaokar JS (2006) Improved HPLC method for the simultaneous determination of allantoin, uric acid and creatinine in cattle urine. Journal of Chromatography B 832, 134-137.
| Crossref | Google Scholar | PubMed |
Goodwin JF (1970) Quantification of serum inorganic phosphorus, phosphatase, and urinary phosphate without preliminary treatment. Clinical Chemistry 16, 776-780.
| Crossref | Google Scholar | PubMed |
Hart B, Mitchell GL (1965) Effect of phosphorus supplementation on the fertility of an open range beef cattle herd on the Barkly Tableland. Australian Veterinary Journal 41, 305-309.
| Crossref | Google Scholar |
Hendricksen RE, Ternouth JH, Punter LD (1994) Seasonal nutrient intake and phosphorus kinetics of grazing steers in northern Australia. Australian Journal of Agricultural Research 45, 1817-1829.
| Crossref | Google Scholar |
Henry Y, Gueguen L, Rerat A (1979) Influence of the level of dietary phosphorus on the voluntary intake of energy and metabolic utilization of nutrients in the growing rat. British Journal of Nutrition 42, 127-137.
| Crossref | Google Scholar | PubMed |
Holroyd RG, O’Rourke PK, Clarke MR, Loxton ID (1983) Influence of pasture type and supplement on fertility and liveweight of cows, and progeny growth rate in the dry tropics of northern Queensland. Australian Journal of Experimental Agriculture 23, 4-13.
| Crossref | Google Scholar |
Holroyd RG, Mason GWJ, Loxton ID, Knights PT, O’Rourke PK (1988) Effects of weaning and supplementation on performance of Brahman cross cows and their progeny. Australian Journal of Experimental Agriculture 28, 11-20.
| Crossref | Google Scholar |
Hunter RA, Siebert BD (1986) The effects of genotype, age, pregnancy, lactation and rumen characteristics on voluntary intake of roughage diets by cattle. Australian Journal of Agricultural Research 37, 549-560.
| Crossref | Google Scholar |
Iwaniec UT, Turner RT (2016) Influence of body weight on bone mass, architecture, and turnover. Journal of Endocrinology 230, R115-R130.
| Crossref | Google Scholar | PubMed |
Kerridge PC, Gilbert MA, Coates DB (1990) Phosphorus and beef production in northern Australia. 8. The status and management of soil phosphorus in relation to beef production. Tropical Grasslands 24, 221-230.
| Google Scholar |
Knowlton KF, Herbein JH (2002) Phosphorus partitioning during early lactation in dairy cows fed diets varying in phosphorus content. Journal of Dairy Science 85, 1227-1236.
| Crossref | Google Scholar | PubMed |
Little DA (1972) Bone biopsy in cattle and sheep for studies of phosphorus status. Australian Veterinary Journal 48, 668-670.
| Crossref | Google Scholar | PubMed |
McLean RW, Hendricksen RE, Coates DB, Winter WH (1990) Phosphorus and beef production in northern Australia. 6. Dietary attributes and their relation to growth. Tropical Grasslands 24, 197-208.
| Google Scholar |
Naito Y, Shindo N, Sato R, Murakami D (1990) Plasma osteocalcin in preparturient and postparturient cows: correlation with plasma 1,25-dihydroxyvitamin D, calcium, and inorganic phosphorus. Journal of Dairy Science 73, 3481-3484.
| Crossref | Google Scholar | PubMed |
O’Rourke PK, Entwistle KW, Arman C, Esdale CR, Burns BM (1991) Fetal development and gestational changes in Bos taurus and Bos indicus genotypes in the tropics. Theriogenology 36, 839-853.
| Crossref | Google Scholar | PubMed |
Penzhorn EJ, Meintjies JP (1972) Influence of pregnancy and lactation on the voluntary feed intake of Afrikaner heifers and cows. Agroanimalia 4, 83-92.
| Google Scholar |
Puggaard L, Lund P, Liesegang A, Schested J (2014) Long term effect of reduced dietary phosphorus on feed intake and milk yield in dry and lactating dairy cows. Livestock Science 159, 18-28.
| Crossref | Google Scholar |
Read MVP, Engels EAN, Smith WA (1986a) Phosphorus and the grazing ruminant. 2. The effects of supplementary P on cattle at Glen and Armoedsvlakte. South African Journal of Animal Science 16, 7-12.
| Google Scholar |
Read MVP, Engels EAN, Smith WA (1986b) Phosphorus and the grazing ruminant. 3. Rib bone samples as an indicator of the P status of cattle. South African Journal of Animal Science 16, 13-17.
| Google Scholar |
Read MVP, Engels EAN, Smith WA (1986c) Phosphorus and the grazing ruminant. 4. Blood and faecal grab samples as indicators of the P status of cattle. South African Journal of Animal Science 16, 18-22.
| Google Scholar |
Rodehutscord M, Pauen A, Windhausen P, Brintrup R, Pfeffer E (1994) Effects of drastic changes in P intake on P concentrations in blood and rumen fluid of lactating ruminants. Journal of Veterinary Medicine, Series A 41, 611-619.
| Crossref | Google Scholar |
Schatz TJ, McCosker KD, Heeb C (2023) Phosphorus supplementation improves the growth and reproductive performance of female Brahman cattle grazing phosphorus deficient pastures in the Victoria River District, Northern Territory, Australia. Animal Production Science 63, 544-559.
| Crossref | Google Scholar |
Schild CO, Boabaid FM, Olivera LGS, Armendano JI, Saravia A, Custodio A, Algorta J, Alvarez C, Jaurena M, Dixon RM, Riet-Correa F (2023) Response of cows with osteomalacia grazing sub-tropical native pastures to phosphorus supplementation with loose mineral mix or feed blocks. The Veterinary Journal 298–299, 106013.
| Crossref | Google Scholar | PubMed |
Schneider CA, Rasband WS, Eliceiri KW (2012) NIH Image to ImageJ: 25 years of image analysis. Nature Methods 9, 671-675.
| Crossref | Google Scholar |
Seeman E (2013) Age- and menopause-related bone loss compromise cortical and trabecular microstructure. Journal of Gerontology: Series A. Biological Sciences and Medical Sciences 68, 1218-1225.
| Crossref | Google Scholar |
Shupe JL, Butcher JE, Call JW, Olson AE, Blake JT (1988) Clinical signs and bone changes associated with phosphorus deficiency in beef cattle. American Journal of Veterinary Research 49, 1629-1636.
| Google Scholar | PubMed |
Siemon NJ, Moodie EW (1973) Body weight as a criterion in judging bone mineral adequacy. Nature 243, 541-543.
| Crossref | Google Scholar | PubMed |
Silva TACC, Quigley SP, Kidd LJ, Anderson ST, McLennan SR, Poppi DP (2021) Effect of a high crude protein content diet during energy restriction and re-alimentation on animal performance, skeletal growth and metabolism of bone tissue in two genotypes of cattle. PLosONE 16(2), e0247718.
| Crossref | Google Scholar |
Ternouth JH, Coates DB (1997) Phosphorus homoeostasis in grazing breeder cattle. Journal of Agricultural Science 128, 331-337.
| Crossref | Google Scholar |
Ternouth JH, Sevilla CC (1990) The effects of low levels of dietary phosphorus upon the dry matter intake and metabolism of lambs. Australian Journal of Agricultural Research 41, 175-184.
| Crossref | Google Scholar |
Ternouth JH, Bortolussi G, Coates DB, Hendricksen RE, McLean RW (1996) The phosphorus requirements of growing cattle consuming forage diets. Journal of Agricultural Science 126, 503-510.
| Crossref | Google Scholar |
Tuen AA, Wadsworth JC, Murray M (1984) Absorption of calcium and phosphorus by growing cattle during dietary protein deficiency. Proceedings of the Nutrition Society of Australia 9, 144-147.
| Google Scholar |
Valk H, Sebek LBJ (1999) Influence of long-term feeding of limited amounts of phosphorus on dry matter intake, milk production, and body weight of dairy cows. Journal of Dairy Science 82, 2157-2163.
| Crossref | Google Scholar | PubMed |
Wadsworth JC, McLean RW, Coates DB, Winter WH (1990) Phosphorus and beef production in northern Australia. 5. Animal phosphorus status and diagnosis. Tropical Grasslands 24, 185-196.
| Google Scholar |
Wang X-L, Gallagher CH, McClure TJ, Reeve VE, Canfield PJ (1985) Bovine post-parturient haemoglobinuria: effect of inorganic phosphate on red cell metabolism. Research in Veterinary Science 39, 333-339.
| Crossref | Google Scholar | PubMed |
Winks L (1990) Phosphorus and beef production in northern Australia. 2. Responses to phosphorus by ruminants: a review. Tropical Grasslands 24, 140-158.
| Google Scholar |
Winter WH (1988) Supplementation of steers grazing Stylosanthes hamata pastures at Katherine, Northern Territory. Australian Journal of Experimental Agriculture 28, 669-682.
| Crossref | Google Scholar |
Winter WH, Coates DB, Hendricksen RE, Kerridge PC, McLean RW, Miller PC (1990) Phosphorus and beef production in northern Australia. 4. The response of cattle to fertiliser and supplementary phosphorus. Tropical Grasslands 24, 170-184.
| Google Scholar |
Winter EM, Ireland A, Butterfield NC, Haffner-Luntzer M, Horcajada M-N, Veldhuis-Vlug AG, Oei L, Colaianni G, Bonnet N (2020) Pregnancy and lactation, a challenge for the skeleton. Endocrine Connections 9, R143-R147.
| Crossref | Google Scholar | PubMed |