Environmental impacts of Australian pork in 2020 and 2022 determined using lifecycle assessments
M. A. Copley

A
B
Abstract
The Australian pork industry is highly efficient, with a history of ongoing productivity and environmental improvement. The introduction of economy-wide environmental targets require delivering and tracking performance improvement.
This study determined carbon footprint (greenhouse gas [GHG] and land use [LU] and direct land use change [dLUC] emissions, reported as kg CO2-e), fossil energy (MJ), freshwater consumption (L), water stress (L H2O-e), land occupation (m2) and eutrophication potential (nitrogen and phosphorus) for Australian pork for 2020 and 2022. Variability between housing, manure management systems, and regions were identified, and systems analysed to determine new options for low-impact pork.
In the largest Australian study of its kind, data for ~70% of pigs produced were collected using a stratified design. Using attributional life cycle assessment, impacts were reported per kilogram of liveweight (LW), post-processed, retail, and boneless, fat-corrected pork. Results are presented as industry averages ± 2 × s.d.
Key results were 3.0 ± 0.1 and 3.0 ± 0.1 kg CO2-e GHG, 0.4 ± 0.07 and 0.3 ± 0.03 kg CO2-e LU and dLUC, 12.9 ± 0.5 and 13.4 ± 0.5 MJ, 93.8 ± 9.6 and 52.5 ± 3.6 L, 68.4 ± 6.7 and 43.2 ± 3.3 L H2O-e, and 12.0 ± 0.9 and 12.7 ± 0.9 m2/kg LW in 2020 and 2022, respectively. Due to industry growth, total emissions were higher in 2022. Eutrophication potential for Australian pork (2.2 × 10−4 ± 3.0 × 10−5 kg phosphorus and 8.7 × 10−3 ± 3.5 × 10−4 kg nitrogen/kg LW), reported for the first time, was low compared with grazing systems and European piggeries.
Industry has demonstrated long-term performance improvement, though the rate slowed between 2020 and 2022. Ongoing interventions are required to return to trend. Covered pond, deep litter, and outdoor systems produce lower carbon footprint pork and can provide other environmental benefits from renewable energy, and reduced fossil energy demand.
There is potential to further reduce environmental impacts through practice change. If industry is to meet formal targets, investment and proactive policy settings are required to overcome barriers to adoption of existing technology and support the techno-economic case for novel strategies.
Keywords: agricultural systems, carbon footprint, energy, eutrophication potential, greenhouse gases, land use change, life cycle assessment, pigs, pork, water stress.
Introduction
The Australian pork industry has experienced substantial growth over the past four decades, resulting in a national herd of approximately 2.25 million pigs in 2019–2020 (ABS 2021a). The pork industry makes a significant contribution to the Australian economy, valued at $5.3 billion in 2020–2021 (Australian Pork Limited 2023). Production occurs in each state in Australia, with the eastern seaboard (Queensland, New South Wales, and Victoria) responsible for two-thirds of the national herd.
Pork is typically a lower environmental impact protein (de Vries and de Boer 2010; Wiedemann 2018) but life cycle assessments (LCAs) in Australia have focused most strongly on greenhouse gas (GHG) emissions, energy and water (Wiedemann et al. 2016, 2017, in review) and not on other relevant impacts such as nutrient losses. Environmental impacts arise within the farm boundary and from upstream feed production. Most feed production occurs outside the operational boundary of the piggery, where there is less control over reducing impacts. It is important for industry to understand both the direct impacts (within operational control) and indirect impacts (outside operational control) when developing environmental improvement plans.
Baseline environmental impacts (GHG emissions, fossil energy use, freshwater consumption, stress-weighted water use, and land occupation) for Australian pork production were determined previously for 2010–2011 (Wiedemann et al. 2016, 2017). The study covered 14 farms in four major production regions, finding that impacts were strongly influenced by housing and manure management system (MMS) and feed systems. The study concluded that, particularly in conventional systems, improvement in feed conversion ratios (FCR) should result in lower impacts over time (Wiedemann et al. 2016). Compared with international production, baseline environmental impacts of Australian pork tended to be higher than northern hemisphere production for carbon footprint and land occupation (e.g. Dorca-Preda et al. 2021; Zira et al. 2021) but lower for fossil energy use (e.g. Noya et al. 2017; Bonesmo and Enger 2021).
In 2022, the Australian Government legislated an economy-wide 43% emission reduction target (from a 2005 baseline) by 2030 and a target for net zero emissions by 2050. To align with the 2030 target, the Australian pork industry requires an approximate 41% reduction in GHG emissions from its 2010 baseline. Also in 2022, the Australian Government joined the Global Methane Pledge, committing to a minimum 30% reduction in methane emissions across all sectors of the economy by 2030 from a 2020 baseline (Climate and Clean Air Coalition Secretariat 2022). The commitment has implications for pork production and its methane emissions from enteric fermentation, manure handling, and uncovered effluent ponds at both farms and processing plants.
Under the Carbon Credits (Carbon Farming Initiative – Animal Effluent Management) Methodology Determination (Commonwealth of Australia 2019) (Cth), methane destruction and avoidance at piggeries, such as covered anaerobic ponds (CAPs) and solids removal can be registered with the Clean Energy Regulator (CER) and earn Australian carbon credit units (ACCUs). The ACCUs can then be held, sold to the private market, or sold to the Australian Government. The implications around ACCU sales, when this abatement leaves one sector for another (for example, if ACCUs were generated by pig farms but sold to the mining sector to meet government requirements), have not been explored previously. In the context of publicly communicated targets and commitments, to avoid double counting abatement, these reductions need to be removed from the pig industry account when being traded out of the sector, but not where they are sold to the Federal Government (Sevenster et al. 2023).
In addition to GHG, other priority indicators for Australian agri-food production include fossil energy use, land occupation, and freshwater consumption, scarcity and stress (Harris and Narayanaswamy 2009). In addition, nutrient losses are an area of concern for pig production but, although commonly assessed in overseas studies (Noya et al. 2017; Bonesmo and Enger 2021; Dorca-Preda et al. 2021; Zira et al. 2021), eutrophication potential has not previously been assessed for pork using LCA in Australia. Although the pork industry is responsible for impacts across all these indicators, it is also exposed to impacts arising outside its operational control in other industries, most notably via feed grains. It is anticipated that, as industry continues to improve performance, the relative contribution to environmental performance of external sources, such as feed grain production, will increase over time unless substantial intervention is undertaken to achieve equivalent environmental improvement in other industries.
The shift both in Australia and globally to public commitments to long-term environmental improvement targets necessitates ongoing monitoring and reanalysis or interpretation of past results to understand trends and countertrends. In response to this, recent studies of pork production have explored and reported on trends over time (see Bonesmo and Enger 2021; Dai et al. 2021; Dorca-Preda et al. 2021). This study provides two updated benchmarks (financial year (FY)20 and FY22) for the environmental performance of the Australian pork industry and discusses the short- and long-term performance of the industry drawing on past studies of Australian production (Wiedemann et al. 2016, 2017, Wiedemann et al. in review). The updated analysis is fundamental to reporting improvement trends, sustaining improvement by identifying priority areas for industry to address, and identifying and managing emerging countertrends.
Materials and methods
Goal and scope
The study was an attributional life cycle assessment (aLCA) of pork production, including conventional (C), deep litter (DL) and outdoor (O) housing systems. All major production regions, housing and manure management systems (MMS) were assessed using primary industry data, representing 72 and 70% of pigs produced in Australia in the analysis years of FY20 and FY22, respectively.
This study aimed to (1) determine carbon footprint, fossil energy use, freshwater consumption (water footprint), water stress, and land occupation for Australian pork production, and by housing/MMS, (2) determine baseline eutrophication potential (EP) for Australian pork production, and by housing/MMS (3) compare national herd impacts over a 2-year period, (4) compare short-term performance to long-term industry trends (see Wiedemann et al. in review), and (5) identify implications and priorities for the industry into the future.
The study assessed GHG emissions using the Intergovernmental Panel on Climate Change Fifth Assessment Report (IPCC AR5) global warming potentials (GWP100) of 28 kg CO2-equivalent (CO2-e)/kg CH4 and 265 kg CO2-e/kg N2O as applied in the National Greenhouse Accounts Factors (Commonwealth of Australia 2023a). GHG emissions associated with land use (LU) and direct land use change (dLUC) were included and reported separately, as recommended in ISO 14067 (2018).
Demand for fossil fuel energy was assessed by aggregating all fossil fuel energy inputs throughout the system and reporting these per megajoule (MJ) of energy, using lower heating values. Land occupation, reported in square metres (m2), was assessed by aggregating impacts throughout the supply chain.
Freshwater consumption (L) was assessed using methods consistent with ISO 14046 (2018), stress-weighted water use was assessed using the Water Stress Index (WSI) (Pfister et al. 2009) and water scarcity using the available water remaining (AWARE) method (Boulay et al. 2019).
Modelling was undertaken to determine freshwater EP, reported per kilogram of phosphorous (P), and marine EP, reported per kilogram of nitrogen (N), using characterisation factors from ReCiPe (Goedkoop et al. 2009). Results were reported for direct EP (on-farm from pig production) and indirect EP (predominantly from upstream feed grain production).
For direct EP, N and P excretion rates were determined using mass balance principles, from feed inputs, liveweight gain, mortalities, and mean nutrient retention data from Skerman et al. (2016). Nutrient application to land through effluent irrigation and outdoor systems was modelled using MEDLI (Department of Science, Information Technology and Innovation QG 2015) for 11 production areas around Australia. Effluent irrigation was modelled in accordance with practices required by the National Environmental Guidelines for Indoor Piggeries (Tucker 2018) and outdoor production in line with practices required by the National Environmental Guidelines for Rotational Outdoor Piggeries (Tucker et al. 2013).
These results were used to determine typical mass of N and P in groundwater leaching and surface water runoff by region. Impacts from sludge, spent litter, and separated solids to land were not included, as they were outside the system boundary and were treated as residuals used in other enterprises (see Life cycle inventory).
Indirect EP associated with feed production was determined from background grain databases, e.g. AusLCI (ALCAS 2017), ecoinvent (Ecoinvent 2020) and grains processes developed and published in previous LCA studies by the authors.
Water stress, water scarcity, and EP were averaged over both years because they were influenced by changes in reported grain source regions that were likely attributable to changes in the producers surveyed rather than an industry-wide shift in sourcing.
All modelling was carried out using SimaPro™ 9.3 (Pré-Consultants 2021).
Inventory data for three housing systems were collected: C (housing with partially or fully slatted floors where manure, urine, waste feed and water fall into channels or pits that are flushed or released regularly into a pond treatment system), DL (pigs are housed on litter, e.g. straw, sawdust, rice hulls) and O, where pigs are allowed to range in an open paddock and are supplied with shelters.
The primary production supply chain included farrow-to-finish operations (breeding to grow-out) and meat processing, with all associated inputs. Data were collected for 36 and 42 farrow-to-finish operations to cover a 12-month production period from 1 July 2019 to 30 June 2020 and from 1 July 2021 to 30 June 2022, respectively. Data were deidentified and aggregated to ensure company data remained confidential.
The endpoint of the supply chain was the retail shelf (see Supplementary Fig. S1). Results were presented at three points in the supply chain with the following reference units: 1 kg of liveweight at the abattoir gate, 1 kg of pork ready for distribution to retail (a mix of primary and further processed product), and 1 kg of pork at the retail shelf. The distribution and retail functional units reflect the product mix of bone-in and boneless portions. In addition, results were reported per kilogram of boneless pork at the retail shelf.
Stratification
Producer data were aggregated, weighted, and stratified based on housing system, MMS and production region, to develop a national herd dataset for FY20 and FY22. The proportion of each manure management system type for FY20 (Table 1) and FY22 (Table 2) were determined from the collated project survey data, total sow numbers and insights regarding the proportion of sows housed in each MMS from the National Inventory Report (Commonwealth of Australia 2022, 2023b), industry consultation, and expert judgement. Further detail on the stratification is provided in the Supplementary Materials.
Australia | NSW | Qld | Vic. | WA | SA | Tas. | ||
---|---|---|---|---|---|---|---|---|
Outdoor | 7.8% | 6.0% | 3.0% | 7.2% | 22.4% | 2.0% | 2.0% | |
Deep litter | 31.1% | 35.6% | 6.0% | 35.5% | 33.0% | 52.6% | 20.0% | |
Effluent pond (uncovered) | 34.8% | 21.4% | 53.9% | 37.9% | 29.7% | 23.1% | 73.0% | |
Anaerobic digester/CAP | 19.0% | 34.6% | 34.6% | 13.6% | 0.0% | 6.7% | 0.0% | |
Short HRT A | 1.4% | 0.7% | 0.4% | 1.9% | 1.4% | 3.0% | 3.0% | |
Solids separation | 5.9% | 1.7% | 2.1% | 3.9% | 13.5% | 12.7% | 2.0% |
Australia | NSW | Qld | Vic. | WA | SA | Tas. | ||
---|---|---|---|---|---|---|---|---|
Outdoor | 8.2% | 6.0% | 3.0% | 7.2% | 24.4% | 2.0% | 2.0% | |
Deep litter | 30.9% | 35.6% | 6.0% | 35.5% | 32.2% | 52.6% | 20.0% | |
Effluent pond (uncovered) | 35.9% | 21.4% | 53.0% | 44.7% | 26.3% | 23.1% | 73.0% | |
Anaerobic digester/CAP | 17.7% | 34.6% | 35.5% | 6.7% | 2.2% | 6.7% | 0.0% | |
Short HRT A | 1.4% | 0.7% | 0.4% | 1.9% | 1.4% | 3.0% | 3.0% | |
Solids separation | 5.9% | 1.7% | 2.1% | 3.9% | 13.5% | 12.7% | 2.0% |
Life cycle inventory
The study collected data from a representative cross-section of the industry, reflecting 72% and 70% of sows in all major regions (in FY20 and FY22, respectively), for all housing and MMS, and included farrow-to-finish production, feed milling, and primary and further meat processing. Summary data and methods for the development of the benchmarking by housing/MMS are outlined in Table S1 and the Supplementary Materials.
Average feedmilling processes (n20 = 5 and n22 = 5) were developed using inventory data collected as part of the project and existing datasets (Table S2).
Pigs were phase fed, and diets may change during the year according to the availability of commodities. Diets (n20 = 35 and n22 = 43) were reported by each producer. Weighted average commodity inputs for the national herd for FY20 and FY22 are presented in Table 3, where lesser volume commodities are grouped under general headings for conciseness and to protect confidential diet formulations. The weighted average national herd composition was derived from weighted averages of each state, which were developed from the diets reported by each producer. The ranges given in Table 3 reflect the variation in producer diets reported for each year.
Feed commodity (% of total diet) | FY20 | FY22 | |||
---|---|---|---|---|---|
National herd | Range in farm dataset | National herd | Range in farm dataset | ||
Barley grain | 29.5% | 1.2–51.6% | 25.0% | 0.5–57.4% | |
Wheat and triticale grain | 37.3% | 1.7–59.0% | 39.8% | 9.4–53.8% | |
Sorghum grain | 1.5% | 0.0–37.5% | 3.1% | 0.0–35.5% | |
Maize grain | 0.0% | 0.0–3.1% | 0.0% | 0.0–1.4% | |
Pulse grains, e.g. faba beans, lupins | 6.6% | 0.0–24.5% | 8.2% | 0.0–29.8% | |
Plant protein meals (excluding soy) | 7.2% | 0.0–13.0% | 7.0% | 0.0–13.1% | |
Soybean meal | 2.7% | 0.5–10.1% | 2.6% | 0.0–8.9% | |
Animal proteins, e.g. bloodmeal, meat and bone | 3.6% | 0.7–8.3% | 3.8% | 1.0–8.8% | |
Fats and oils, e.g. canola, vegetable, palm | 0.5% | 0.0–5.3% | 0.5% | 0.0–1.8% | |
Tallow | 1.4% | 0.0–2.4% | 1.0% | 0.0–1.5% | |
High-cost additives, e.g. synthetic amino acids, enzymes | 1.6% | 0.4–5.0% | 1.4% | 0.1–3.5% | |
Low-cost additives e.g. salt, lime | 1.8% | 0.3–4.6% | 2.1% | 0.1–9.1% | |
Diverted food waste | 0.9% | 0.0–34.0% | 1.0% | 0.0–44.0% | |
By-products | 5.0% | 0.0–27.6% | 4.4% | 0.0–31.0% | |
Residuals | 0.7% | 0.0–19.9% | 0.5% | 0.0–20.2% | |
Total | 100% | – | 100% | – |
Feed production was modelled as described in Copley et al. (2023) and methods are not repeated in detail here. Briefly, major feed grains were modelled from Australian grain processes from the AusLCI database (ALCAS 2017), where available. Average irrigation rates in each region and the proportion of cropland irrigated were used to determine the proportion of cereal grains produced in dryland and irrigated systems (ABS 2021a, 2021b, 2022a, 2022b), to build markets for FY20 and FY22. The FY22 grain market was based on FY21 data as complete FY22 data were not available at the time of analysis.
LU and dLUC emissions and removals in Australian cropland for the 10 years prior to both the analysis periods were determined. National and state datasets (from the National Greenhouse Accounts (Commonwealth of Australia 2023a)): cropland remaining cropland, excluding woody perennials, and land converted to cropland) were used to determine emissions and removals (see Table S3) for Australia and for each state. As each producer reported source regions for feed grains, state LU and dLUC emissions were weighted according to the proportion of feed grains sourced by the national herd from each state.
Imported soybean meal was modelled to reflect the Australian import market breakdown for FY20 and FY22 (OEC 2020, 2023), using data from the ecoinvent database (Ecoinvent 2020).
Herd performance, including feed intake and the total mass of pigs produced, was determined from records supplied by each producer (n20 = 35 and n22 = 44) and represents actual performance under commercial conditions (Table 4). Records of pig numbers, water use, and energy use and renewable energy generation were collected from farms operating in five states by surveying producers. Data were then deidentified, aggregated and stratified for the national herd (see Stratification).
National herd FY20 | National herd FY22 | |||
---|---|---|---|---|
Progeny FCR – wean to finish | kg feed/kg LW | 2.37 | 2.33 | |
Herd feed conversion (HFC) | kg feed/kg DW | 3.82 | 3.75 | |
Progeny sold/sow.year | No. | 20.49 | 20.94 | |
DW progeny sold/sow.year | kg | 1572 | 1632 |
DW, dressed weight.
A mass balance, based on primary data for feed and pig production, was used to estimate manure excretion. Manure GHG emissions (methane [CH4], nitrous oxide [N2O]) were estimated by predicting nitrogen (N) and volatile solids (VS) excretion using mass balance principles. Subtracting N inputs (in feed) from N outputs in pig mass and mortalities gave excreted N. Excreted VS was determined by subtracting manure ash from excreted total solids (TS), which represented the residual of non-digested feed (Dong et al. 2006). Waste VS included excreted VS and waste feed that was deposited into the MMS. The sensitivity of key assumptions regarding feed was assessed (see Sensitivity Analysis Methodology in Supplementary Materials).
N retention was determined using methods and factors determined using liveweight data from farms and pig composition data. Emissions were determined using state-specific emission factors for each MMS, based on those used in the National Inventory Report (NIR) (Commonwealth of Australia 2022). Indirect N2O was modelled from manure ammonia (NH3) volatilisation, leaching and runoff where relevant. All DL and C sheds were assumed to be constructed with impervious floors and, as effluent and manure capture were modelled according to environmental regulations, nitrate leaching from sheds was therefore assumed to be negligible. For outdoor production, leaching and runoff were assessed for range areas. Allocation of impacts to spent litter, sludge and separated solids is described in Handling co-production.
Inventory data were collected from primary processing (n20 = 7 and n22 = 7) plants (Table S4) operating across five states, and for further processing plants (n20 = 3 and n22 = 5) (Table S5).
Processing inputs were reported per tonne of hot standard carcase weight (HSCW) and chilled carcase weight (CCW), respectively, but results are reported relative to 1 kg of pork product ready for distribution (i.e. the average mix of products from across the industry). This product mix was 48% and 46% direct from primary processing in FY20 and FY22, respectively, and 52% and 54% from further processing in FY20 and FY22. Energy sources differed between processing plants, resulting in an apparently wide range for some energy inputs, e.g. liquid petroleum gas (LPG) and Natural Gas were substitutable heat energy sources meaning that (in Table S4) where processing facilities reported zero LPG, natural gas would be used as a heat source and vice versa.
The retail phase included transport to warehouses, warehouse operations, transport to retail, and retail operations. Average impacts from warehouse and retail operations were derived from published Australian supermarket data for GHG emissions, energy consumption, and waste for FY20 and FY22 (Coles Group 2022a; Woolworths Group 2022a), and an intensity per kilogram of pork at the retail shelf was derived from an economic allocation using published financial data (Woolworths Group 2020, 2022b; Coles Group 2022b). Transport to warehousing and from warehousing to retail shelf was derived from the known location of primary and major secondary pork processing facilities, state and territory population statistics (ABS 2021c, 2023), and assumed population distribution within each state/territory. Summary data used to model environmental impacts per kilogram of pork at the retail shelf are outlined in Table S6.
Handling co-production
The total product mass from the system was the sum of human-edible meat, including edible offal. Other outputs included manure, effluent (including separated solids) and spent litter, pet food and processing by-products for rendering (co-products from feed inputs arose outside the system boundary). Primary production and meat processing co-products and handling methods were described in Wiedemann et al. (2016).
Effluent from conventional piggeries was typically land-applied on site to crops or pastures grazed by beef cattle or sheep. Solid residues such as sludge and spent litter were more readily transported off site for application to crop land. Emissions arising from land application of these solid residues were allocated to the industry that utilised the manure nutrients. Following Wiedemann et al. (2016), 30% of nutrients from manure solids were assumed to return to the grain production system, representing 0.7% of cereal crop fertiliser requirements nationally in FY20 and 0.6% in FY22. Manure solids were included as an input to the modelled cereal crop systems used in the feed inventory.
To determine impacts per kilogram of boneless fat-corrected pork, the reference unit of one kilogram of pork at the retail shelf was adjusted by the edible yield for retail portions of pork (85%), determined previously by Wiedemann and Yan (2014), and the average fat content (20.3%) of Australian retail pork (derived from Greenfield et al. 2009). Results were then reported as 1 kg of boneless, fat-corrected pork at the retail shelf.
Sensitivity analysis
The sensitivity of the model and results to key assumptions and parameters were tested. Methodological details for the sensitivity analyses were outlined in the Supplementary materials. The sensitivity of the model to assumptions regarding feed waste (% of total feed fed) and dietary crude protein (CP) for pigs in O systems were determined. The sensitivity of the results to assumptions regarding grain source regions was also determined.
Accounting for Australian Carbon Credit Units (ACCUs)
Carbon credits provide a means of transferring emission reductions, and carbon removals, between businesses and between businesses and the Federal Government. In accordance with Sevenster et al. (2023), carbon credits from activities within the pig industry (e.g. Australian Carbon Credit Unit (ACCU) Scheme projects for CAPs) that are sold via the private market are to be reported separately in the industry carbon account.
No adjustment was made to the product carbon footprint to add the emissions associated with the sale of ACCUs, i.e. emission intensities were inclusive of all avoided emissions from CAPs, regardless of whether the ACCUs were held, sold to the Federal Government or the private market. This ensured that the product carbon footprint (CF) was representative of actual biological performance and reduced complexity in multi-year results comparisons of product CF, i.e. that the impacts were reported for ‘steady state’ production and could be compared with established pork and other industry benchmarks.
Uncertainty analysis
Two types of uncertainties (alpha and beta) were assessed via Monte Carlo analysis in SimaPro (Pré-Consultants 2021), using one thousand iterations to provide a 95% confidence interval for the results for each year. Results are presented as the mean plus or minus two times the standard deviation (s.d.), i.e. mean ± 2 s.d.
Results
Impacts per kilogram of liveweight
Environmental impacts per kilogram of liveweight for the national herd in FY20 and FY22 are reported in Table S7. Impact sources are presented graphically in Fig. S2 for GHG emissions (incl. LU and dLUC), fossil energy, and freshwater consumption. The product carbon footprints for FY20 and FY22 are reported in Table S8 disaggregated as Scope 1, 2 and 3 sources.
Benchmark GHG emissions (excl. LU and dLUC), fossil energy use, freshwater consumption, and land occupation impacts per kilogram of liveweight for each farm surveyed in 2020 and 2022 are presented in Figs 1, S3, S4, and S5, alongside the averages (of both years) for different housing and MMS. Note that F1.2020 and F1.2022 denotes the result for Farm 1 in 2020 and 2022, i.e. the same farm in both years. Full results for the Australian average production by housing and MMS are reported in Table S9 and are presented graphically in Fig. 2 to demonstrate differing impact sources.
GHG emissions (excl. LU and dLUC) per kilogram of liveweight for surveyed farms for FY20 and FY22, presented alongside national average results for each housing and manure management system, and national average results for FY20 and FY22. Note that the averages in the figure are the simple averages of the results for each housing/MMS for both years.
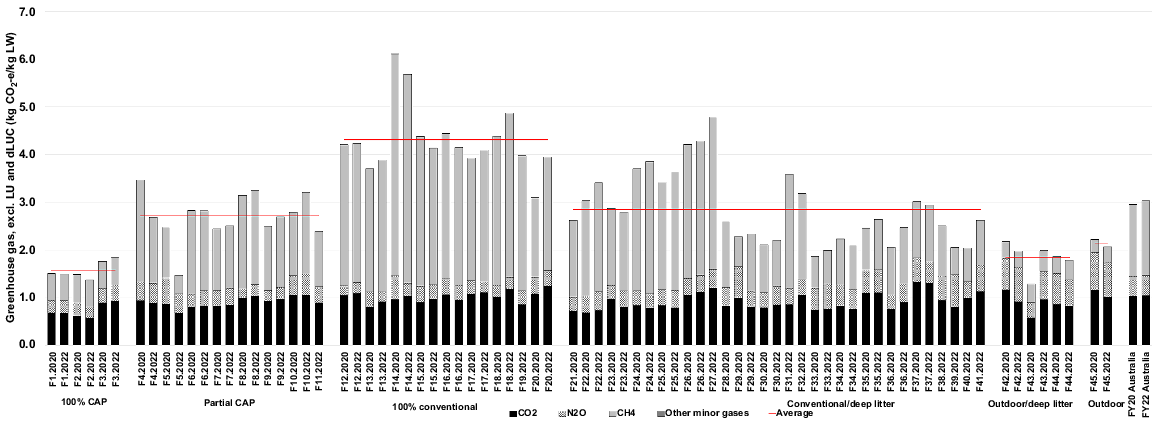
Environmental impacts for the national herd by MMS for FY22. (a) Greenhouse gas emissions (incl. LU and dLUC), (b) fossil energy use, (c) freshwater consumption, and eutrophication potential in (d) freshwater and (e) marine settings.
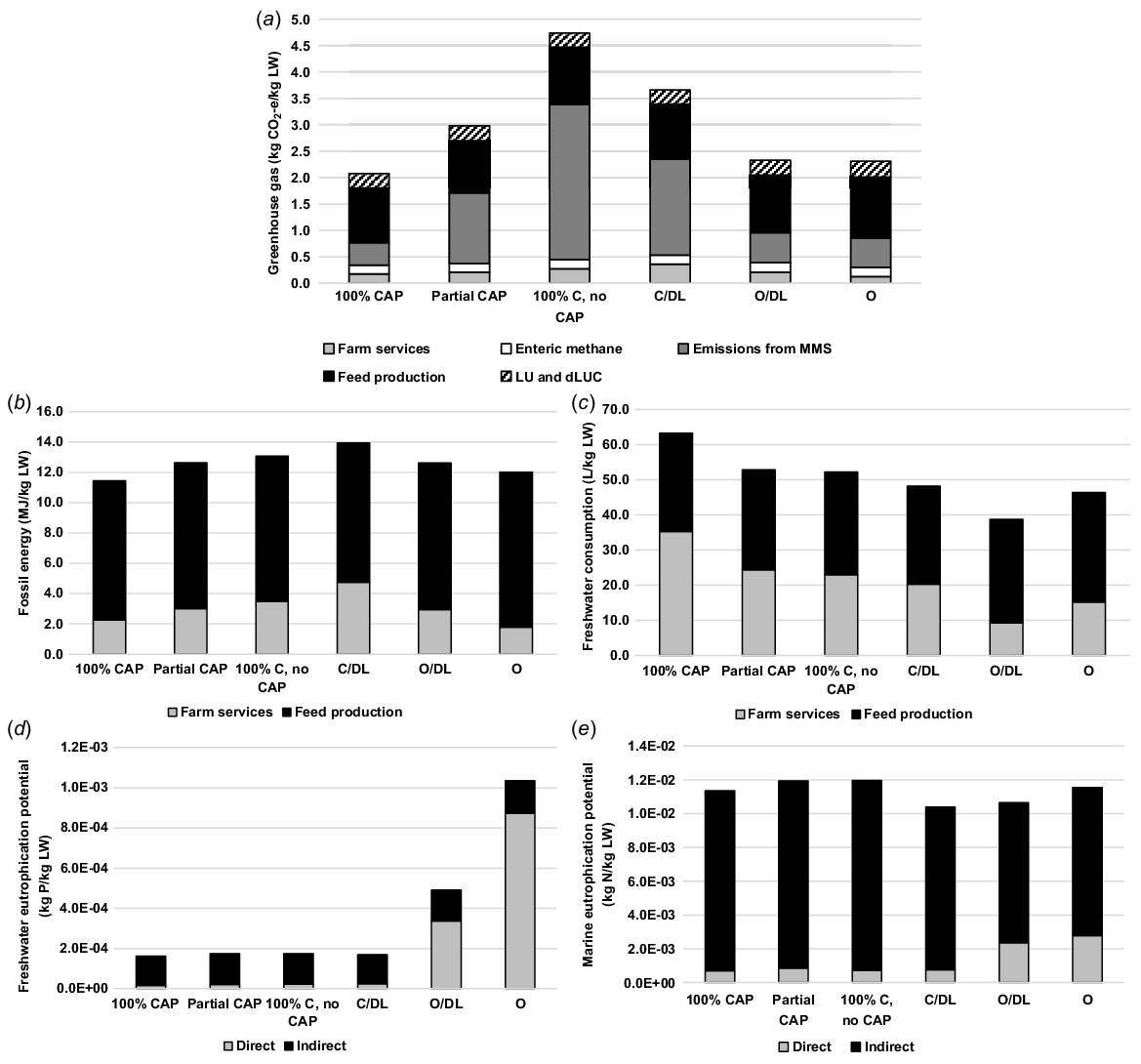
The weighted average results for Australia in 2020 and 2022 respectively were 3.0 ± 0.1 and 3.0 ± 0.1 kg CO2-e/kg GHG emissions, 0.4 ± 0.07 and 0.3 ± 0.03 kg CO2-e/kg LU and dLUC emissions, 12.9 ± 0.5 and 13.4 ± 0.5 MJ/kg energy use, 93.8 ± 9.6 and 52.5 ± 3.6 L/kg LW freshwater consumption and 12.0 ± 0.9 and 12.7 ± 0.9 m2/kg LW land occupation. Water stress, scarcity, and EP were 55.8 ± 5.0 L H2O-e/kg LW and 14.0 ± 3.3 m3/kg LW, 2.2 × 10−4 ± 3.0 × 10−5 kg P/kg LW, 8.7 × 10−3 ± 3.5 × 10−4 kg N/kg LW respectively, presented as an average of 2020 and 2022.
Between years there was no significant difference in GHG emissions or energy use. There was a trend towards a slight decline in GHG emissions from LU and dLUC in 2022 in response to a change in sourcing for imported soybean meal. Interestingly, MMS emissions were slightly higher in FY22 because of a change in the proportion of pigs housed in conventional systems compared to other lower-emitting MMS systems but HFC improved marginally in FY22, resulting in no change overall in GHG emissions. GHG impacts (excl. LU and dLUC) ranged from 1.3 to 6.1 kg CO2-e/kg LW in the farm dataset, with the highest impacts arising in C housing systems with uncovered ponds and relatively poor herd performance.
Three production systems that produced low carbon footprint pork were observed. First, farms with 100% CAP systems, and high fractions of waste and residual feed commodity use, second, O systems, and third, C breeder, DL grow-out farms where more than 80% of manure was generated in the DL system (i.e. sows are housed on litter for a portion of the year). In CAP systems (i.e. where CH4 emissions from uncovered effluent ponds are avoided), CH4 capture facilitates generation of renewable energy (from biogas), and the feeding systems are conducive to large volumes of diverted food waste and residuals in feed. Overall, CAP systems offer circular economy advantages. Product CF was low in O systems and DL systems because manure is deposited directly to land or shed and decomposes in an aerobic environment, leading to lower methane emissions than uncovered effluent ponds. O systems were also found to have relatively low on-farm energy requirements (see Fig. 2).
Total fossil energy use ranged from 6.7 to 16.0 MJ/kg LW. Lowest total fossil energy was associated with 100% CAP systems, which generated the majority of their on-farm requirements, and exported surplus energy, whilst also having lower indirect energy as a result of higher volumes of residuals and waste products in feed.
Fresh water consumption decreased substantially, largely because feed grains in 2020 had a higher proportion of irrigation than in 2022, which was influenced by seasonal rainfall in these seasons and the preceding grain-growing season. Freshwater consumption and water stress ranged from 15.7 to 352.0 L/kg LW and 5.3 to 261.9 L H2O-e/kg LW in the farm dataset. Variation in freshwater consumption was larger between farms than between housing/MMS but removing the irrigation variable was found to show a trend in lower water consumption in DL/O systems, as per Wiedemann et al. (2017). Water consumption and water stress were primarily a function of grain source region, with farms that utilised feed grains from regions with irrigation having significantly higher water footprints.
Similarly, land occupation showed a considerable range in impacts, from 1.8 to 20.8 m2 in the farm dataset (see Fig. S5) and this was principally related to the amount of waste and residual commodities in feed, variation in grain yields in source regions and, to a lesser extent, HFC. Systems that had higher HFC utilised more grain, corresponding to higher land occupation when all other factors remained equal. The lowest land occupation impacts were found in systems with the highest inclusion rates of residuals and diverted food waste (i.e. lower than average cereal grain inclusion rates).
EP was found to range from 6.8 × 10−5 to 2.4 × 10−3 kg P/kg LW and 2.3 × 10−3 to 1.8 × 10−2 kg N/kg LW in the farm dataset. EP was influenced by feed production, but there was also a trend related to MMS, with higher direct impacts for P found in O housing systems compared to C and C/DL, and no substantial differences between housing systems for direct N EP.
Total impacts from primary production
Total impacts for the industry to the farm gate in FY22 and FY22 were 1.81 and 1.99 Mt CO2-e respectively for GHG emissions including all stages of the life cycle and LU and dLUC emissions, and having accounted for ACCUs sold from the industry to private sector (see Tables S10 and S11). Total emissions, disaggregated by Scope 1, 2 and 3 sources, are reported in Table S12. Fossil energy use was 6839 and 7758 GJ and freshwater consumption was 49,678 and 30,281 ML in FY20 and FY22, respectively.
Impacts per kilogram of post-processed pork ready for distribution to retail
Environmental impacts per kilogram of post-processed pork (i.e. the market mix of pork ready for distribution to retail) are reported for Australia for FY20 and FY22 in Table S13. Except where there were substantial changes in impacts in the primary production phase (LU and dLUC emissions, freshwater consumption and stress), there were no major changes in environmental impacts between the 2 years as there was little change in primary and further processing between FY20 and FY22. Key results per kilogram were 5.3 ± 0.2 and 5.4 ± 0.2 kg CO2-e, 0.7 ± 0.1 and 0.4 ± 0.1 kg CO2-e, 26.1 ± 0.8 and 26.5 ± 0.9 MJ, and 160.4 ± 20.7 and 74.9 ± 6.3 L for GHG, LU and dLUC, fossil energy and freshwater consumption in FY20 and FY22, respectively.
Impacts per kilogram of retail pork
Environmental impacts per kilogram of pork at the retail shelf (retail pork, RP) are reported for Australia for FY20 and FY22 in Table 5 and graphically for GHG emissions, fossil energy use and freshwater consumption in Fig. 3.
Units | Australia FY20 | 2.s.d. (±) | Australia FY22 | 2.s.d. (±) | ||
---|---|---|---|---|---|---|
GHG emissions | kg CO2-e | 6.4 | 0.2 | 6.5 | 0.2 | |
GHG emissions – LU and dLUC | kg CO2-e | 0.7 | 0.1 | 0.4 | 0.1 | |
Total GHG emissions | kg CO2-e | 7.1 | 0.4 | 6.8 | 0.3 | |
Fossil energy use | MJ | 37.6 | 0.8 | 37.8 | 1.1 | |
Renewable energy generated and consumed | MJ | 0.2 | 0.0 | 0.2 | 0.0 | |
Freshwater consumption | L | 164.4 | 43.6 | 94.3 | 28.2 | |
Water stress | L H2O-e | 120.4 | 31.9 | 75.0 | 22.5 | |
Land occupation | m2 | 19.0 | 5.0 | 20.3 | 6.1 | |
Total freshwater eutrophication potential | kg P | 3.5 × 10−4 | 1.3 × 10−4 | 4.0 × 10−4 | 4.5 × 10−5 | |
Total marine eutrophication potential | kg N | 1.2 × 10−2 | 4.4× 10−4 | 1.6× 10−2 | 6.5× 10−4 |
Impacts per kilogram of boneless, fat-corrected pork
Impacts per kilogram of bone-adjusted (boneless) and fat-corrected pork portions at the retail shelf are shown in Table 6. Results were 48% higher than the market mix of pork at the retail shelf due to the loss of mass associated with bones and fat.
Units | National herd FY20 | 2 s.d. (±) | National herd FY22 | 2 s.d. (±) | ||
---|---|---|---|---|---|---|
GHG emissions | kg CO2-e | 9.4 | 0.3 | 9.5 | 0.3 | |
GHG emissions – LU and dLUC | kg CO2-e | 1.0 | 0.2 | 0.6 | 0.1 | |
Total GHG emissions | kg CO2-e | 10.4 | 0.5 | 10.1 | 0.4 | |
Fossil energy use | MJ | 55.5 | 1.2 | 55.8 | 1.7 | |
Renewable energy generated and consumed | MJ | 0.3 | 0.0 | 0.3 | 0.0 | |
Freshwater consumption | L | 242.6 | 64.3 | 136.6 | 41.6 | |
Water stress | L H2O-e | 177.7 | 47.1 | 110.7 | 33.1 | |
Land occupation | m2 | 28.0 | 7.4 | 29.9 | 9.0 | |
Total freshwater eutrophication potential | kg P | 5.2 × 10−4 | 1.9 × 10−4 | 5.9 × 10−4 | 6.6 × 10−5 | |
Total marine eutrophication potential | kg N | 1.8 × 10−2 | 6.5 × 10−4 | 2.4 × 10−2 | 9.6 × 10−4 |
Discussion
Major impacts and trends in farm production
GHG emission profiles were generally consistent with the national herd for 2010, reported by Wiedemann et al. (2016), and international LCAs (see Arrieta and González 2019; Pexas et al. 2020; Zira et al. 2021). Longer term trends in Australia were examined through comparison with the Wiedemann et al. (in review) dataset, which was re-analysed using AR5 Global Warming Potentials (GWPs). These findings are described below.
The benchmark product carbon footprint (excl. LU and dLUC) for 2022 was 74% lower than 1980. Across the full time series, the industry has achieved an annualised rate of improvement of 1.8%, but no improvement was observed between 2020 and 2022.
When analysed at greater depth, the GHG results revealed an increase in emissions from direct (Scope 1) sources at the farm level (see Table S8). Particularly notable was an increase in MMS related emissions between 2020 and 2022, which was driven by a slightly higher proportion of the national turnoff from conventional piggeries, and no further expansion in CAPs or other low-emission housing systems. The average proportion of manure treated in CAPs in FY20 was 70% and ranged from 20% to 100%. In FY22 the average was 68% and ranged from 19% to 100%, decreasing slightly because one CAP was decommissioned, and two others built on farms where 20–30% of manure was treated in the CAP. Considering the increased societal focus on GHG during this time period, it is clear that the rate of practice change is controlled and limited by other factors, which have stalled uptake. Although not the focus of this analysis, anecdotally, barriers to adoption include the high cost of implementation and the diminishing number of highly suitable sites, as the majority of the optimum sites have already taken up CAP technology.
It was also notable that a sizeable proportion of the uptake in CAPs has occurred in supply chains where only a proportion of total manure was treated by CAP. Multi-site, geographically separated piggeries have a general mismatch between the location of the high energy yielding manure and high volumes of manure (grower-finisher sites) and the lower energy yielding, lower manure volume and higher energy demand breeder sites. Anecdotally, this has been a factor limiting complete (i.e. 100%) uptake of CAPs in farrow-finish systems. This trend implies interventions are required to drive uptake, and a review of strategies to achieve this appears warranted. Interestingly, the analysis also showed that some sites were achieving comparably low GHG emissions through very high utilisation of litter-based systems. Farm 33, for example, with majority (>85%) DL production and on-farm solar generation, achieved a product CF similar to 100% CAP production. For this to be achieved, a proportion of sows also need to be housed on straw, together with the grower and finisher pigs. This housing design has had much less attention than CAPs and may be an alternative, lower cost option for producing low GHG emission pork in southern Australia.
A range of other factors also affected inter-annual variability in production, which occurred even within similar housing and MMS types and on the same farms between years. External factors, such as disease, for example, resulted in poorer productivity across several farms (e.g. Farm 8, Farm 9, Farm 17) in 2022, contributing to higher product CFs in that year.
Indirect factors were also influential on the farm-gate emissions, and the emission trend. The majority of LU and dLUC impacts per kilogram of liveweight were attributable to two sources: imported soybean meal from South America (Arrieta et al. 2018) and Australian crop production. Between FY20 and FY22, LU and dLUC impacts per kilogram of LW fell by 36% (0.43–0.28 kg CO2-e). This substantial decrease was driven by a change in the import market for soybean meal between FY20 and FY22, from 98.3% South American to 82.6% (OEC 2020, 2023), which resulted in considerably lower LU and dLUC emissions per kilogram of soybean meal. Alternative markets which have grown as a proportion of total imports have comparatively negligible LU and dLUC impacts, e.g. LU and dLUC emissions of 0.0001 kg CO2-e/kg soy from the United States compared with 3.27 kg CO2-e/kg for South America (Ecoinvent 2020).
In accordance with updated LCA guidance, LU and dLUC emissions from Australian cropland were also included in the analysis using a rolling 10-year baseline period of assessment. Disaggregating LU and dLUC impacts from Australian cropland in the analysis period revealed substantial variations between Australian states. The national average over 10 years showed cropland was a source of LU and dLUC emissions, whereas some states had removals (see Table S3). In Western Australia (WA), for example, LU and dLUC emissions from cropland were –0.33 Mt CO2-e in 2020 (where negative emissions indicate removals), compared with 1.74 Mt CO2-e (emissions) in New South Wales (NSW) in the same year (Table S3).
This finding presents a challenge for pork. Grain is a major input to production, and although there are significant hopes that soil will be a source of emission removal in the agricultural sector, this conflicts with recent 10-year trends from the National Greenhouse Accounts for cropping soils in most states. Urgent work is needed to quantify soil carbon under cropland at a more granular level within LCA to match guideline requirements and confirm regional performance. A range of initiatives are underway to reverse the trend in soil carbon decline in cropping which, if achieved, will benefit grain users such as pork.
Between 1980 and 2022 fossil energy use declined by 61%. Interestingly, total energy per kilogram of liveweight was 4% higher in FY22 than FY20, driven by changes in grain source regions. Most impacts in energy use were associated with feed production upstream from the farm, which contributed three-quarters of the energy per kilogram of liveweight (Fig. S2).
For individual farms, fossil energy use also exhibited considerable variation (Fig. S3), which was partly masked by the substantial amount of indirect energy associated with feed. For farms surveyed in both 2020 and 2022, average fossil energy use was found to increase by 4% over the 2 years. Aside from poor productivity influencing total fossil energy (e.g. in Farm 20 in FY22, Farm 18 in FY22, and O production), some farms’ total fossil energy footprint was comparatively high due to on-farm diesel feedmilling (e.g. Farm 41). External factors (i.e. disease) resulted in elevated energy intensity for some farms (e.g. Farm 8) between 2020 and 2022.
Average fossil energy consumption was lowest in 100% CAP and O systems; however, on-farm consumption was lowest in the latter (see Fig. 2). Average 100% CAP production and partial CAP production systems generated and used the greatest proportion of renewable energy (Table S9). Several farms reported generation of renewable energy on-farm either through biogas or solar systems. In the case of some farms, renewable energy generation (and sale) was substantial enough that the farm was a net exporter of energy. As pig farms have a combination of available land and residual energy in manure, they have the potential to be small scale, rural energy generators, which may provide a unique solution in the national energy mix. It was noted that at industry level, on-site renewable energy generation remained a relatively small part of the energy mix, suggesting there is significant room for expansion (see Table S7).
Freshwater consumption in 2022 was 90% lower than the reported result for 1980, and 44% lower in 2022 than in 2020. Comparing 2020 and 2022, irrigation in feed grain production decreased from 60 L per kilogram of liveweight, to 30 L in FY22 (see Fig. S2). Shortening the analysis interval from 10 years to 2 years demonstrated the sensitivity of the analysis to these shorter-term seasonal impacts. In some cases, as for Farm 2, Farm 14, and Farm 26, considerable declines in impacts were observed. Although the longer-term trend of declining water use is expected to continue (largely due to declining availability of irrigation water for cereal grains), this will be subject to more volatility when measured at closer intervals and this should be taken into account when interpreting trends. At farm scale, further work is needed to provide traceable grain, and specifically to note the presence or absence of irrigation water, in order to generate accurate, farm scale water footprints.
Housing/MMS also influenced results, with average water consumption in O production being slightly higher than in O/DL (Fig. 2) because of productivity impacts and likely higher water use for wallows. Conventional systems generally had higher on-farm water consumption because of shed cleaning with freshwater (Fig. 2).
Water stress results have declined over time (see Wiedemann et al. in review) however, in the present study comparisons between years were confounded by a lack of regionally characterised, temporally specific water stress data. For this reason, comparisons were not made, and future analyses will require much more specific and regularly updated grains and water stress datasets.
Between 1980 and 2022, land occupation impacts for the industry declined by 42%. Land occupation impacts were driven by upstream feed production; more than 99% of land occupation impacts per kilogram of liveweight were associated with feed. Between FY20 and FY22, land occupation impacts increased slightly (in contrast with freshwater consumption) as the proportion of feed grains from irrigated production declined as production returned to trend, reducing the proportion of feed grains grown in high-yield irrigated systems. As with water, closer analysis intervals will result in greater fluctuation in land occupation, associated with seasonal variation in grain yield.
Freshwater EP and marine EP were reported here for the first time for the pig industry. Results in Fig. 2 are disaggregated between direct (piggery) and indirect (cropping etc.) sources for the national herd, broken down by housing/MMS. EP was generally dominated by the indirect contribution of upstream feed production, primarily assumed losses of synthetic fertilisers used in cropping.
Direct freshwater EP arose from direct application of manure to soil in O production, and effluent irrigation in C production. These loss rates must be carefully contextualised. Firstly, the study outcomes were modelled, not measured. Confirmation of EP would require measurement of loss rates from the boundary of farms. Secondly, loss rates were relatively low compared with international studies (Noya et al. 2017; Zira et al. 2021) (see Table S16), partly in response to strict environmental controls in Australia and more significantly, because of the lower rates of water flow compared with regions such as Europe.
Presenting results on an intensity basis allowed for interesting comparisons to be drawn with alternative production systems. In the present study, extensive (O) production was found to have much higher EP, because all manure was deposited on relatively small areas of land that were then rotated with cropping over time. C systems provided the capability to centrally manage nutrients (particularly P), allowing it to be controlled, then spread over large areas of cropland infrequently, when sludge is removed from pig ponds. Extending this comparison, based on the nutrient loss data of Drewry et al. (2006), P losses from sheep meat production in Vic. may be similar to or significantly higher than intensive pork production (see Comparison with other proteins), depending on stocking density. This demonstrates that assessing total nutrient load, as is typically done in regulatory contexts, could be complemented by assessing nutrient intensities, which reveal piggeries are efficient producers of meat relative to environmental impacts.
Pig farms’ contribution to EP is further complicated by the modelling of point-source and dispersed-source nutrient loads. Nutrient loads are transported from point source and diffuse sources in different ways, however, LCA EP models do not take these different sources into account when determining impacts. Point sources often deliver a constant or frequent discharge of nutrients from a particular location, whereas most diffuse sources are transported to waterways during episodic storm events over a wide area of the waterway (Davis and Koop 2006). In the Australian context, EP in several river catchments is strongly associated with point-source emissions and low flow conditions (Kerr 1994; Eyre et al. 1997). Nutrients from diffuse sources that occur during episodic storm events over a wide area of the waterway are usually sufficiently diluted and dispersed to minimise EP (Eyre et al. 1997). The disregard of nutrient sources could significantly affect the eutrophication potential, limiting the accuracy of knowledge derived from these studies. Piggeries will typically contribute to diffuse nutrient loss sources because nutrients are dispersed across crop or pastureland following effluent irrigation, solids separation or direct deposition. EP models are therefore likely to overestimate impacts.
We note also that different methods rely on different fate and transport models which, when paired together with varying user assumptions and generalised characterisation factors to represent different conditions, can lead to vastly different results, making meaningful comparison between systems difficult (Morelli et al. 2018).
As a newly applied method in the context of Australian pork production, there were several limitations with the EP model. EP impacts, particularly direct EP, should be interpreted with caution as the background model (ReCiPe) relies on European theory of EP risks. As marine environments in Europe are usually N-limited, whereas freshwater environments are P-limited, ReCiPe distinguishes between P-limited freshwater impacts and N-limited marine eutrophication impacts. Australian environments, however, can be N-limited, P-limited or co-limited depending on catchment (McCaskill et al. 2003). Davis and Koop (2006) found that N plays an equally significant role with P in controlling the biomass of freshwater algal blooms in south-east and south-west Australian rivers. Thus, models that use a single limiting nutrient to determine EP only characterise part of the risk in the Australian context.
For all housing and manure management systems except for O, direct marine EP was very low. In O production, direct N EP occurred as a result of direct deposition to soil. In other systems, direct N EP was associated with effluent irrigation and spent litter handling.
EP was reported here for the first time, but limitations exist in interpretation of the results, particularly for N where a high proportion of total impacts were driven by ammonia-N loss. Interestingly, EP was relatively low compared with international literature and compared with limited analysis of extensive Australian livestock production, indicating that reporting impacts on an intensity basis provides a useful comparative view and may improve the general understanding of environmental performance of pig farms.
Whole of supply chain impacts
All impacts were higher at the retail shelf than at other stages of the supply chain for multiple reasons. Mass losses that occurred in the product flow between stages uniformly increased impacts across all categories. Additional GHG emissions and energy use were attributable to fossil energy inputs in transport, at distribution centres and retail operations. Retail operations and logistics accounted for 13% of GHG emissions (incl. LU and dLUC) per kilogram of retail pork in FY22 (see Fig. 3). Of this, grid electricity consumption at retail operations was the major source of emissions. There were no significant differences between the two analysis periods for impacts at primary and further processing.
Per kilogram of pork at the retail shelf, energy inputs at processing and post processing accounted for 15% of the total, and fossil energy at the retail level accounted for 27% of the total (Fig. 3). This demonstrates that although primary production may dominate the emission profile, distribution and retail networks are considerable contributors to energy consumption.
Between 2020 and 2022, freshwater consumption and stress also declined per kilogram of pork ready for distribution to retail (Table S13) and per kilogram of retail pork (Table 5), however, this was overwhelmingly attributable to upstream feed production rather than reduced water consumption at processing or retail. In 2022, water consumption at retail represented only 1% of the total freshwater consumption per kilogram of pork at the retail shelf whereas consumption at primary and further processing accounted for 9%. Any additional direct EP at primary processing was attributable to irrigation of treated wastewater on-site, which was a minor contribution.
Total emissions
Although the carbon account (total industry emissions incl. LU and dLUC) in 2022 was 56% lower than in 1980 (see Wiedemann et al. in review), industry expansion to increase food production resulted in higher total GHG emissions in FY22 compared to the previous analysis period (see Table S10). Net GHG emissions for the industry (i.e. total emissions, including LU and dLUC, plus ACCUs generated and sold on the private market) also increased between FY20 and FY22 by 10%, a combination of a 6% increase in total emissions from expanded production and the sale of more than 70,000 carbon credits to private enterprises outside the Australian pork industries (see Table S11). Where ACCU Scheme project holders sell to the Federal Government, as the credits are not retired against a claim, it is not double counting to account for these reductions in the pork industry. This market dimension is a new consideration for agricultural industries including pork, where the apparent environmental benefits from interventions may not be attributable to the industry because these are sold to other sectors. In FY22, ACCU sales outside the industry accounted for 6% of the sectoral (Scope 1 and 2) net emissions reported in Table S12.
Sectoral emissions (not adjusted for ACCU sales) represented 54% and 53% of the industry’s net carbon account (reported in Table S11) in FY20 and FY22. Scope 1 emissions from MMS were the greatest contributor to the sectoral carbon account, accounting for 78% and 75% of the emissions in FY20 and FY22, respectively. This finding demonstrates that the potential for the greatest emission reduction lies in the transition of to lower emission intensity housing systems (for those producers not already operating CAPs, with high proportions (>85%) of deep litter, or outdoor production).
The contribution of the major gases to total industry emissions (excl. LU and dLUC) did not change between FY20 and FY22 (Table S11). For net emissions, however, the contribution of CH4 increased in both percentage and absolute terms between the 2 years in response to a decrease in CO2-e emissions from LU and dLUC.
Comparison with other proteins
The present study analysed impacts to the retail shelf, which was an extended supply chain compared with most protein LCAs reported to date. To aid comparability, we compared results excluding the retail and distribution stages for pork, i.e. per kilogram of boneless, fat-corrected pork ready for distribution to retail (see Table S17).
Environmental impacts were higher than retail-ready, boneless chicken meat (Copley and Wiedemann 2022), and shell- and protein-corrected eggs (Copley et al. 2023) for GHG, LU and dLUC, fossil energy use, freshwater consumption, water stress, and land occupation. Compared with protein- and fat-corrected lamb (see Wiedemann 2018), boneless, retail-ready pork had lower impacts for GHG excl. LU and dLUC, freshwater consumption and stress, and total land occupation but higher impacts for fossil energy use and arable land occupation. Boneless, fat-corrected beef (see Wiedemann 2018), had higher GHG emissions (excl. LU and dLUC), freshwater consumption and stress, and total land occupation impacts per kilogram than pork, but lower fossil energy use and arable land occupation impacts.
None of the comparison Australian studies for poultry, beef, or lamb reported EP. Piggeries may lose a small proportion of nutrients from the site in runoff losses even under best management practices, but comparatively, total meat production is very high. Sheep production in southern Australia, for example, may have stocking rates from 4 to 25 dry sheep equivalent (DSE) per hectare, yielding between 80 and 500 kg LW/ha.year and 20–120 kg wool/ha.year. Australian research has indicated that levels of P loss at the paddock scale is in the range from 0.01 to 0.56 kg P/ha.year, with higher values associated with heavily fertilised, southern grazing regions where rainfall is winter dominant (McCaskill et al. 2003) and both runoff and drainage represent a considerable fraction of annual rainfall (Stevens et al. 1999; Cox and Ashley 2000). Using an average P loss of 0.2 kg P/ha.year and N losses of 2.5 kg N/ha.year (Drewry et al. 2006) and converting to an emission per kilogram of product, impacts from sheep may range from 0.0002 to 0.001 kg P/ha.year, which was considerably higher than the results presented for pork here, suggesting that intensive production may have lower impacts as a meat production system when managed to regulatory requirements.
Implications for industry
This study has several implications for the Australian pork industry and other industries more broadly. First, the sale of carbon credits generated by one industry and then sold to the private market represents an impediment to the former achieving absolute emission reductions. It is possible that the number of carbon credits sold outside the industry may decline in future years, i.e. this is a short-term anomaly, as several producers’ ACCU Scheme projects came to the end of their 7-year life. From a carbon accounting perspective, the expiration of these projects is advantageous as the industry may henceforth retain the full benefit of CAPs. However, from an investment perspective, ability to sell credits to the private market and generate an alternative revenue stream may be fundamental to producers’ capacity to invest in the required infrastructure. Without appropriate alternative incentives, it will be difficult to cease the sale of credits outside the industry as the financial incentive is substantial and this underpins investment.
The results also show that passive improvements resulting from changes in the supply chain can occur and result in lower impacts over time. The change in import location for soymeal was an example, as was the decline in irrigation water use for cropping. Notably, these trends can also reverse without the industry having control. One option in the future is for industries to take a much more proactive stance in requiring higher environmental performance from suppliers, to drive better performance. It is unclear if this will result in higher costs but will be necessary if long-term reduction trends are to be continued.
We noted several instances where the move to short-term reporting will result in higher inter-annual variability, e.g. the fluctuations in freshwater consumption and land occupation. At farm scale, year-on-year performance improvement is also not certain, and individuals and industry should be cautious when setting public short-term improvement targets until a clear trend, with consistent drivers, has been established. Emerging regulation, however, will over time see major stakeholders in the Australian pork industry annually publicly report GHG emissions and eventually progress against emission reduction targets.
This study revealed no reduction in product CF from direct sources in the most recent time period, though with the impact of LU and dLUC included, this did result in a slight decline overall. This indicated industry will need to focus strongly on enabling technology, cost reduction and a broader suite of options for reducing GHG emissions. It is also likely industry will require transition support from government to achieve further large emission reductions. Although there are established and proven technologies and strategies that facilitate environmental improvement (e.g. covering effluent ponds, use of diverted food waste (only in compliance with Restricted Animal Material requirements) as a dietary input, renewable energy), the cost-effective uptake has begun to plateau, necessitating further research and investment to overcome barriers to adoption and determine the techno-economic feasibility of other strategies, e.g. reduced dietary CP in O production, optimal spent litter handling on farm loss, supplying piggery nutrients to grain sector to displace synthetic fertiliser, and grain traceability to optimise sourcing from dryland production systems without soil carbon losses.
A further implication from this study was the comparison of impacts to international imports (i.e. from Europe (see Bonesmo and Enger 2021; Dorca-Preda et al. 2021; Zira et al. 2021) and the United States (see Tallaksen et al. 2020)). Australian pork generally had a higher product CF (compared with 3.6 kg/CO2-e kg pork at retail gate in Sweden (Zira et al. 2021), 2.6 kg/CO2-e kg meat at slaughterhouse gate in Denmark (Dorca-Preda et al. 2021), however, nutrient losses in European systems were much higher. This suggests the practice of importing from Europe results in greater nutrient problems in these nations, which is an acute challenge, and that this problem may be alleviated through expanding local production, though at the cost of slightly higher GHG emissions, even when transport from Europe is included.
Limitations
As temporally specific water stress factors were not available in the grains inventory or for piggeries, comparison of water scarcity between 2020 and 2022 should not be made as the results described alongside Table S7 were based on changes in volume only and did not reflect inter-annual variation in scarcity. This was limited by the lack of temporally specific analyses of water stress in background processes.
The lack of Australian-specific characterisation factors for EP is a significant knowledge gap. Further research is required, including to benchmark field edge nutrient loss in Australian piggery systems by region, soil type and rainfall zone. This research could improve the knowledge base around nutrient losses by also reporting impact on an intensity basis. In the absence of this specific research, caution should be exercised when interpreting the reported EP.
Conclusions
As the environmental credentials of agri-food products become more important to customers and consumers, the ability of the Australian pork industry to quantify, understand and communicate performance and long-term trends is increasingly important for the industry’s social licence. This study was the most comprehensive of its kind in Australia based on industry coverage, which represented 70–72% by volume. Between 1980 and 2022, the industry has reduced product carbon footprint (incl. LU and dLUC) by 78%, fossil energy use by 61%, freshwater consumption by 90%, and land occupation by 42%, though the rate of change was found to have slowed in the most recent period for GHG emissions, suggesting interventions and concerted effort will be required to continue emission reduction. Scope 1 emissions from housing and manure management systems were the greatest contributor to the sectoral carbon account (Scope 1 and 2 emissions), accounting for 78% and 75% of the emissions in FY20 and FY22, respectively. This finding demonstrates that the potential for the greatest emission reduction lies in the ongoing transition to lower emission intensity housing systems. Piggeries with CAPs, high proportions (>85%) of deep litter, or outdoor housing produce pork with lower GHG emissions, providing a range of options for the industry. Advancing progress towards low-emissions pork would be enhanced by a structured emission reduction pathway for industry, developed with broad input from stakeholders extending from suppliers to retail, and from government and finance. Further, pork is a relatively low impact meat production system with significant potential to further reduce environmental impacts by implementing sustainable practice change.
Data availability
The disaggregated inventory data that support this study cannot be publicly shared because of confidentiality requirements for supply of commercially sensitive data.
Declaration of funding
This research was funded by Australian Pork Limited (APL Project No. 2021/0014). Funding for APL is provided primarily through statutory pig slaughter levies with additional research-specific funds provided by the Australian Government.
Acknowledgements
The authors gratefully acknowledge the support of Australian Pork Limited. The participating companies, growers and facility managers are thanked for supplying data. Sara Willis (Queensland Department of Agriculture and Fisheries) is thanked for assisting with data collection.
References
ABS (2021a) 7121.0 – agricultural commodities, Australia, 2019–20. Australian Bureau of Statistics, Australia. Available at https://www.abs.gov.au/statistics/industry/agriculture/agricultural-commodities-australia/2019-20
ABS (2021b) Water use on Australian farms, 2019–2020 – 4618.0. Australian Bureau of Statistics, Canberra, Australia. Available at https://www.abs.gov.au/statistics/industry/agriculture/water-use-australian-farms/
ABS (2021c) National, state and territory population, December 2020. Available at https://www.abs.gov.au/statistics/people/population/national-state-and-territory-population/dec-2020
ABS (2022a) Agricultural commodities, Australia – 2020–2021. Available at https://www.abs.gov.au/statistics/industry/agriculture/agricultural-commodities-australia/2020-21
ABS (2022b) Water use on Australian farms, 2020–2021. Available at https://www.abs.gov.au/statistics/industry/agriculture/water-use-australian-farms/latest-release
ABS (2023) National, state and territory population, December 2022. Available at https://www.abs.gov.au/statistics/people/population/national-state-and-territory-population/dec-2022
ALCAS (2017) AusLCI. Australian Life Cycle Assessment Society, Australia. Available at%20http://auslci.com.au/
Arrieta EM, González AD (2019) Energy and carbon footprints of chicken and pork from intensive production systems in Argentina. Science of The Total Environment 673, 20-28.
| Crossref | Google Scholar | PubMed |
Arrieta EM, Cuchietti A, Cabrol D, Gonzalez AD (2018) Greenhouse gas emissions and energy efficiencies for soybeans and maize cultivated in different agronomic zones: a case study of Argentina. Science of The Total Environment 625, 199-208.
| Crossref | Google Scholar | PubMed |
Australian Pork Limited (2023) Industry facts. Available at https://www.australianpork.com.au/industry-facts
Bonesmo H, Enger EG (2021) The effects of progress in genetics and management on intensities of greenhouse gas emissions from Norwegian pork production. Livestock Science 254, 104746.
| Crossref | Google Scholar |
Boulay A-M, Benini L, Sala S (2019) Marginal and non-marginal approaches in characterization: how context and scale affect the selection of an adequate characterization model. The AWARE model example. The International Journal of Life Cycle Assessment 25, 2380-2392.
| Crossref | Google Scholar |
Climate and Clean Air Coalition Secretariat (2022) Global methane pledge. Available at https://www.globalmethanepledge.org/
Commonwealth of Australia (2022) National inventory report 2020. Vol. 1. (Commonwealth of Australia). Available at https://www.dcceew.gov.au/climate-change/publications/national-inventory-report-2020
Commonwealth of Australia (2023b) National inventory report 2021. Vol. 1. (Commonwealth of Australia). Available at https://www.dcceew.gov.au/climate-change/publications/national-inventory-report-2021
Copley MA, Wiedemann SG (2022) Environmental impacts of the Australian poultry industry. 1. Chicken meat production. Animal Production Science 63, 489-504.
| Crossref | Google Scholar |
Copley MA, Wiedemann SG, McGahan EJ (2023) Environmental impacts of the Australian poultry industry: 2. Egg production. Animal Production Science 63, 505-521.
| Crossref | Google Scholar |
Cox JW, Ashley R (2000) Water quality of gully drainage from texture-contrast soils in the Adelaide Hills in low rainfall years. Soil Research 38, 959-972.
| Crossref | Google Scholar |
Dai X, Sun Z, Müller D (2021) Driving factors of direct greenhouse gas emissions from China’s pig industry from 1976 to 2016. Journal of Integrative Agriculture 20, 319-329.
| Crossref | Google Scholar |
Davis J, Koop K (2006) Eutrophication in Australian Rivers, Reservoirs and Estuaries - A Southern hemisphere perspective on the science and its implications. Hydrobiologia 559, 23-76.
| Crossref | Google Scholar |
de Vries M, de Boer IJM (2010) Comparing environmental impacts for livestock products: a review of life cycle assessments. Livestock Science 128, 1-11.
| Crossref | Google Scholar |
Department of Science, Information Technology and Innovation QG (2015) Model for Effluent Disposal Using Land Irrigation (MEDLI). Available at https://science.des.qld.gov.au/government/science-division/water-and-coastal/medli
Dong H, Mangino J, McAllister T, Hatfield J, Johnson D, Lassey K, Aparecida de Lima M, Romanovskaya A, Bartram D, Gibb D, Martin J (2006) Emissions from livestock and manure management. In ‘IPCC guidelines for national greenhouse gas inventories. Vol. 4: Agriculture, Forestry and Other Land Use’. (Eds S Eggleston, L Buendia, K Miwa, T Ngara, K Tanabe) pp. 10.1–10.87. (Institute for Global Environmental Strategies: Kanagawa, Japan)
Dorca-Preda T, Mogensen L, Kristensen T, Knudsen MT (2021) Environmental impact of Danish pork at slaughterhouse gate – a life cycle assessment following biological and technological changes over a 10-year period. Livestock Science 251, 104622.
| Crossref | Google Scholar |
Drewry JJ, Newham LTH, Greene RSB, Jakeman AJ, Croke BFW (2006) A review of nitrogen and phosphorus export to waterways: context for catchment modelling. Marine and Freshwater Research 57, 757-774.
| Crossref | Google Scholar |
Ecoinvent (2020) Ecoinvent 3.6 database. ecoinvent Centre. Available at https://www.ecoinvent.org/database/ecoinvent-36/ecoinvent-36.html
Goedkoop M, Heijungs R, Huijbregts M, De Schryver A, Struijs J, Van Zelm R (2009) ReCiPe 2008. A life cycle impact assessment method which comprises harmonised category indicators at the midpoint and the endpoint level. First edition, Report I: Characterisation. Ministrie van Volkshuisvesting, Ruimtelijke Ordening en Milieubeheer.
Greenfield H, Arcot J, Barnes JA, Cunningham J, Adorno P, Stobaus T, Tume RK, Beilken SL, Muller WJ (2009) Nutrient composition of Australian retail pork cuts 2005/2006. Food Chemistry 117, 721-730.
| Crossref | Google Scholar |
Harris S, Narayanaswamy V (2009) A literature review of life cycle assessment in agriculture. Rural Industries Research and Development Corporation. Barton, Australia. Available at https://agrifutures.com.au/product/a-literature-review-of-life-cycle
McCaskill MR, Ridley AM, Okom A, White RE, Andrew MH, Michalk DL, Melland A, Johnston WH, Murphy SR (2003) SGS nutrient theme: environmental assessment of nutrient application to extensive pastures in the high rainfall zone of southern Australia. Australian Journal of Experimental Agriculture 43, 927-944.
| Crossref | Google Scholar |
Morelli B, Hawkins TR, Niblick B, Henderson AD, Golden HE, Compton JE, Cooter EJ, Bare JC (2018) Critical review of eutrophication models for life cycle assessment. Environmental Science & Technology 52, 9562-9578.
| Crossref | Google Scholar | PubMed |
Noya I, Villanueva-Rey P, González-García S, Fernandez MD, Rodriguez MR, Moreira MT (2017) Life cycle assessment of pig production: a case study in Galicia. Journal of Cleaner Production 142, 4327-4338.
| Crossref | Google Scholar |
OEC (2020) Where does Australia import soybean meal from? Observatory of Economic Complexity. Available at https://oec.world/en/resources/about
OEC (2023) Where does Australia import soybean meal from? (2021). Available at https://oec.world/en/visualize/tree_map/hs92/import/aus/show/42304/2021/
Pexas G, Mackenzie SG, Wallace M, Kyriazakis I (2020) Environmental impacts of housing conditions and manure management in European pig production systems through a life cycle perspective: a case study in Denmark. Journal of Cleaner Production 253, 120005.
| Crossref | Google Scholar |
Pfister S, Koehler A, Hellweg S (2009) Assessing the environmental impacts of freshwater consumption in LCA. Environmental Science & Technology 43, 4098-4104.
| Crossref | Google Scholar | PubMed |
Skerman AG, Willis S, McGahan EJ, Borgognone MG, Batstone DJ (2016) Validation of PigBal model predictions for pig manure production. Animal Production Science 56, 1081-1090.
| Crossref | Google Scholar |
Stevens DP, Cox JW, Chittleborough DJ (1999) Pathways of phosphorus, nitrogen, and carbon movement over and through texturally differentiated soils, South Australia. Australian Journal of Soil Research 37, 679-693.
| Google Scholar |
Tallaksen J, Johnston L, Sharpe K, Reese M, Buchanan E (2020) Reducing life cycle fossil energy and greenhouse gas emissions for Midwest swine production systems. Journal of Cleaner Production 246, 118998.
| Crossref | Google Scholar |
Tucker R, O’Keefe M, St AU, Ian AL, Rk PO, Ited IM (2013) National environmental guidelines for rotational outdoor piggeries. (Australian Pork Limited: Barton, ACT). Available at http://australianpork.com.au/wp-content/uploads/2016/07/NGforOP_2013_22_lowres.pdf
Wiedemann S (2018) Analysis of resource use and greenhouse gas emissions from four Australian meat production systems, with investigation of mitigation opportunities and trade-offs. Doctoral thesis, Charles Sturt University, Bathurst, New South Wales. Available at https://researchoutput.csu.edu.au/ws/portalfiles/portal/75667383/Stephen_Wiedemann_Thesis.pdf
Wiedemann SG, McGahan EJ, Murphy CM (2016) Environmental impacts and resource use from Australian pork production assessed using life-cycle assessment. 1. Greenhouse gas emissions. Animal Production Science 56, 1418-1431.
| Crossref | Google Scholar |
Wiedemann SG, McGahan EJ, Murphy CM (2017) Environmental impacts and resource use from australian pork production determined using life cycle assessment. 2. Energy, water and land occupation. Animal Production Science 58, 1153-1163.
| Crossref | Google Scholar |
Wiedemann S, Watson K, Biggs L, McGahan E, Copley M-F (in review) Trends in the environmental impacts of the Australian pork industry. Animal Production Science. doi:10.1071/AN23361
Zira S, Rydhmer L, Ivarsson E, Hoffmann R, Röös E (2021) A life cycle sustainability assessment of organic and conventional pork supply chains in Sweden. Sustainable Production and Consumption 28, 21-38.
| Crossref | Google Scholar |